DOI:
10.1039/D1RA00987G
(Paper)
RSC Adv., 2021,
11, 13034-13039
Bio-CaRGOS: capture and release gels for optimized storage of hemoglobin†
Received
5th February 2021
, Accepted 12th March 2021
First published on 7th April 2021
Abstract
Room temperature biospecimen storage for prolonged periods is essential to eliminate energy consumption by ultra-low freezing or refrigeration-based storage techniques. State of the art practices that sufficiently minimize the direct or hidden costs associated with cold-chain logistics include ambient temperature storage of biospecimens (i.e., DNA, RNA, proteins, lipids) in the dry state. However, the biospecimens are still well-exposed to the stress associated with drying and reconstitution cycles, which augments the pre-analytical degradation of biospecimens prior to their downstream processing. An aqueous storage solution that can eliminate these stresses which are correlated to several cycles of drying/rehydration or freezing of biospecimens, is yet to be achieved by any current technology. In our study, we have addressed this room temperature biospecimen-protection challenge using aqueous capture and release gels for optimized storage (Bio-CaRGOS) of biospecimens. Herein, we have demonstrated a single-step ∼95% recovery of a metalloprotein hemoglobin at room temperature using a cost-effective standard microwave-based aqueous formulation of Bio-CaRGOS. Although hemoglobin samples are currently stored at sub-zero or under refrigeration (4 °C) conditions to avoid loss of integrity and an unpredictable diagnosis during their downstream assays, our results have displayed an unprecedented room temperature integrity preservation of hemoglobin. Bio-CaRGOS formulations efficiently preserve hemoglobin in its native state, with single-step protein recovery of ∼95% at ambient conditions (1 month) and ∼96% (7 months) under refrigeration conditions. In contrast, two-thirds of the control samples degrade under ambient (1 month) and refrigeration (7 months) settings.
1. Introduction
Diagnosis of several ailments requires the extraction of whole blood from patients and further requires transportation and storage under refrigeration conditions (4 °C). This is specifically done to preserve the integrity of model proteins and specific markers whose structure and concentration are used to diagnose an ailment. These refrigerated conditions, as well as lyophilization, is also frequently used to ship immunotherapy and other protein-based treatments to hospitals for treatment. Considering how integrated refrigerated shipping has become within transport protocols, it is necessary to explore cheaper and more efficient alternative methods of preserving the integrity of proteins and other target compounds. In doing so, the cost of patient diagnosis and treatment may be substantially reduced.
The importance of hybrid organic–inorganic gels derived from a silica precursor (e.g. tetraethoxysilane) for encapsulation of biomolecules has been well recognized. Typically, a silane molecule is hydrolyzed using catalysts such as acids or bases and solvated using co-solvents such as alcohol, which results in the formation of a sol. The resulting sol can be added to buffers containing biomolecules and results in immobilization. The process is nearly bio-compatible, allows for good control of pH and ionic strength and is applicable to variety of biomolecules.1,2 Specifically, nanostructured porous silica matrices are well-known for their excellent payload capacity for proteins/peptides,3–7 nucleic acids (DNA, RNA),8,9 nucleic acid aptamers10 and microRNA specimens.11 Despite the success over the last three decades, the inherently complex nature of conventional sol–gel methods makes them hardly applicable to clinical setting. Second, most sol–gel techniques are marred with a critical issue. i.e. the release of biospecimen from gels or glasses after long-term encapsulation. The primary reason for the low recovery of biospecimen is due to the use of high concentrations of silica precursor (typically 30–50%), as the techniques always strived to obtain intact immobilization in a glass like matrix, to restrict the motion of biomolecule. In the past, we have developed several sol gel routes and demonstrated their efficacy in biomolecule storage.12–15 Specifically, we had developed a novel CVD based sol gel route, where a solution containing any biomolecule of choice is simply exposed to vapors of silica precursor tetramethyl orthosilicate (TMOS) at room temperature for 1 to 12 h in a simple Petri dish at room temperature. During this process, TMOS evaporates and hydrolyzes at the interface of aqueous solution, then diffuses into the aqueous solution and results in encapsulation of the biomolecule. This process was extremely successful and was proven to work for a variety of proteins, chlorosomes, microbial cells, and liposomes.16 Although we circumvented the issues of utilization of alcohols and acids, several challenges still remain including precise control of silica hydrolysis and condensation reactions, lack of release of biomolecule upon immobilization, incompatibility with clinical practices, as well as contamination issues. However, a key observation was realized during the CVD process, where results indicate that a low-concentration (<10% silica) can preserve the biomolecule, and second, if the concentration can be further decreased, then the release of the biomolecule is possible. Therefore, this triggered research in the direction of a process that is compatible with clinical settings, requires minimal technical expertise, utilizes low concentration of silica precursor and can be performed around the globe in any environment.
In this article, we have engineered capture and release gels for optimized biospecimen storage (CaRGOS) by hydrolysis of TMOS using standard microwave. The key attributes of CaRGOS are (i) a clinically compatible ∼1 minute hydrolysis process using a standard bench top microwave (ii) minimal presence of methanol (byproduct) that is not deleterious to biospecimen (iii) effective long-term storage of biospecimen and (iv) ease of biospecimen recovery through CaRGOS matrix using the biocompatible molecule PEG. We have specifically addressed the room-temperature stability and mechanical handling of hemoglobin in nearly aqueous CaRGOS formulations (less than 5%) that preserve metalloprotein's nativity, homogeneity, activity and reproducibility over long-term storage. Using CaRGOS of 5% TMOS, greater than 95% of hemoglobin retained native structure at room temperature for a period of 33 days at room temperature and up to 7 months in refrigerator at 4 °C. Control groups (w/o CaRGOS) degraded significantly under similar conditions. The PEG release protocol allowed for 91% of the Hb preserved in 1% gels to be extracted via centrifuge. Such strong stability of the hemoglobin content within CaRGOS formulations is strongly correlated to the scientific premise of isoelectric pH (pI) of proteins.17 Protein's solubility, stability, activity and net charge [(+)ve or (−)ve] is heavily determined by the pI of the proteins.18 Hemoglobin, for instance has pI of 6.8 and therefore incur (−)ve charge at pH 8.2 (used in study), therefore it stays soluble and is stable within our (−)vely charged silica formulations (pH 8.2). The hemoglobin stability in our CaRGOS formulations is therefore attributed to two factors. Firstly, the electrostatic repulsions between (−)vely charged hemoglobin and silica is possibly driving the protein stability within these colloidal dispersions. Secondly, the immobilization or restricted rotation of biospecimens imparted by silica formulations is stabilizing the hemoglobin content. Immobilization is the result of either entrapping or collaterally depositing themselves alongside the native conformation of biospecimen. This immobilization is unique due to their conformation or shape recognizing capabilities, such that a congruent coupling of silica nanostructures occurs alongside the biospecimens.3 Therefore, the CaRGOS formulation technique in this article is remarkably valid for preservation of most proteins and various biomarkers including nucleotides.
2. Experimental
2.1 Materials
Tetramethyl orthosilicate (TMOS, > 98% purity), freeze-dried hemoglobin, polyethylene glycol (2 kDa), sodium phosphate monobasic, sodium phosphate dibasic, 15.0 mL centrifuge tubes and UV-vis cuvettes were purchased from Sigma Aldrich (St. Louis, MO). UV-vis absorption spectra were collected using a UV-vis spectrometer (Varian Cary 50 BIO UV, Agilent Technologies, Santa Clara, CA). Raman spectra were acquired on Reva Educational Raman platform (Hellma, Plainview, NY).
2.2 CaRGOS synthesis
A 10.0 v/v % TMOS stock-solution was prepared in de-ionized water and transferred to a 40.0 mL glass test tube, screw capped and hydrolyzed via microwave for thirty seconds. Post-microwave, the screwcap was removed to evaporate the volatile byproduct (i.e., methanol) of the CaRGOS synthesis. This CaRGOS stock solution was allowed to cool to room temperature. After room temperature was reached, appropriate amounts of CaRGOS were added to 4.0 mL cuvettes to create final concentrations (v/v %) of 0, 1, 2.5, 5 and 7.5 respectively. Phosphate buffer (0.5 M pH 8.2) was added to constitute the remainder of the 3 mL solution, as well as 0.03 mL of 1.0 w/v% hemoglobin.
2.3 Storage of hemoglobin in CaRGOS
Several samples of CaRGOS–hemoglobin [(0.0–7.5) v/v % TMOS; 0.01 w/v % hemoglobin; 0.5 M phosphate buffer, pH 8.2; 3.0 mL] solutions were formulated in 4.0 mL UV-vis cuvettes, capped and stored for a desired amount of time. The UV-vis spectra of stored CaRGOS–hemoglobin [(0.0–7.5) v/v % TMOS; 0.01 w/v % hemoglobin; 0.5 M PB, pH 8.2; 3.0 mL] solutions were measured on 0, 2, 6, 9, 13, 18, 20, 24, 27, 31, 33 days at room-temperature, to validate integrity of hemoglobin in the CaRGOS. For long-term studies, we used optimized CaRGOS concentration [i.e., 5.0 v/v % TMOS], while keeping rest of formulation parameter fixed [0.01 w/v % hemoglobin; 0.5 M PB, pH 8.2; 3.0 mL] and were measured over a period of 210 days, to validate integrity of hemoglobin over the prolonged room-temperature and refrigerated storage conditions.
2.4 Release of hemoglobin from CaRGOS
Polyethylene glycol (65.0 μM, 1.0 mL) was added to 3 mL CaRGOS containing hemoglobin for facile re-dissolution of the silica-dispersions. After vortexing the sample for 30 seconds, 1.0 mL of the resulting solution was pipetted to a 15.0 mL centrifuge tube. This process was completed until a total of 5.0 mL PEG had been added to each sample, after which the remainder of the dissolved CaRGOS was pipetted into the 15.0 mL centrifuge tube. 3.0 mL of the dissolved CaRGOS was transferred to two 1.5 mL centrifuge tubes for each sample, after which they were centrifuged for 13 minutes at 10
000 rpm. The supernatant hemoglobin solution at the top of each tube was pipetted into the corresponding UV-vis cuvette, where UV-vis spectroscopy was used to determine the concentration and structure of native hemoglobin.
2.5 Evaluation of CaRGOS evolution using Raman spectroscopy
The Raman spectra was performed on CaRGOS [(0.0–10.0) v/v % TMOS], CaRGOS with buffer [(0.0–10.0) v/v % TMOS; 0.5 M PB, pH 8.2; 3.0 mL] and CaRGOS with hemoglobin/buffer [(0.0–7.5) v/v % TMOS; 0.01 w/v % hemoglobin; 0.5 M PB, pH 8.2; 3.0 mL] using a Reva Educational Raman platform (Hellma, Plainview, NY, USA). The laser power of 450.0 mW and current 959.0 mA was optimized to analyze the samples. The laser temperatures [diode = 30 °C; case = 24.4 °C] and spectrometer temperature [23.1 °C] were optimized for collecting the Raman spectra.
2.6 Evaluation of gel formation using IR spectroscopy
FT-IR spectra were measured with an FT-IR spectrometer (PerkinElmer Spectrum 100) with universal attenuated total reflectance (ATR) sample accessory. The CaRGOS samples with different concentration were placed on diamond/ZnSe crystal and the spectra were recorded over the wavenumber range 4000–500 cm−1.
3. Results and discussions
3.1 Long-term UV-vis analysis of hemoglobin content within the CaRGOS formulations
Hemoglobin binds and transport analytes (i.e., oxygen, nitric oxide, carbon monoxide) and plays significant role in the regulation of the blood pressure. Hemoglobin is a model protein of our choice for investigating the preservation of structural integrity under environmental stimuli (heat, mechanical excursions, nuclease/protease/microbial contamination), due to its complex four protein-chain frameworks, with each chain having heme group and metal center (i.e., iron) in the central cavity. Purified proteins in their native state are known to be slightly disordered and for having certain sections in their unfolded state.19 Therefore, instead of investigating secondary structures (i.e., α-helix), our thermal stability (∼25 °C) and mechanical handling (mixing, vortexing, shaking) investigations with CaRGOS–hemoglobin formulations are focused on the analysis of heme groups of the four-polypeptide chain network of hemoglobin. UV-vis spectra, can detect loss or alterations in heme and is an effective indicator of changes in primary and secondary structure.20,21 In addition, losses in heme and the resulting change in the secondary structure are indicative of alteration of tertiary structure conformation, as each of the subunits are integral to the tertiary structure of the molecule.21 Therefore, in this manuscript, we have utilized hemoglobin as a model protein for our studies, however the process is compatible with any nucleotide or protein of choice. Fig. 1a shows the schematic of our CaRGOS process developed for encapsulation of a biomolecule such as hemoglobin. Typically, TMOS is hydrolyzed in an aqueous solution by imparting energy from a benchtop microwave for 30 s. The key hydrolysis reaction is highlighted in Fig. 1b. TMOS has 4 hydrolysable groups and the reaction with water results in the formation of silicic acid and methanol. The resulting solution is allowed to rest for 5 minutes to reach equilibrium at room temperature and to vent methanol, which is a byproduct of the hydrolysis reaction. A known concentration of CaRGOS is added to hemoglobin (in buffer), resulting in the condensation of hydrolyzed silica precursor as shown in Fig. 1b. Fig. 1c shows the Raman spectra of 5% silica precursor (TMOS): pre-hydrolysis, after hydrolysis (microwave for 30 s), and after condensation (addition of buffer). The theoretical peak positions of TMOS precursor, intermediates, silicic acid/dimers and methanol are expected at 640–650 cm−1, 673–725 cm−1, 750–780 cm−1 and 1020 cm−1.22 Experimentally, we observe a peak at 646 cm−1 for 10% TMOS/water solution prior to hydrolysis indicating the presence of intact Si(OCH3)4. Upon ∼30 s microwave exposure, the Si(OCH3)4 peak at 646 cm−1 disappears and an increase in Si(OH)4 and methanol peak was observed at 750 cm−1 and 1020 cm−1. Absence of intermediate peaks for partially hydrolyzed TMOS species at 673 cm−1, 697 cm−1, and 725 cm−1 indicate completed hydrolysis of Si(OCH3)4.22 Fig. S1–S3† display Raman spectra of serial-diluted TMOS (0.1–5.0) v/v % solutions, before and after the TMOS hydrolysis under microwave exposure. Upon addition of the buffer containing Hb, the intensity of methanol peak decreases due to dilution and we also observe emergence of a peak at 980 cm−1, which arises due to the presence of phosphate buffer, Fig. S4.† The resulting solution, undergoes gelation depending upon the concentration of the precursor, were stored for further analysis. We further performed IR analysis to monitor the formation of Si–O–SI and Si–OH bonds in the gels. IR spectra of the silica gels 0.5, 1, 2.5, 5 and 7.5 v/v% CARGOS are shown in Fig. ESI S5.† The wide band at 1100 cm−1 (to ∼1250 cm−1) is generated by contribution of the vibrational modes of –Si–O–Si– and –Si–OCH3. At 1015 cm−1 the formation of CH3OH and Si–OH are also observed. Peak intensity increases as the concentration of silica precursor is increased thus determining the extent of gelation.
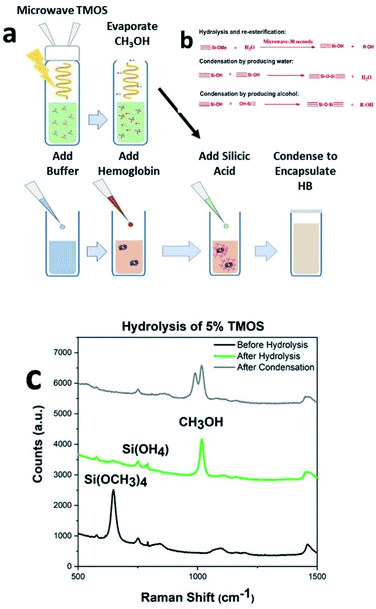 |
| Fig. 1 Synthesis and Raman characterization of CaRGOS formulations. (a and b) Schematic of CaRGOS formulations and encapsulation of hemoglobin for long-term room-temperature storage and (c) complete hydrolysis of 5.0 v/v % tetramethyl orthosilicate (TMOS) was demonstrated by Raman spectra with an elimination of TMOS peak (646 cm−1) and formation of methanol peak (1030 cm−1) after a standard microwave synthesis. | |
This complete self-sterile immobilization of any biospecimen can be achieved in less than 10 minutes utilizing only one chemical and a benchtop microwave. The process is extremely compatible with clinical settings and even for on-field operations. The integrity of hemoglobin in CaRGOS was evaluated using UV-vis spectroscopy. UV-vis spectroscopy is a rapid and routinely used method to validate structural stability of hemoglobin (λ = 406 nm; heme group) Fig. 2a shows a sharp UV-vis band (λ = 406 nm) of the prosthetic heme group (C34H32O4N4Fe) in aqueous hemoglobin solutions (0.01 w/v %; 0.5 M PB; pH 8.2) and in the CaRGOS (1.0–7.5) v/v % formulations immediately after immobilization. This absorbance band indicates the excellent initial stability of hemoglobin in both the CaRGOS and control samples. The intact absorbance band shown in Fig. 2a also supports that silica condensation and the presence of the methanol by product do not affect hemoglobin nativity.
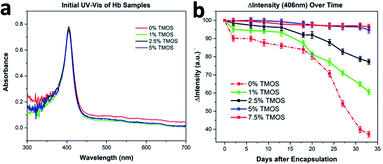 |
| Fig. 2 UV-vis analysis of hemoglobin content within CaRGOS formulations. (a) Incremental increase in the hemoglobin stability with incremental increase in CaRGOS concentrations (0–5.0) v/v %. An unaltered UV-vis absorbance band (406 nm) of heme group of hemoglobin framework is observed in CaRGOS formulations (5.0 v/v%). (b) Hemoglobin stability with incremental increase in CaRGOS concentrations (0–7.5) v/v %. An unaltered UV-vis absorbance band (406 nm) of heme group in hemoglobin framework is observed in CaRGOS formulations (5.0 and 7.5 v/v%). | |
While maintaining constant hemoglobin concentration (0.01 w/v%) and buffer environment (0.5 M PB, pH 8.2), the CaRGOS concentration (0.0–7.5) v/v % range were observed over a period of month. Relative to the control-group hemoglobin solutions (i.e., w/o CaRGOS), Fig. 2b shows a two-fold [CaRGOS (1.0 v/v %)] and three-fold [CaRGOS (2.5 v/v %)] hemoglobin stability in CaRGOS formulations. This demonstrates a CaRGOS-concentration dependent trend in determining the physical and chemical stability of hemoglobin. Fig. 2b shows that CaRGOS (5.0 and 7.5) v/v % solutions retained nearly ∼100% hemoglobin-stability up to 3 weeks and ∼95% stability for 33 days. Relatively high CaRGOS concentrations (5.0–7.5) v/v%, were, therefore, ideal for storing hemoglobin under room-temperature and mechanical-handling (i.e., mixing, vortexing) based conditions. Fig. 2b also shows that control samples (i.e., w/o CaRGOS) at room-temperature had a significant decrease in UV-vis absorbance: ∼10% in 1 week, ∼20% in 3 weeks and ∼63% in four weeks respectively.
Prolonged storage at refrigerated temperature and room-temperature of proteins is highly desirable for numerous medical applications. We performed prolonged storage (several months) studies in a similar format to the 33 day hemoglobin storage, described above. As shown in Fig. 2b, CaRGOS formulations (5.0 & 7.5 v/v %) had demonstrated exceptional hemoglobin storage capabilities over 1 month storage interval. However, the 5.0 v/v % formulation was preferentially chosen over 7.5 v/v % formulation towards investigating hemoglobin integrity over 210 days (7 month), attributing to an easier biospecimen passage/recovery through CaRGOS matrices, and less cost per sample. As shown in the Fig. 3a, our optimized CaRGOS [(5.0 v/v %) TMOS; 0.01 w/v % hemoglobin; 0.15 M PB, pH 8.2; 3.0 mL] solutions had demonstrated an unprecedented hemoglobin-stability (∼96%) up to 7 month period at 4 °C, under the non-sterile, room-temperature storage conditions. During prolonged refrigeration, the control group hemoglobin solutions (0.01 w/v %; 0.15 M PB; pH 8.2) also displayed robust stability (∼96%) up to the 40 day period, demonstrating the short-term stabilizing effect of refrigeration as well as the phosphate buffer environment on control group hemoglobin solutions. However, Fig. 3a shows escalated hemoglobin degradation over the long-term refrigeration period for control samples, with a significant loss of heme group (406 nm) absorbance (∼70%). Under room temperature conditions, Fig. 3b shows that 5% CaRGOS samples retained 47% absorbance over the 210 day time period, while control samples retained 3% absorbance under the same conditions. This supports the long-term storage capabilities of 5% CaRGOS under both ambient room temperature and refrigerated conditions.
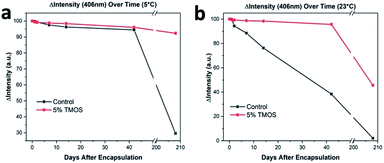 |
| Fig. 3 Excellent long-term stability of hemoglobin in CaRGOS formulations (5.0 v/v % TMOS; 0.01 w/v % hemoglobin; 0.15 M PB, pH 8.2) against control hemoglobin solutions (0.01 w/v % hemoglobin; 0.15 M PB, pH 8.2). (a) Refrigeration (5 °C) and (b) room-temperature (23 °C) conditions. | |
3.2 Polyethylene glycol (PEG) induced hemoglobin release in CaRGOS formulations
The development of a biocompatible release protocol is desirable as it allows lab technicians to run diagnostics on the extracted proteins. Post-encapsulation of hemoglobin within CaRGOS matrices, PEG was systematically added to all CaRGOS formulations, Fig. 4a. A quick re-dissolution of low-to-high viscous CaRGOS–hemoglobin formulations [(1.0–7.5) v/v % TMOS; 0.01 w/v % hemoglobin; 0.5 M PB, pH 8.2; 3.0 mL] was observed upon addition of polyethylene glycol [PEG (65 μM, 2 kDa)]. Upon centrifuging the dissolved CaRGOS samples and extracting the supinated solution, a three to five-fold increase in hemoglobin's UV-vis absorbance (406 nm) was observed in the resulting solution Fig. 4b. This large increment in the absorbance intensity of heme group [406 nm] is attributed to a synergistic hydrophilicity imparted by PEG (260 nm) to the CaRGOS formulations, indicating a facile passage and release of hemoglobin throughout the CaRGOS matrices without any loss of protein nativity as shown in Fig. 4. Particularly, an ideal ensilication matrix allows efficient bioanalyte immobilization (i.e., encapsulation entrapment or collaterally depositing) and a facile passage without any physical rupture. Therefore, our highly porous and moderately viscous CaRGOS formulations meets these standards, due to the long-term storage capabilities of CaRGOS as shown in Fig. 2b, and the biocompatible PEG release protocol as shown in Fig. 4.
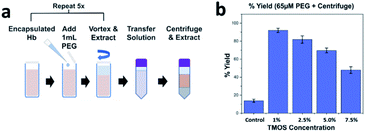 |
| Fig. 4 Polyethylene glycol (PEG) induced hemoglobin content release. (a) Schematic of PEG addition to the CaRGOS formulation for facile hemoglobin extraction and (b) significant hemoglobin release in CaRGOS formulations (1.0–7.5) v/v% upon PEGylation. | |
3.3 Raman analysis of CaRGOS formulations
Fig. 5 shows analogous Raman spectra of the CaRGOS formulations (5.0 v/v % TMOS; PB pH 8.0) with and w/o hemoglobin at days 7, 14 and 21 respectively. The Raman peaks of TMOS solution was assigned to 646 cm−1, dimerized silica or silicic acid to 830 cm−1 and the intense methanol C–O stretch to 1030 cm−1 respectively.22 Similar peak intensities over 21 days is attributed to the robust physio-chemical stability of CaRGOS dispersions under room-temperature and mechanical handling (i.e., mixing, shaking, vortexing) conditions. Also, the unaltered peak intensities of CaRGOS formulations, with and without hemoglobin are tentatively attributed to the unique shape-recognition capabilities of silica nanostructures. Notably, the CaRGOS nanoformulations could potentially deposit around hemoglobin and match its shape/conformation, resulting in similar rotational and vibrational fingerprints of the CaRGOS formulations, with and without hemoglobin.3
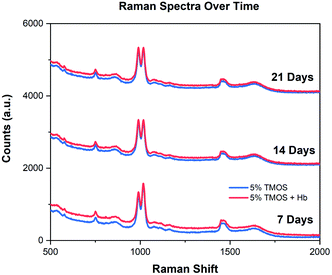 |
| Fig. 5 Raman spectra of CaRGOS. An unaltered Raman spectrum of CaRGOS (5.0 v/v %) formulations (with and without hemoglobin) over 21 days. | |
4. Conclusions
We developed a technologically and clinically relevant TMOS based sol gels called CaRGOS using a standard bench top microwave. These gels were utilized to demonstrate effective protein storage over long durations of time. The model protein used in the study was hemoglobin and its stability was monitored using UV-vis measurements. In addition, stability of sol was determined using Raman Spectroscopy. Up to 5.0 v/v %, CaRGOS enhance the shelf life as compared to control samples but lose their efficacy over time. High concentration CaRGOS (5.0 & 7.5) v/v % formulations, displayed excellent stability of hemoglobin protein over 33 days at room temperature and 7 months at 4 °C. Furthermore, we developed a biocompatible PEG based protocol to release the proteins from the CaRGOS with extremely high yields. Based on our findings, we propose that the final optimal protein storage condition is utilization of (5.0 v/v %) TMOS; 0.01 w/v % hemoglobin; 0.15 M PB, pH 8.2; 3.0 mL, that had demonstrated an unprecedented protein integrity of ∼95% at ambient (1 month) and ∼96% (7 months) under refrigeration conditions. Using current PEG protocol, the release efficiency is 75%, which can be further optimized.
The results indicate that CaRGOS have enormous potential to stabilize biomolecules. The scientific insight gained in the study suggests that if the pI of the protein in below the pH of the CaRGOS, it will achieve stabilization and would be amenable to extraction. This robust storage, transport, and extraction are the keystone of this innovation, and present a technology that can encapsulate a plethora of biospecimens including proteins and nucleotides as most of them exhibit a pI of less than 7. Thus, the clinical applications of CaRGOS are vast when compared to its predecessors and present a feasible alternative to conventional cryopreservation platforms.
Conflicts of interest
There are no conflicts to declare.
Acknowledgements
Authors acknowledge financial support from the Office of the Executive Vice President for Research and Innovation at the University of Louisville (UofL). The author also acknowledges financial support from National Cancer Institute (NCI) exploratory/development grant [ID: grant number: 1R21CA251042-01]. Gupta acknowledge the startup funds from University of Louisville. We acknowledge Nanotherapeutics laboratory, Bioengineering Department (UofL) for sterilized biological hood/accessories support. Author contributions: Corresponding authors Gautam Gupta* and Robert S. Keynton* conceived the idea. The first author Boylan developed the synthetic and experimental designs and performed the UV-vis and Raman experiments. The second author Chauhan co-wrote the manuscript with Gupta. Bansal performed Raman spectroscopy on the sol–gel formulations. All authors discussed the results and contributed to the final manuscript.
References
- T. Coradin and J. Livage, Acc. Chem. Res., 2007, 40, 819–826 CrossRef CAS PubMed.
- W. Xiaolin, A. Nada Ben, S. A. Gisela, V. T. Maria, H. Christophe, F. D. Martin and C. Thibaud, Curr. Top. Med. Chem., 2015, 15, 223–244 CrossRef PubMed.
- Y.-C. Chen, T. Smith, R. H. Hicks, A. Doekhie, F. Koumanov, S. A. Wells, K. J. Edler, J. van den Elsen, G. D. Holman, K. J. Marchbank and A. Sartbaeva, Sci. Rep., 2017, 7, 46568 CrossRef PubMed.
- P. J. Calabretta, M. C. Chancellor, C. Torres, G. R. Abel, C. Niehaus, N. J. Birtwhistle, N. M. Khouderchah, G. H. Zemede and D. K. Eggers, J. Funct. Biomater., 2012, 3, 514–527 CrossRef CAS PubMed.
- B. G. Cha, J. H. Jeong and J. Kim, ACS Cent. Sci., 2018, 4, 484–492 CrossRef CAS PubMed.
- Y. Yang, J. Wan, Y. Niu, Z. Gu, J. Zhang, M. Yu and C. Yu, Chem. Mater., 2016, 28, 9008–9016 CrossRef CAS.
- J. Tu, A. L. Boyle, H. Friedrich, P. H. H. Bomans, J. Bussmann, N. A. J. M. Sommerdijk, W. Jiskoot and A. Kros, ACS Appl. Mater. Interfaces, 2016, 8, 32211–32219 CrossRef CAS PubMed.
- A. M. Chen, M. Zhang, D. Wei, D. Stueber, O. Taratula, T. Minko and H. He, Small, 2009, 5, 2673–2677 CrossRef CAS PubMed.
- H. Meng, M. Liong, T. Xia, Z. Li, Z. Ji, J. I. Zink and A. E. Nel, ACS Nano, 2010, 4, 4539–4550 CrossRef CAS PubMed.
- L. Pascual, C. Cerqueira-Coutinho, A. García-Fernández, B. de Luis, E. S. Bernardes, M. S. Albernaz, S. Missailidis, R. Martínez-Máñez, R. Santos-Oliveira, M. Orzaez and F. Sancenón, Nanomedicine, 2017, 13, 2495–2505 CrossRef CAS PubMed.
- P. Zhang, F. Cheng, R. Zhou, J.-T. Cao, J. Li, C. Burda, Q. Min and J.-J. Zhu, DNA-Hybrid-Gated Multifunctional Mesoporous Silica Nanocarriers for Dual-Targeted and MicroRNA-Responsive Controlled Drug Delivery**, 2014 Search PubMed.
- G. Gupta, S. B. Rathod, K. W. Staggs, L. K. Ista, K. Abbou Oucherif, P. B. Atanassov, M. S. Tartis, G. A. Montaño and G. P. López, Langmuir, 2009, 25, 13322–13327 CrossRef CAS PubMed.
- G. Gupta, P. Atanassov and G. P. López, Biointerphases, 2006, 1, 6–10 CrossRef CAS PubMed.
- G. Gupta, J. G. Duque, S. Doorn and A. M. Dattelbaum, MRS Proceedings, 2010, 1258, 1258 CrossRef.
- G. Gupta, A. Kearns, M. Bansal, T. Kalbfleisch, A. Keller, K. Ellison, R. Chauhan and M. Ghorbanian, Environ. Sci.: Water Res. Technol., 2021 10.1039/d1ew00081k.
- W. Han, L. K. Ista, G. Gupta, L. Li, J. M. Harris and G. P. López, in Handbook of Nanomaterials Properties, ed. B. Bhushan, D. Luo, S. R. Schricker, W. Sigmund and S. Zauscher, Springer Berlin Heidelberg, Berlin, Heidelberg, 2014, pp. 963–993, DOI:10.1007/978-3-642-31107-9_30.
- E. Audain, Y. Ramos, H. Hermjakob, D. R. Flower and Y. Perez-Riverol, Bioinformatics, 2016, 32, 821–827 CrossRef CAS PubMed.
- K. L. Shaw, G. R. Grimsley, G. I. Yakovlev, A. A. Makarov and C. N. Pace, Protein Sci., 2001, 10, 1206–1215 CrossRef CAS PubMed.
- B. Raynal, P. Lenormand, B. Baron, S. Hoos and P. England, Microb. Cell Fact., 2014, 13, 180 CrossRef PubMed.
- M. Goodarzi, A. A. Moosavi-Movahedi, M. Habibi-Rezaei, M. Shourian, H. Ghourchian, F. Ahmad, M. Farhadi, A. A. Saboury and N. Sheibani, Spectrochim. Acta, Part A, 2014, 130, 561–567 CrossRef CAS PubMed.
- Y. Zhu, G. Cheng and S. Dong, Biophys. Chem., 2002, 97, 129–138 CrossRef CAS PubMed.
- T. W. Zerda and G. Hoang, J. Non-Cryst. Solids, 1989, 109, 9–17 CrossRef CAS.
Footnotes |
† Electronic supplementary information (ESI) available. See DOI: 10.1039/d1ra00987g |
‡ These authors contributed equally to this work. |
|
This journal is © The Royal Society of Chemistry 2021 |
Click here to see how this site uses Cookies. View our privacy policy here.