DOI:
10.1039/D1RA00805F
(Paper)
RSC Adv., 2021,
11, 12710-12719
The improvement in hole-transporting and electroluminescent properties of diketopyrrolopyrrole pigment by grafting with carbazole dendrons†
Received
30th January 2021
, Accepted 15th March 2021
First published on 1st April 2021
Abstract
Diketopyrrolopyrrole (DPP) pigments are essential and have been intensively exploited as building-blocks for the synthesis of organic semiconducting polymers and small molecules; however, DPP derivatives as emissive materials for electroluminescent (EL) devices have rarely been explored. In this work, a series of new DPP derivatives grafted with carbazole dendrons in a non-conjugated fashion using an amide linkage was designed to improve the performance of DPP in EL devices. Three DPP derivatives (G0DPP, G1DPP and G2DPP) bearing di(p-chlorophenyl)-DPP (Pigment Red 254) as the core substituted with a hexyl chain, N-hexyl carbazole and N-hexyl-N′-9,3′:6′,N′′-tercarbazole, respectively, were synthesized to afford improved hole-transporting properties without affecting the photophysical and electronic properties of the DPP core. The synthesized DPP derivatives displayed an intense yellow fluorescence emission peaked at 536 nm with an absolute photoluminescence quantum yield close to unity in solution. The hole-transporting capability of molecules was improved when carbazole dendrons were incorporated, which increased with an increase in the generation of substituent carbazole dendrons in the order of G0DPP < G1DPP < G2DPP. Significantly, the use of G2DPP, showing the highest hole mobility, in an EL device yielded a strong and stable yellow emission peaked at 556 nm (CIE x, y color coordinates of (0.45, 0.53)) with a brightness of 3060 cd m−2, maximum luminous efficiency of 9.24 cd A−1 and a maximum EQE of 3.11%.
Introduction
The application of organic materials in organic light-emitting diodes (OLEDs) received significant interest due to their successful application in full-color displays (mobile phones, digital cameras, and televisions) and potential use as solid-state lighting sources after it was first reported by Tang and Vanslyke in 1987.1–5 To realize highly efficient electroluminescent (EL) performances in OLED devices, thermally evaporated multilayer devices integrated with efficient charge injection and charge transporting layers are fabricated.6–8 Conversely, solution processing can offer simpler and more cost-effective processing and is suitable for large-area roll-to-roll production.9 Nonetheless, the further advancement of solution-processed OLED performances is still very challenging for competitive lighting devices. One key aspect for achieving high-performance OLEDs is the design and synthesis of advanced light-emitting materials with suitable electrical and optical properties to fully use excitons for efficient energy conversion, which is still a challenge.10–12
Diketopyrrolopyrroles (DPPs) are a family of high-performance industrial pigments with remarkable thermal stability and excellent photostability.13 They have been widely used as high-performance pigments such as inks, paints, and plastics.13 Furthermore, these characteristics are also desirable for active materials in mass-producible low-cost organic electronics.14 DPPs have several advantages, for example, they are strong acceptors that exhibit a high molar absorption coefficient and high fluorescence quantum yield (ΦPL) and can undergo several synthetic modifications.15 Thus, based on these features, in recent years DPPs have emerged as one of the most successful building blocks in polymers, oligomers, and small molecules for the synthesis of high-mobility transistor materials,16–20 sensing materials,21–24 bioimaging fluorescent probes,25,26 two-photon absorption materials27,28 and materials for the fabrication of photovoltaic devices.29–32 Many efficient donor and acceptor materials used in high-performance organic photovoltaics (OPV) are derivatives of DPP.33–36 However, despite their intense emission in solution, DPP derivatives as emissive materials in OLEDs are found to be rare.37–41 Most of them are DPP-containing polymers, but the small DPP molecule has not been investigated to date. This is because the planar structure of DPP induces strong intermolecular π–π stacking, leading to dissipative intermolecular charge transfer, aggravated charge recombination, and low fluorescence emission in the solid state.
Hence, in this work, we report our attempt to design efficient emissive materials based on DPP for application in solution-processed OLEDs. As depicted in Scheme 1, di(p-chlorophenyl)-DPP (Pigment Red 254) was utilized as the DPP emissive core owing to its strong fluorescence emission and inexpensive commercial availability.42 The carbazole dendron was chosen as the grafting moiety given that the carbazole unit has a high triplet energy (3.0 eV)43 and excellent hole-transporting ability. Carbazole has also been extensively exploited as a building block for the design of wide-band-gap host materials44,45 and multifunctional phosphors.46–48 Additionally, the carbazole dendron was varied to tune the properties of the molecule. Besides, carbazole dendrons have been effectively used to construct many highly efficient hole-transporting materials49–51 and hole-transporting light-emitting materials52–54 for OLEDs. Meanwhile, an alkyl linkage was selected to connect the nitrogen atoms in the DPP unit and dendron, aiming to retain the optical and electronic properties of DPP and achieve convenient synthesis. The hexyl chain was favourably used because longer chains may separate the dendron and core too far from each other, resulting in reduced effects (energy transfer or electron transfer) between the two moieties, while shorter chains may cause some difficulties in the synthesis. It has been reported that carbazole-containing alkyl bromide is unstable, and thus undergoes elimination reactions to form inactive olefins.55 The hexyl linkage would also give decent solubility to the dendrimers in common organic solvents, allowing the materials to be fabricated via solution-based processes. Thus, employing this design strategy, we achieved the synthesis of efficient green phosphorescent iridium dendrimers, where the affixing carbazole dendrons were anticipated to improve the hole-transporting capability and function as a host for the iridium emissive core. Accordingly, furnishing high and balanced charge carrier ability to the materials should pave the way to improve the EL performance of DPP-based OLEDs. Indeed, the G2DPP-based device achieved the best EL performance with a strong and stable yellow emission with CIE x, y color coordinates of (0.45, 0.53) and EQE as high as 3.11% (9.24 cd A−1).
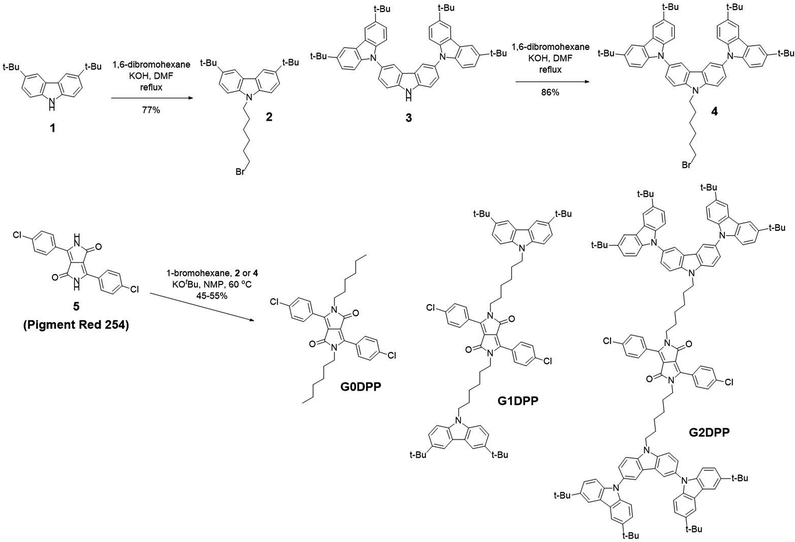 |
| Scheme 1 . Synthesis of diketopyrrolopyrrole derivatives (G0DPP–G2DPP). | |
Experimental
Materials and methods
All chemicals were supplied and used as received without further purification. Pigment Red 254 was obtained from Tokyo Chemical Industry (TCI) Chemicals. 3,6-Di-tert-butylcarbazole (1) and 3,3′′,6,6′′-tetra-tert-butyl-N′,3′:6′,N′′-tercarbazole (3) were synthesized following the literature methods.50,52
1H and 13C NMR spectra were acquired on a Bruker Ascend III (600 MHz) spectrometer in CDCl3 solvent. High-resolution mass spectra were analyzed using a Bruker Autoflex Speed mass spectrometer. Infrared spectra were recorded on a Perkin Elmer FTIR spectrophotometer. UV-Vis absorption spectra were measured using a Perkin Elmer Lambda 1050 UV/Vis/NIR spectrometer. AFM images were taken using a Park System model NX-10 instrument operated in the tapping mode under ambient conditions. TGA analyses were performed using a Perkin-Elmer TGA Pyris 1 instrument at a heating rate of 10 °C min−1 under an N2 flow. PL spectra and decay profiles were obtained using an Edinburgh Instruments FLS980 spectrometer. Absolute photoluminescence quantum yields (ΦPL) were measured on an Edinburgh Instruments FLS980 spectrometer integrated with a calibrated integrating sphere. Electrochemical analyses were done on an Autolab PGSTA101 with a three-electrode setup (Pt, glassy carbon, and Ag/AgCl) in CH2Cl2 and n-Bu4NPF6 (0.1 M) as the supporting electrolyte. Melting points were measured using a Krüss KSP1N melting point meter and are uncorrected.
Quantum chemical calculations were performed using the Gaussian 09 software.56 The ground-state geometries, HOMO and LUMO distributions, and HOMO, LUMO energy levels were calculated using the B3LYP/6-31G (d,p) basis set in CH2Cl2 solvent.
OLED devices (ITO/PEDOT:PSS (35 nm)/EML (35 nm)/TAZ (40 nm)/LiF (0.5 nm)
:
Al (150 nm)) were fabricated on patterned ITO glass substrates (12 Ω sq−1). The substrates were pre-cleaned carefully and cured with UV/O3 for 2 min. As the hole injection layer, a 40 nm-think PEDOT:PSS thin film was spin-coated on top from a 1.2 wt% aqueous dispersion of PEDOT:PSS (CLEVIOSTM P VP Al 4083) at a spinning speed of 5000 rpm for 30 s followed by drying at 120 °C for 15 min. Subsequently, thin films with a thickness of 35 nm of G0DPP–G2DPP 3 wt% in mCP host as the EML were spin-coated on top from toluene solution of EML (2% w/v) at a spin speed of 2500 rpm for 30 s. The thickness of all the spin-coated films was assessed using a Dektak XTL stylus profiler. Then TAZ as an electron transporting layer of 40 nm, LiF of 0.5 nm, and aluminum of 150 nm were thermally evaporated using a Kurt J. Lasker Mini SPECTROS 100 thin film deposition system. The thin film thicknesses were monitored and controlled using a quartz crystal. All devices were unencapsulated and measured under ambient conditions in the dark. They were analyzed using a Keithley 2400 source meter, a Hamamatsu Photonics PMA-12 multi-channel analyzer, and an integrating sphere equipped with a Hamamatsu Photonics C9920-12 EQE Measurement System.
Synthesis and characterization
N-(6-Bromohexyl)-3,6-di-tert-butyl-carbazole (2). 1,6-Dibromohexane (2.8 mL, 18.0 mmol) was added to a solution of compound 1 (1.0 g, 3.6 mmol) and KOH (0.81 g, 14.4 mmol) in DMF (50 mL) at 0 °C. The mixture was stirred at room temperature for 24 h and then diluted with water (100 mL) and extracted with CH2Cl2 (3 × 50 mL). The combined organic layer was washed with water (50 mL), brine solution (50 mL), dried over anhydrous Na2SO4, filtered and concentrated under reduced pressure. The crude product was purified by column chromatography (CH2Cl2/hexane) to afford a white solid (1.22 g, 77%). Mp 120–122 °C; FTIR (ATR, cm−1) ν 2937, 2842, 1482, 1376, 1074, 831, 694. 1H-NMR (600 MHz, CDCl3, ppm) δ 8.10 (s, 2H), 7.50 (d, J = 8.4 Hz, 2H), 7.30 (d, J = 8.4 Hz, 2H), 4.25 (t, J = 7.2 Hz, 2H), 3.36 (t, J = 6.6 Hz, 2H), 1.81–1.90 (m, 4H), 1.44 (s, 18H), 1.41–1.39 (m, 4H); 13C-NMR (150 MHz, CDCl3, ppm) δ 141.5, 139.0, 123.3, 122.7, 116.3, 108.0, 43.0, 34.7, 33.7, 32.6, 32.4, 32.1, 29.0, 28.0, 26.5; m/z (HRMS MALDI-TOF) C26H36BrN calcd 441.2031; found 441.3331 (M+).
N-(6-Bromohexyl)-3,3′′,6,6′′-tetra-tert-butyl-N′,3′:6′,N′′-tercarbazole (4). It was synthesized from compound 3 using the same conditions as that for 2 and obtained as a white solid (1.06 g, 86%). Mp 293–295 °C; FTIR (ATR, cm−1) ν 2935, 2847, 1485, 1375, 1074, 831, 694. 1H-NMR (600 MHz, CDCl3, ppm) δ 8.18 (s, 2H), 8.15 (s, 4H), 7.64 (m, 4H), 7.44 (m, 4H), 7.31 (m, 4H), 4.49 (t, J = 7.2 Hz, 2H), 3.43 (m, 2H), 2.09–1.91 (m, 4H), 1.46 (s, 36H), 1.44 (m, 4H) ppm; 13C-NMR (150 MHz, CDCl3, ppm) δ 142.5, 140.3, 139.9, 129.9, 125.7, 123.5, 123.4, 123.0, 119.5, 116.2, 109.9, 109.1, 43.5, 34.7, 33.7, 32.6, 31.9, 29.1, 28.0, 27.3, 26.6 ppm; m/z (HRMS MALDI-TOF) C58H66BrN3 calcd 883.4440; found 883.605 (M+).
3,6-Bis(4-chlorophenyl)-2,5-dihexyl-2,5-dihydropyrrolo[3,4-c]pyrrole-1,4-dione (G0DPP). Pigment Red 254 (5) (0.10 g, 0.28 mmol), KOtBu (0.0691 g, 0.62 mmol) and NMP (15 mL) were heated to 60 °C. 1-Bromohexane (0.12 mL, 0.84 mmol) was then added, and the mixture was stirred at 60 °C for 18 h. After cooling to room temperature, it was diluted with water (50 mL) and extracted with CH2Cl2 (3 × 50 mL). The combined organic layer was washed with water (50 mL), brine solution (50 mL), dried over anhydrous Na2SO4, filtered and concentrated under reduced pressure. The crude product was purified by column chromatography (CH2Cl2/hexane) to afford an orange solid (0.080 g, 55%). Mp 183 °C; FTIR (ATR, cm−1) ν 2936, 2847, 1686, 1612, 1483, 1374, 1076, 831, 695. 1H-NMR (600 MHz, CDCl3, ppm) δ 7.76 (d, 4H, J = 8.4 Hz), 7.50 (d, 4H, J = 8.4 Hz), 3.72 (t, 4H, J = 7.5 Hz), 1.56 (m, 4H), 1.21 (m, 12H), 0.83 (t, 6H); 13C-NMR (150 MHz, CDCl3, ppm) δ 162.5, 147.4, 137.4, 130.0, 129.3, 136.6, 110.0, 41.9, 31.2, 29.7, 29.4, 26.4, 22.5, 13.9; m/z (HRMS MALDI-TOF) C30H34Cl2N2O2 calcd 524.1997; found 524.4251 (M+).
3,6-Bis(4-chlorophenyl)-2,5-bis(6-(3,6-di-tert-butyl-carbazol-N-yl)hexyl)-2,5-dihydropyrrolo[3,4-c]pyrrole-1,4-dione (G1DPP). It was synthesized from compound 2 (0.372, 0.84 mmol), KOtBu (0.0691 g, 0.62 mmol) and Pigment Red 254 (5) (0.10 g, 0.28 mmol) using the same conditions as that for G0DPP and obtained as an orange solid (0.15 g, 45%). Mp 192 °C; FTIR (ATR, cm−1) ν 2950, 2868, 2311, 1673, 1605, 1475, 1353, 1082, 797, 716. 1H-NMR (600 MHz, CDCl3, ppm) δ 8.09 (s, 4H), 7.70 (d, 4H, J = 8.4 Hz), 7.45–7.49 (m, 8H), 7.23 (d, J = 8.4 Hz, 4H), 4.18 (t, 4H, J = 6.8 Hz), 3.69 (t, 4H, J = 7.3 Hz), 1.78 (m, 4H), 1.53 (m, 4H), 1.45 (m, 36H), 1.24–1.30 (m, 8H). 13C-NMR (150 MHz, CDCl3, ppm) δ 162.4, 147.3, 141.5, 138.9, 137.4, 129.9, 129.3, 126.4, 123.2, 122.7, 116.3, 109.9, 107.9, 42.9, 41.8, 34.7, 32.1, 29.3, 29.0, 26.8, 26.6; m/z (HRMS MALDI-TOF) C70H80Cl2N4O2 calcd 1078.5658; found 1078.6823 (M+).
3,6-Bis(4-chlorophenyl)-2,5-bis(6-(3,3′′,6,6′′-tetra-tert-butyl-[N,3′:6′,N′′-tercarbazol]-N′-yl)hexyl)-2,5-dihydropyrrolo[3,4-c]pyrrole-1,4-dione (G2DPP). It was synthesized from compound 4 (0.372, 0.42), KOtBu (0.035 g, 0.31 mmol) and Pigment Red 254 (5) (0.05 g, 0.14 mmol) using the same conditions as that for G0DPP and obtained as an orange solid (0.083 g, 52%). Mp 247 °C; FTIR (ATR, cm−1) ν 2963, 2854, 2359, 1673, 1503, 1258, 811, 606. 1H-NMR (600 MHz, CDCl3, ppm) δ 8.17 (s, 4H), 8.15 (s, 8H), 7.73 (d, 4H, J = 8.4 Hz), 7.55–7.61 (m, 8H), 7.42–7.46 (d, 12H), 7.30 (d, 8H, J = 8.4 Hz), 4.40 (t, 4H, J = 7.2 Hz), 3.76 (m, 4H), 1.94–1.99 (m, 4H), 1.61–1.65 (m, 4H), 1.26–1.46 (m, 80H); 13C-NMR (150 MHz, CDCl3, ppm) δ 162.4, 147.3, 142.5, 140.3, 139.9, 137.5, 129.9, 129.4, 126.4, 125.7, 123.5, 123.1, 119.5, 116.2, 110.0, 109.8, 109.1, 43.4, 41.8, 34.7, 32.1, 29.4, 29.0, 26.8, 26.6; m/z (HRMS MALDI-TOF) C134H140Cl2N8O2 calcd 1963.0476; found 1963.0941 (M+).
Results and discussion
Synthesis and characterization
The synthesis of di(p-chlorophenyl)-DPP (Pigment Red 254) functionalized with carbazole dendrons is outlined in Scheme 1. Firstly, the synthesized 3,6-di-tert-butylcarbazole (1) and 3,3′′,6,6′′-tetra-tert-butyl-N′,3′:6′,N′′-tercarbazole (3)50,52 were alkylated with an excess amount of 1,6-dibromohexane in the presence of KOH as a base in dimethylformamide to afford the monoalkylated molecules 2 and 4 in good yield, respectively. In the final step, alkylation of Pigment Red 254 (5) with 1-bromohexane, compound 2, or compound 4 under basic conditions of KOtBu in N-methyl-2-pyrrolidone gave the desired products G0DPP, G1DPP, and G2DPP as yellow-orange solids in moderate yields, respectively. All molecules were chemically characterized by 1H NMR, 13C NMR, and high-resolution MALDI-TOF mass spectra, agreeing well with their proposed structures (Fig. S3–S7, ESI†). Due to the existence of a hexyl chain and carbazole dendron, the newly synthesized di(p-chlorophenyl)-DPP derivatives displayed good solubility in most organic solvents and could be processed into thin films using simple solution-processed casting methods.
Theoretical calculation
The electronic structure and molecular geometry were investigated using the density functional theory (DFT) method with the B3LYP hybrid function and 6-31G(d) basis set as implemented in Gaussian 09.56 The optimized geometries of G0DPP–G2DPP revealed that the di(p-chlorophenyl)-DPP core adopts a planar conformation (Fig. S1, ESI†). In case of G1DPP and G2DPP, the core is sandwiched by pendant carbazole dendrons. The molecules become more hindered as the generation of dendrons increased due to the strongly twisted structure of each carbazole unit in the dendron. These structural characteristics can influence the physical properties of the material. The lowest unoccupied molecular orbital (LUMO) energy level of all the molecules was calculated to be approximately the same (−2.63 to −2.70 eV), while their highest occupied molecular orbital (HOMO) energy levels varied (−5.36 to −5.01 eV). A more detailed picture comparing the HOMO and LUMO for all three molecules is depicted in Fig. 1. As is evident from this picture, the LUMO orbitals of all the molecules are located on the electron-deficient di(p-chlorophenyl)-DPP core. The distribution of the π-electrons in the HOMO of G0DPP is on the DPP orbitals, whereas the π-electrons are delocalized over the entire carbazole moieties in the case of G1DPP and G2DPP. Accordingly, the HOMO levels decreased with an increase in the generation of dendrons, while the LUMO levels remained unchanged. The low HOMO levels of both G1DPP (−5.13 eV) and G2DPP (5.01 eV) imply that they are susceptible to hole injection from the indium tin oxide (ITO) anode when fabricated as an emissive layer (EML) in OLED devices.
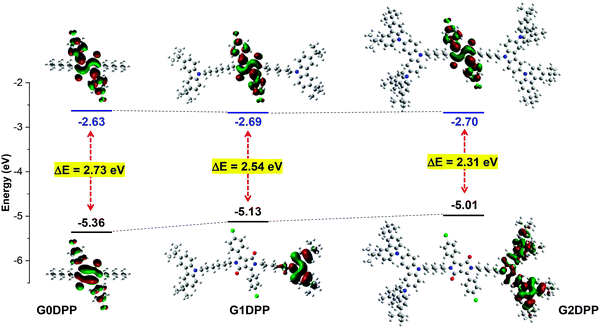 |
| Fig. 1 Frontier-orbital distribution, HOMO and LUMO energy levels of G0DPP–G2DPP simulated using the B3LYP/6-31G(d) basis set. | |
Optical properties
The UV-visible absorption spectra of the di(p-chlorophenyl)-DPP derivatives in toluene solution (10−6 M) are shown in Fig. 2a. The detailed optical data are summarized in Table 1. All the spectra showed the main broad absorption band maximum at 479 nm, which can be assigned to the π–π* transition of the di(p-chlorophenyl)-DPP moiety (Fig. 2a).42 In G1DPP and G2DPP, a sharp absorption band at a lower wavelength (298 nm), corresponding to the π–π* transition of the carbazole moiety, was also observed. Noticeably, the DDP absorption peak intensities of all the molecules barely changed (Fig. 2a and Table 1), suggesting that grating the DPP core with electron-donating carbazole dendrons in a non-conjugated manner does not affect its electronic properties. This result is consistent with the constant in LUMO levels observed in the DFT calculation. Moreover, the intensity of the absorption peak at 298 nm increases from G1DPP to G2DPP, which is related to the increased number of carbazole units in the substituent moieties. Thus, the optical band gap (Egopt) of these molecules will not be affected. As depicted in Table 1, the Egopt values calculated from the onset of the absorption spectra are 2.33 eV for G0DPP, 2.34 eV for G1DPP, and 2.35 eV for G2DPP. In solution, G0DPP–G2DPP demonstrated a strong yellow emission with an absolute PL quantum yield (ΦPL) close to unity (Table 1). They showed identical photoluminescence (PL) spectra with peaks at 536 and 578sh nm, which are attributed to the emission of the di(p-chlorophenyl)-DPP core (Fig. 2a). The PL spectra of G1DPP and G2DPP are excitation wavelength independent, where excitation occurs either at the carbazole moieties (at 298 nm) or DDP core (at 479 nm), and thus the PL spectra obtained are identical. The results suggest that energy or excitons can efficiently be transferred from the carbazole moiety to the emissive DPP unit. In the neat film, the PL spectra displayed a large bathochromic-shift compared to the PL in solution, indicating the substantial aggregation of the molecule in the film state. G0DPP, in particular, its PL spectrum was red-shifted to 610 nm and became broad with a featureless band. G2DPP bearing a 2nd carbazole dendron exhibited weaker intermolecular aggregation. Consequently, the ΦPL values of these compounds doped in the neat film were as low as 9–20% (Table 1). The transient PL decay spectra of G0DPP–G2DPP in solution and neat film are shown in Fig. 2b and c, and the lifetimes are listed in Table 1, respectively. In both conditions, all the molecules displayed only prompt fluorescence with a short lifetime (ns). It is interesting to note that the PL lifetime of G2DPP in the neat film (13 ns) was somewhat longer than in solution. This can be attributed to the fact that the bulky molecular structure of the 2nd carbazole dendron hampers the π-stacked aggregation of the di(p-chlorophenyl)-DPP core in the solid state, leading to less planarization of the molecule, and thus giving rise to a long PL lifetime.57 Thus, to improve the ΦPLs of G0DPP–G2DPP in the film state, they were doped in the mCP host matrix. With the optimum doping concentration of 3 wt% of the compound in CBP, the thin films exhibited strong yellow emissions, with the ΦPL values insignificantly enhanced up to 71% (Table 1). As shown in Fig. 2d, the PL spectra of all the doped thin films display pure dopant emissions peaked at 553–557 nm, which are slightly blue-shifted compared to that in the PL spectrum of the corresponding neat film. These results imply that doping of the compounds in the mCP host matrix well suppresses molecular aggregation and restores their high ΦPL as that in solution.
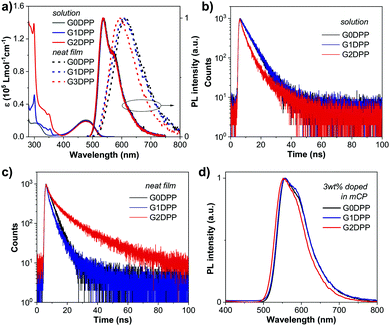 |
| Fig. 2 (a) UV-vis absorption spectra in toluene solution and normalized PL spectra of G0DPP–G2DPP in toluene solution and thin film spin-coated on quartz substrates of G0DPP–G2DPP. Transient PL decay spectra of G0DPP–G2DPP (b) in toluene solution and (c) as neat films. (d) Normalized PL spectra of 3 wt% G0DPP–G2DPP doped in mCP. | |
Table 1 Key physical data of the synthesized compounds
Compd |
λabs (log ε)a (nm, M−1 cm−1) |
λem (nm) sola/filmb/dfilmc |
τd (ns) solb/filmb |
T5de (°C) |
E1/2 vs. Ag/Ag+f (V) |
ΦPLg (%) sola/filmb/dfilmc |
Egopth (eV) |
HOMO/LUMO/Eelegi (eV) |
LUMOj (eV) |
Hole mobilityk (cm2 V−1 s−1) |
Measured in toluene. Measured as the neat film. Measured as 3 wt% doped in mCP thin film. Transient PL decay time. Analysed by TGA at a heating rate of 10 °C min−1 under an N2 flow. Obtained from CV at a scan rate of 50 mV s−1 in CH2Cl2 and n-Bu4NPF6 as the electrolyte. Absolute PL quantum yield measured by integrating sphere. Estimated from the optical absorption edge, Egopt = 1240/λonset. Calculated using HOMO = −(4.44 + Eooxnset); LUMO = −(4.44 + Eorenset); Eeleg = Eooxnset − Eorenset, where Eooxnset is the onset potential of the oxidation and Eorenset is the onset potential of the reduction. LUMO = HOMO − Egopt. Obtained from hole-only device (ITO/PEDOT:PSS/Compd/MoO3/Al). |
G0DPP |
479 (4.31) |
536/610/557 |
6/4 |
356 |
−1.73, −1.2, 1.23, 1.47 |
96/9/67 |
2.33 |
−5.69/−3.33/2.36 |
3.34 |
2.00 × 10−6 |
G1DPP |
298 (4.71), 479 (4.29) |
536/608/557 |
6/4 |
409 |
−1.75, −1.19, 1.15, 1.52 |
95/12/71 |
2.34 |
−5.51/−3.33/2.18 |
3.16 |
2.56 × 10−6 |
G2DPP |
298 (5.14), 479 (4.27) |
536/595/553 |
6/13 |
432 |
−1.76, −1.18, 1.02, 1.20, 1.50 |
92/20/70 |
2.35 |
−5.29/−3.33/1.96 |
2.94 |
3.35 × 10−5 |
Thermal and electrochemical properties and hole mobility
The thermal properties of G0DPP–G2DPP were investigated by thermal gravimetric analysis (TGA), which revealed that all the molecules exhibit high thermal stability with decomposition temperatures at 5% weight loss (T5d) of over 356 °C (Fig. 3a and Table 1).
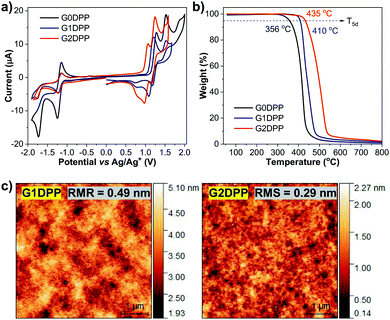 |
| Fig. 3 (a) TGA plots of G0DPP–G2DPP analyzed under N2 at a heating rate of 10 °C min−1. (b) CV curves of G0DPP–G2DPP measured in CH2Cl2 solution containing 0.1 M Bu4NPF6 at a scan rate of 50 mV s−1. (c) AFM images of G1DPP and G2DPP 3 wt% doped in mCP thin films. | |
The electrochemical properties of the samples were explored by cyclic voltammetry (CV) to estimate the oxidation, reduction potentials, and HOMO–LUMO energy levels of each molecule. The experiments were performed in CH2Cl2 solution with 0.1 M n-Bu4NPF6 as the supporting electrolyte. The CV plots of G0DPP–G2DPP are shown in Fig. 3a, and the data is tabulated in Table 1. All the compounds displayed multiple oxidation and reduction processes. Their reductions occurred at the same reduction half-wave potentials (E1/2) of ∼−1.2 and ∼−1.7 V, which are attributed to the reduction of the di(p-chlorophenyl)-DPP moiety.42 The oxidations at E1/2 of ∼1.2 and ∼1.5 V can also be ascribed to the oxidation of the DPP core. Thus, the oxidation wave at the lower potential of 1.15 V for G1DPP and 1.02 for G2DPP can be related to the oxidation of the grafting carbazole dendrons. Moreover, the CV traces remained unchanged in their continual CV scans, proving the high electrochemical stability of these molecules. Subsequently, the HOMOs, LUMOs, and band gaps were deduced from the oxidation onset (Eooxnset) and reduction onset (Eorenset) following the equations described in the footnote of Table 1. Although optical band gaps for all three materials were found to be identical, the electrochemical HOMO and LUMO values and electrochemical band gaps (Eeleg) varied among the molecules. These values are compared in Table 1. G2DPP was found to have the smallest Eeleg value (1.96 eV) and also the lowest-lying LUMO level (−5.29 eV). Upon going from the unsubstituted hexyl chain (G0DPP) to the 1st generation carbazole dendron (G1DPP) and 2nd generation carbazole dendron (G2DPP), the trend of increasing HOMO energy was observed, and thus narrowing of the bandgap. These electrochemical results are similar to the calculated HOMO, LUMO, and ΔE values calculated in the DFT experiment. The presence of the carbazole dendron is innately likely to make the molecules easier to oxidize. The LUMO levels of the compounds were calculated using the difference between the optical band gap (Egopt) and HOMO level obtained from electrochemical measurements and are listed in Table 1. Markedly, the HOMO levels of G1DPP and G2DPP are close to the work function of the ITO/PEDOT:PSS anode (5.20 eV) (Fig. 5a),58 and as an emissive layer, they can be coated on top of the ITO/PEDOT:PSS anode without any assistance from hole-injection materials (HIM) and hole-transporting materials (HTM), giving rise to a simple device architecture.
The hole-transporting ability of G0DPP–G2DPP was also determined using the space charge limited current (SCLC) method based on the current density–voltage (J–V) measurements of a hole-only device (ITO/PEDOT:PSS (35 nm)/G0DPP–G2DPP (100 nm)/MoO3 (10 nm)/Al (100 nm))59 (Fig. 4). The hole mobilities of G0DPP–G2DPP were estimated by combining the Mott–Gurney equation and Frenkel effect60 to be in the range of 2.00 × 10−6 to 3.35 × 10−5 cm2 V−1 s−1 (Table 1). Among them, G2DPP possessed the highest hole mobility of 3.35 × 10−5 cm2 V−1 s−1. This high hole mobility can be beneficial for high-performance OLED devices.61 It has been established that high mobility emitters can widen the recombination zone in the emissive layer and result in a longer device lifetime and lower driving voltages.61,62
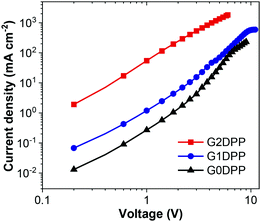 |
| Fig. 4 Current density–voltage (J–V) plots of the hole-only devices. | |
Electroluminescent properties
To evaluate the electroluminescence properties of the newly synthesized di(p-chlorophenyl)-DPP derivatives, OLED devices with the optimized structure of indium tin oxide (ITO)/PEDOT:PSS (35 nm)/EML (35 nm)/TAZ (40 nm)/LiF (0.5 nm)
:
Al (150 nm) were fabricated. The EML was spin-coated from a CHCl3–toluene (1
:
1) solution of G0DPP–G2DPP 3 wt% in mCP with a controllable thickness. The morphology of the casted thin films was studied by atomic force microscopy (AFM), which showed that they all displayed amorphous features with uniform smooth surfaces and root-mean-square roughness (RMS) values of less than 1 nm (Fig. 3c). The surface was observed to have no pinholes and crystalline islands, indicating high-quality nanoscale thin films. To realize the optimal device performance from the EML, TAZ was utilized as an electron-transporting layer (ETL) and hole blocking layer (HBL). As shown in Fig. 5a, the suitable LUMO level of TAZ and its high electron mobility can enable the control and enhancement of the electron–hole recombination zone in the EML,63 whereas the large energy barrier between the HOMOs of the EML and the ETL will confine hole accumulation and exciton recombination close to this interface.64,65 The electroluminescence (EL) spectra, current density–voltage-luminance (J–V–L) plots, current density dependence of the luminance efficiency (LE) and external quantum efficiency (EQE) of the devices are shown in Fig. 5b–e, and the essential EL data is summarized in Table 2.
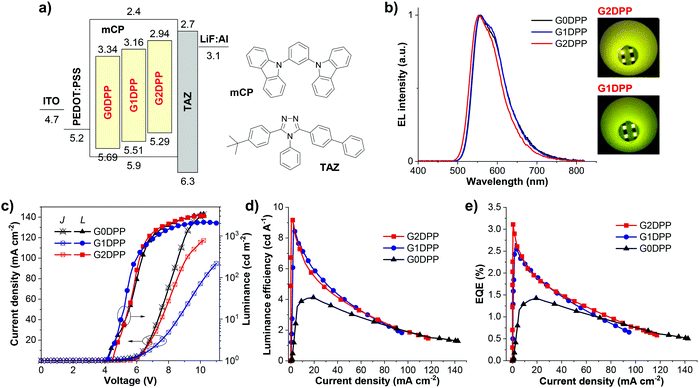 |
| Fig. 5 (a) Device structure and energy levels (relative to the vacuum energy level) of the materials used. (b) Normalized EL spectra (inset: photographs of yellow G1DPP and G2DPP-based OLEDs), (c) current density–voltage–luminance (J–V–L) plots, (d) luminance efficiency–current density (CE–J) plots and (e) external quantum efficiency–current density (EQE–J) plots of the fabricated G0DPP–G3DPP-based OLEDs. | |
Table 2 Electroluminescent data of the fabricated OLED devicesa
Device |
EML |
Vonb (V) |
λELmax (nm) |
FWHM (nm) |
Lmaxc (cd m−2) |
Jmaxd (mA cm−2) |
CEmaxe (cd A−1) |
EQEmaxf (%) |
CIE (x,y) |
ITO/PEDOT:PSS/EML 3 wt% in mCP/TAZ/LiF : Al. Turn-on voltage at 1 cd m−2. Maximum luminance. Current density at maximum luminance. Maximum luminous efficiency. Maximum external quantum efficiency. |
I |
G0DPP |
4.2 |
556 |
88 |
3376 |
142 |
4.13 |
1.42 |
0.48, 0.51 |
II |
G1DPP |
4.2 |
556 |
88 |
2034 |
95 |
8.91 |
2.67 |
0.48, 0.51 |
III |
G2DPP |
4.5 |
553 |
84 |
3060 |
117 |
9.24 |
3.11 |
0.45, 0.53 |
Under an applied voltage, all the devices (I, II, and III) emitted intense yellow EL emission colors peaked at 553–556 nm, as shown in Fig. 5b and the inset. The EL spectra overlapped well with the PL spectra of the EML in a thin film with no emission peaks from the mCP host matrix (404 and 426 nm)66,67 and TAZ layer (380 nm),67 indicating that the EL is purely the emissions from the EML. Remarkably, for each device, the shape and maximum wavelength of its EL spectrum remained unchanged with an increase in applied voltage from 7 V to 11 V (Fig. S2, ESI†). This implies efficient charge injection and recombination in the EML, and the excimer emission and exciplex emissions from the EML/TAZ interface are effectively repressed. All the devices were confirmed to be well-balanced and optimized for both charge transport and energy transfer from the mCP host to the G0DPP–G2DPP dopants and operated stably. Among the three devices, device III having G2DPP as the EML exhibited the best EL performance with a maximum luminance of 3060 cd m−2, maximum LE of 9.24 cd A−1, maximum EQE of 3.11%, and turn-on voltage (Von) of 4.5 V. Device II using G1DPP as the EML showed a slightly lower device EL performance with a maximum luminance of 2034 cd m−2 and maximum LE and EQE values of 8.91 cd A−1 and 2.67%, respectively. The G0DPP-based OLED (device I) exhibited the lowest EL performance with the maximum LE and EQE values of 4.13 cd A−1 and 1.42%, respectively. According to these results, the performance of the devices (Von, LE, and EQE values) decreased in the order of III > II > I, following a similar trend for the hole mobility of the EML materials, which was observed to be G2DPP > G1DPP > G0DPP. It is well-known that a high mobility EML material can widen the recombination zone in the emissive layer and result in a longer device lifetime and lower driving voltages.68 Thus, the outstanding EL performance of the G2DPP emitter can be ascribed to the combination of its high thin film ΦPL, high hole mobility, and suitable HOMO/LUMO levels, ensuring more balanced and improved recharge recombination behavior within the device.69
Conclusions
In conclusion, we reported the design strategy and synthesis of new di(p-chlorophenyl)-DPP derivatives (G0DPP–G2DPP) for use as emissive materials in OLEDs. The molecules were grafted with a series of hole-transporting carbazole dendrons on the DPP core through a hexyl chain linkage to enhance their hole-transporting property and electroluminescent performance in the devices. Owing to the non-conjugated link between the carbazole dendrons and DPP core, they exhibited a slight effect on the optical and electronic properties of the molecules, as expected. Moreover, the carbazole dendron not only assembled in a bulky geometry in the molecules but also enhanced their hole-transporting ability. Because of their weak molecular interactions, these materials exhibited decent thermal stability, good thin film-forming capability, and high photoluminescence quantum yield in the film state. Especially, G2DPP carrying the 2nd generation carbazole dendron as the grating moiety exhibited high hole mobility and realized an excellent electroluminescent performance in a solution-processed double-layered OLED. The device displayed a strong and stable yellow emission color with a brightness of 3060 cd m−2, maximum LE of 9.24 cd A−1, and maximum EQE of 3.11%. It is believed that this work can present a useful methodology to decorate DPP as an efficient light-emitting material with a hole-transporting facet for acquiring simple-structured OLEDs. This work also ascertained that carbazole dendrons can be a worthwhile moiety capable of being exploited for the development of solution-processed hole-transporting emissive compounds.
Author contributions
W. K. performed the synthesis and characterizations of the materials, discussed the data, and prepared the original draft. S. N. and P. C. performed the quantum chemical calculations and analyzed the data. T. C. and T. S. completed the device fabrication and analyzed the data. V. P. and T. M. conceived and supervised the project and review & editing the final draft. All authors approved the final version of the manuscript.
Conflicts of interest
There are no conflicts to declare.
Acknowledgements
This work was financially supported by the National Research Council of Thailand and Suranaree University of Technology (Grant No. 10/9/1-2561). Many thanks also go to the Thailand Research Fund (RTA6080005) and the National Nanotechnology Center, NSTDA, Ministry of Higher Education, Science, Research and Innovation, Thailand, through its program of Research Network National Nanotechnology Center for the supports. We also gratefully acknowledge the graduate fellowship support for W. K. from Udon Thani Rajabhat University (UDRU).
Notes and references
- C. W. Tang and S. A. Vanslyke, Appl. Phys. Lett., 1987, 51, 913–915 CrossRef CAS.
- H. Jia, Natl. Sci. Rev., 2018, 5, 427–431 CrossRef.
- P. Therdkatanyuphong, P. Chasing, C. Kaiyasuan, S. Boonnab, T. Sudyoadsuk and V. Promarak, Adv. Funct. Mater., 2020, 30, 2002481 CrossRef CAS.
- F. So, J. Kido and P. Burrows, MRS Bull., 2008, 33, 663–669 CrossRef CAS.
- U. Mitschke and P. Bäuerle, J. Mater. Chem., 2000, 10, 1471–1507 RSC.
- A. Kumar, W. Lee, T. Lee, J. Jung, S. Yoo and M. H. Lee, J. Mater. Chem. C, 2020, 8, 4253–4263 RSC.
- D. H. Ahn, S. W. Kim, H. Lee, I. J. Ko, D. Karthik, J. Y. Lee and J. H. Kwon, Nat. Photonics, 2019, 13, 540–546 CrossRef CAS.
- L. Yu, Z. Wu, G. Xie, W. Zeng, D. Ma and C. Yang, Chem. Sci., 2018, 9, 1385–1391 RSC.
- X. Liu, B. Yao, Z. Zhang, X. Zhao, B. Zhang, W. Y. Wong, Y. Cheng and Z. Xie, J. Mater. Chem. C, 2016, 4, 5787–5794 RSC.
- P. Funchien, P. Chasing, T. Sudyoadsuk and V. Promarak, Chem. Commun., 2020, 56, 6305–6308 RSC.
- S. M. Russell, A. M. Brewer, D. M. Stoltzfus, J. Saghaei and P. L. Burn, J. Mater. Chem. C, 2019, 7, 4681–4691 RSC.
- M. Kim, S. K. Jeon, S. H. Hwang and J. Y. Lee, Adv. Mater., 2015, 27, 2515–2520 CrossRef CAS PubMed.
- Z. Hao and A. Iqbal, Chem. Soc. Rev., 1997, 26, 203 RSC.
- Q. Liu, S. E. Bottle and P. Sonar, Adv. Mater., 2020, 32, 1903882 CrossRef CAS.
- M. Kaur and D. H. Choi, Chem. Soc. Rev., 2015, 44, 58–77 RSC.
- C. B. Nielsen, M. Turbiez and I. McCulloch, Adv. Mater., 2013, 25, 1859–1880 CrossRef CAS.
- Y. Li, P. Sonar, L. Murphy and W. Hong, Energy Environ. Sci., 2013, 6, 1684–1710 RSC.
- L. Biniek, B. C. Schroeder, C. B. Nielsen and I. McCulloch, J. Mater. Chem., 2012, 22, 14803–14813 RSC.
- B. Walker, J. Liu, C. Kim, G. C. Welch, J. K. Park, J. Lin, P. Zalar, C. M. Proctor, J. H. Seo, G. C. Bazan and T. Q. Nguyen, Energy Environ. Sci., 2013, 6, 952–962 RSC.
- Z. Yi, S. Wang and Y. Liu, Adv. Mater., 2015, 27, 3589–3606 CrossRef CAS PubMed.
- Y. Qu, Y. Wu, Y. Gao, S. Qu, L. Yang and J. Hua, Sens. Actuators, B, 2014, 197, 13–19 CrossRef CAS.
- G. Zhang, S. Bi, L. Song, F. Wang, J. Yu and L. Wang, Dyes Pigm., 2013, 99, 779–786 CrossRef CAS.
- M. Kaur, M. J. Cho and D. H. Choi, Dyes Pigm., 2014, 103, 154–160 CrossRef CAS.
- Y. H. Jeong, C. H. Lee and W. D. Jang, Chem.–Asian J., 2012, 7, 1562–1566 CrossRef PubMed.
- W. Li, L. Wang, H. Tang and D. Cao, Dyes Pigm., 2019, 162, 934–950 CrossRef CAS.
- C. Du, S. Fu, X. Wang, A. C. Sedgwick, W. Zhen, M. Li, X. Li, J. Zhou, Z. Wang, H. Wang and J. L. Sessler, Chem. Sci., 2019, 10, 5699–5704 RSC.
- E. Q. Guo, P. H. Ren, Y. L. Zhang, H. C. Zhang and W. J. Yang, Chem. Commun., 2009, 5859–5861 RSC.
- A. Nowak-Król, M. Grzybowski, J. Romiszewski, M. Drobizhev, G. Wicks, M. Chotkowski, A. Rebane, E. Górecka and D. T. Gryko, Chem. Commun., 2013, 49, 8368–8370 RSC.
- S. Qu and H. Tian, Chem. Commun., 2012, 48, 3039–3051 RSC.
- J. Liu, Y. Zhang, H. Phan, A. Sharenko, P. Moonsin, B. Walker, V. Promarak and T.-Q. Nguyen, Adv. Mater., 2013, 25, 3645–3650 CrossRef CAS.
- Y. Farré, L. Zhang, Y. Pellegrin, A. Planchat, E. Blart, M. Boujtita, L. Hammarström, D. Jacquemin and F. Odobel, J. Phys. Chem. C, 2016, 120, 7923–7940 CrossRef.
- K. Aoshima, M. Ide and A. Saeki, RSC Adv., 2018, 8, 30201–30206 RSC.
- J. C. Bijleveld, A. P. Zoombelt, S. G. J. Mathijssen, M. M. Wienk, M. Turbiez, D. M. de Leeuw and R. A. J. Janssen, J. Am. Chem. Soc., 2009, 131, 16616–16617 CrossRef CAS PubMed.
- C. Zhao, Y. Guo, Y. Zhang, N. Yan, S. You and W. Li, J. Mater. Chem. A, 2019, 7, 10174–10199 RSC.
- K. Gao, S. B. Jo, X. Shi, L. Nian, M. Zhang, Y. Kan, F. Lin, B. Kan, B. Xu, Q. Rong, L. Shui, F. Liu, X. Peng, G. Zhou, Y. Cao and A. K. Y. Jen, Adv. Mater., 2019, 31, 1807842 CrossRef PubMed.
- L. Huo, J. Hou, H. Y. Chen, S. Zhang, Y. Jiang, T. L. Chen and Y. Yang, Macromolecules, 2009, 42, 6564–6571 CrossRef CAS.
- B. Tieke, A. R. Rabindranath, K. Zhang and Y. Zhu, Beilstein J. Org. Chem., 2010, 6, 830–845 CrossRef PubMed.
- O. Fenwick, S. Fusco, T. N. Baig, F. Di Stasio, T. T. Steckler, P. Henriksson, C. Fléchon, M. R. Andersson and F. Cacialli, APL Mater., 2013, 1, 032108 CrossRef.
- M. Sassi, N. Buccheri, M. Rooney, C. Botta, F. Bruni, U. Giovanella, S. Brovelli and L. Beverina, Sci. Rep., 2016, 6, 34096 CrossRef CAS PubMed.
- Y. Zhu, A. R. Rabindranath, T. Beyerlein and B. Tieke, Macromolecules, 2007, 40, 6981–6989 CrossRef CAS.
- G. Wiosna-Salyga, M. Gora, M. Zagorska, P. Toman, B. Luszczynska, J. Pfleger, I. Glowacki, J. Ulanski, J. Mieczkowski and A. Pron, RSC Adv., 2015, 5, 59616–59629 RSC.
- E. D. Głowacki, H. Coskun, M. A. Blood-Forsythe, U. Monkowius, L. Leonat, M. Grzybowski, D. Gryko, M. S. White, A. Aspuru-Guzik and N. S. Sariciftci, Org. Electron., 2014, 15, 3521–3528 CrossRef PubMed.
- A. Van Dijken, J. J. A. M. Bastiaansen, N. M. M. Kiggen, B. M. W. Langeveld, C. Rothe, A. Monkman, I. Bach, P. Stössel and K. Brunner, J. Am. Chem. Soc., 2004, 126, 7718–7727 CrossRef CAS PubMed.
- H. Huang, X. Yang, B. Pan, L. Wang, J. Chen, D. Ma and C. Yang, J. Mater. Chem., 2012, 22, 13223–13230 RSC.
- S. W. Li, C. H. Yu, C. L. Ko, T. Chatterjee, W. Y. Hung and K. T. Wong, ACS Appl. Mater. Interfaces, 2018, 10, 12930–12936 CrossRef CAS PubMed.
- M. Godumala, S. Choi, M. J. Cho and D. H. Choi, J. Mater. Chem. C, 2019, 7, 2172–2198 RSC.
- D. Xia, B. Wang, B. Chen, S. Wang, B. Zhang, J. Ding, L. Wang, X. Jing and F. Wang, Angew. Chem., Int. Ed., 2014, 53, 1048–1052 CrossRef CAS PubMed.
- T. Keawin, N. Prachumrak, S. Namuangruk, S. Pansay, N. Kungwan, S. Maensiri, S. Jungsuttiwong, T. Sudyoadsuk and V. Promarak, RSC Adv., 2015, 5, 73481–73489 RSC.
- J. You, G. Li and Z. Wang, Polymer, 2012, 53, 5116–5123 CrossRef CAS.
- P. Moonsin, N. Prachumrak, R. Rattanawan, T. Keawin, S. Jungsuttiwong, T. Sudyoadsuk and V. Promarak, Chem. Commun., 2012, 48, 3382–3384 RSC.
- N. Prachumrak, S. Pansay, S. Namuangruk, T. Kaewin, S. Jungsuttiwong, T. Sudyoadsuk and V. Promarak, Eur. J. Org. Chem., 2013, 6619–6628 CrossRef CAS.
- P. Moonsin, N. Prachumrak, S. Namuangruk, S. Jungsuttiwong, T. Keawin, T. Sudyoadsuk and V. Promarak, Chem. Commun., 2013, 49, 6388–6390 RSC.
- T. Sudyoadsuk, P. Moonsin, N. Prachumrak, S. Namuangruk, S. Jungsuttiwong, T. Keawin and V. Promarak, Polym. Chem., 2014, 5, 3982–3993 RSC.
- N. Prachumrak, S. Pojanasopa, S. Namuangruk, T. Kaewin, S. Jungsuttiwong, T. Sudyoadsuk and V. Promarak, ACS Appl. Mater. Interfaces, 2013, 5, 8694–8703 CrossRef CAS PubMed.
- X. Li, J. Wang, R. Mason, X. R. Bu and J. Harrison, Tetrahedron, 2002, 58, 3747–3753 CrossRef CAS.
- M. J. Frisch, G. W. Trucks, H. B. Schlegel, G. E. Scuseria, M. A. Robb, J. R. Cheeseman, G. Scalmani, V. Barone, G. A. Petersson, H. Nakatsuji, X. Li, M. Caricato, A. Marenich, J. Bloino, B. G. Janesko, R. Gomperts, B. Mennucci, H. P. Hratchian, J. V. Ortiz, A. F. Izmaylov, J. L. Sonnenberg, D. Williams-Young, F. Ding, F. Lipparini, F. Egidi, J. Goings, B. Peng, A. Petrone, T. Henderson, D. Ranasinghe, V. G. Zakrzewski, J. Gao, N. Rega, G. Zheng, W. Liang, M. Hada, M. Ehara, K. Toyota, R. Fukuda, J. Hasegawa, M. Ishida, T. Nakajima, Y. Honda, O. Kitao, H. Nakai, T. Vreven, K. Throssell, J. A. Montgomery, Jr., J. E. Peralta, F. Ogliaro, M. Bearpark, J. J. Heyd, E. Brothers, K. N. Kudin, V. N. Staroverov, T. Keith, R. Kobayashi, J. Normand, K. Raghavachari, A. Rendell, J. C. Burant, S. S. Iyengar, J. Tomasi, M. Cossi, J. M. Millam, M. Klene, C. Adamo, R. Cammi, J. W. Ochterski, R. L. Martin, K. Morokuma, O. Farkas, J. B. Foresman and D. J. Fox, Gaussian 09, Revision A.02, Gaussian, Inc., Wallin, 2016 Search PubMed.
- K. Ji, Y. Xue and Z. Cui, Opt. Mater., 2015, 47, 180–184 CrossRef CAS.
- J. Wei, D. Bai and L. Yang, PLoS One, 2015, 10, e0133725 CrossRef PubMed.
- H. Li, L. Duan, D. Zhang and Y. Qiu, J. Phys. Chem. C, 2014, 118, 9990–9995 CrossRef CAS.
- C. Katagiri, T. Yoshida, M. S. White, C. Yumusak, N. S. Sariciftci and K. I. Nakayama, AIP Adv., 2018, 8, 105001 CrossRef.
- S. W. Culligan, A. C.-A. Chen, J. U. Wallace, K. P. Klubek, C. W. Tang and S. H. Chen, Adv. Funct. Mater., 2006, 16, 1481–1487 CrossRef CAS.
- J. H. Jou, S. Kumar, M. Singh, Y. H. Chen, C. C. Chen and M. T. Lee, Molecules, 2015, 20, 13005–13030 CrossRef CAS PubMed.
- P. J. Jesuraj, H. Hafeez, D. H. Kim, J. C. Lee, W. H. Lee, D. K. Choi, C. H. Kim, M. Song, C. S. Kim and S. Y. Ryu, J. Phys. Chem. C, 2018, 122, 2951–2958 CrossRef CAS.
- Z. B. Wang, M. G. Helander, Z. W. Liu, M. T. Greiner, J. Qiu and Z. H. Lu, Appl. Phys. Lett., 2010, 96, 043303 CrossRef.
- Q. Wang, Q. S. Tian, Y. L. Zhang, X. Tang and L. S. Liao, J. Mater. Chem. C, 2019, 7, 11329–11360 RSC.
- J. G. Jang and H. J. Ji, Adv. Mater. Sci. Eng., 2012, 192731 Search PubMed.
- T. Y. Cheng, J. H. Lee, C. H. Chen, P. H. Chen, P. S. Wang, C. E. Lin, B. Y. Lin, Y. H. Lan, Y. H. Hsieh, J. J. Huang, H. F. Lu, I. Chao, M. kit Leung, T. L. Chiu and C. F. Lin, Sci. Rep., 2019, 9, 3654 CrossRef PubMed.
- S. W. Culligan, A. C. A. Chen, J. U. Wallace, K. P. Klubek, C. W. Tang and S. H. Chen, Adv. Funct. Mater., 2006, 16, 1481–1487 CrossRef CAS.
- S. Wehrmeister, L. Jäger, T. Wehlus, A. F. Rausch, T. C. G. Reusch, T. D. Schmidt and W. Brütting, Phys. Rev. Appl., 2015, 3, 024008 CrossRef CAS.
Footnote |
† Electronic supplementary information (ESI) available. See DOI: 10.1039/d1ra00805f |
|
This journal is © The Royal Society of Chemistry 2021 |