DOI:
10.1039/D1RA00615K
(Paper)
RSC Adv., 2021,
11, 14415-14425
Bio-mineralisation, characterization, and stability of calcium carbonate containing organic matter†
Received
23rd January 2021
, Accepted 7th April 2021
First published on 19th April 2021
Abstract
The composition of organic matter in biogenic calcium carbonate has long been a mystery, and its role has not received sufficient attention. This study is aimed at elucidating the bio-mineralisation and stability of amorphous calcium carbonate (ACC) and vaterite containing organic matter, as induced by Bacillus subtilis. The results showed that the bacteria could induce various structural forms of CaCO3, such as biogenic ACC (BACC) or biogenic vaterite (BV), using the bacterial cells as their template, and the carbonic anhydrase secreted by the bacteria plays an important role in the mineralisation of CaCO3. The effects of Ca2+ concentration on the crystal structure of CaCO3 were ascertained; when the amount of CaCl2 increased from 0.1% (m/v) to 0.8% (m/v), the ACC was transformed to polycrystalline vaterite. The XRD results demonstrated that the ACC and vaterite have good stability in air or deionised water for one year, or even when heated to 200 °C or 300 °C for 2 h. Moreover, the FTIR results indicated that the BACC or BV is rich in organic matter, and the contents of organic matter in biogenic ACC and vaterite are 39.67 wt% and 28.47 wt%, respectively. The results of bio-mimetic mineralisation experiments suggest that the protein secreted by bacterial metabolism may be inclined to inhibit the formation of calcite, while polysaccharide may be inclined to promote the formation of vaterite. Our findings advance our knowledge of the CaCO3 family and are valuable for future research into organic-CaCO3 complexes.
1 Introduction
CaCO3 comes from a variety of sources, and the mineralisation of CaCO3 induced by microbes is an important source that cannot be ignored. These biogenic CaCO3 crystals are normally nanometric, of diverse crystal types, wrapped in rich organic matter, and complex elemental compositions, differing from non-biogenic carbonates.1–6 Calcium carbonate deposition is a common phenomenon in seawater, fresh water, soil, and other environments. Calcium carbonate generates seven structural forms: calcite, monohydrocalcite, ikaite, aragonite, vaterite, hemihydrate calcium carbonate, and amorphous calcium carbonate (ACC), among which calcite is the most stable structural form at normal temperature and pressure.7–12 The process and mechanism of calcite biomineralisation have been researched deeply, but the research on the process and mechanisms of ACC and vaterite biomineralisation continues to be lacking.
Carbonic anhydrase (CA) was first found in human erythrocytes and is widely present in plants, animals, and microbes. CA is capable of catalyzing the reversible hydration reaction, CO2 + H2O
H+ + HCO3−, of atmospheric and self-generated CO2.13 CA is currently recognised as being involved in carbonate synthesis.13–15 Although bio-induce mineralisation of CaCO3 is related to urease secreted by bacteria, but it requires the addition of urea as its substrate.16–19 A variety of microbes, such as eukaryotic algae,20,21 fungi,22,23 and bacteria3,12,24 can induce CaCO3 mineralisation, however, the morphology and crystal structure of CaCO3 induced by different microbes are quite different. Even the morphology and crystal structure of CaCO3 induced by the same microbe are also quite different under different culturing conditions. ACC is normally a precursor of crystalline calcium carbonates that plays a key role in biomineralisation and polymorph evolution.25 Where, the regulatory mechanism affecting the specific type of CaCO3 (especially metastable ACC and vaterite) is far from clear in the case of microbial participation and further research into the directional induction mechanism of biogenic CaCO3 is needed. Recent research has established that CaCO3 precipitation induced by microbe contains organic components,26,27 while the role of organic matter in the formation and structural stability (especially thermal stability) of biogenic mineral is rarely reported. Biogenic CaCO3 has broad application prospects in biotechnology, civil engineering, and palaeontology.10 Because the formation and precipitation of CaCO3 lead to the deposition of atmospheric CO2, or reduces CO2 emissions in water or soil, it plays a positive role in reducing atmospheric CO2 concentrations and retarding the greenhouse effect.28–30 In addition, biogenic CaCO3 has good adsorption properties for heavy metals,5 and compared with abiotic calcium carbonate, it shows greater potential.31 Our purpose is to elucidate the nucleation patterns and crystallisation processes involved and determine the composition and structure of biogenic CaCO3. In addition, we hope to identify factors controlling biogenic CaCO3 morphology, and discover how crystallisation parameters influence particle morphology in bacterial systems containing different concentrations of Ca2+ ions. The research is believed to be beneficial to the comprehensive exploitation and utilisation of the different types of biogenic CaCO3.
Bacillus subtilis, as a popular strain used in microbial fertilisers, has been developed rapidly and widely in agricultural production across China.5,32,33 During the process of bacterial culture, a large amount of ACC and vaterite precipitation was observed, which showed obvious biological effects. Although there are already some research reports on bacteria-induced carbonate, further study is still needed outlining the ACC and vaterite mineralisation micro-process induced by agricultural fertiliser strain. Here, the bacterial induction of ACC and vaterite mineralisation and its structural characteristics were researched, which will increase the understanding of biogenic calcium carbonate polymorphisms.
2 Materials and methods
2.1 Strain
Experimental strain: B. subtilis (GenBank accession number: KT343639) is China's official certified microbial fertiliser strain.5,32 The strain is adapted to growth in neutral and weakly alkaline conditions. The bacterial colony is opaque, milk-white and yellowish, with a rough, folded surface and irregular edges; the bacteria are seen in short rod form, measuring about (0.6 to 0.8) μm × (1.2 to 1.6) μm.
2.2 The bacterial culture and related indicators for CaCO3 bio-mineralisation
The B. subtilis was inoculated with two loops into 200 mL LB liquid medium (tryptone 1% (m/v), yeast extract 0.5% (m/v), NaCl 1% (m/v), 6.5 ≤ pH ≤ 7.5), shaking-cultured at 30 °C and 180 rpm for about 10 h to prepare bacterial seed liquid [(7.75 ± 1.19) × 107 cfu mL−1]. We prepared 100 mL of the modified LB medium (containing CaCl2 0.2 g, the CaCl2 was added to the medium after separate sterilization) in a 250 mL Erlenmeyer flask to establish two groups: the experimental group (Group E1) (to which 2 mL bacterial seed liquid was added), and the control group (Group C) (to which 2 mL inactivated bacterial seed liquid was added, the bacterial seed liquid was inactivated at 115 °C for 20 min). At the same time, we prepared 100 mL of the LB medium in 250 mL Erlenmeyer flasks to form the E2 group (to which 2 mL bacterial seed liquid was added) as the control group without CaCl2. Each group contained three replicates. These groups were then cultivated in a constant temperature oscillation incubator at 30 °C and 180 rpm for 0–14 days. The OD600nm value of the culture solution was measured daily, then centrifuged at 8000 rpm for 15 min at 4 °C. The supernatant was used for the determination of HCO3− concentration,34 Ca2+ concentration (atomic absorption spectrophotometer, AAS, AA-6300C, Shimadzu), pH (pH meter, SevenEasy S20), and CA activity.34,35 We weighed the dry mass of precipitate from groups E1 and E2 after shaking-culturing for 7 days.
2.3 The role of CA in biogenic CaCO3 mineralisation
Acetazolamide (AZ) is widely used as a CA inhibitor.36,37 To clarify the role of CA in CaCO3 mineralisation, different concentrations (0, 1.0, and 1.5 mg mL−1) of AZ experimental groups were established and cultured at 30 °C and 180 rpm for 7 days. The centrifugal supernatant Ca2+ concentration was measured by AAS. Ignoring the Ca2+ utilised by the strain, most of the Ca2+ would be converted to CaCO3, and then the Ca2+ biomineralisation efficiency was calculated by use of eqn (1):38 |
 | (1) |
where C1 and C2 are the initial, and end (after shaking-culturing for 7 days) concentrations of Ca2+ (mg L−1), respectively.
2.4 The effect of Ca2+ concentrations on biogenic CaCO3 mineralisation and its stability analysis
To explore the effect of Ca2+ concentration on biogenic CaCO3 mineralisation, we added different amounts of CaCl2 (0, 0.1, 0.2, 0.4, and 0.8 g) to 100 mL LB liquid culture medium, each group was dosed with 2 mL bacterial seed liquid, each group was cultured at 180 rpm for 7 days at 30 °C. The culture medium was centrifuged at 8000 rpm for 15 min after measuring the OD600nm values and the number of bacterial cells. The supernatant Ca2+ concentration was determined by AAS, and the Ca2+ biomineralisation efficiency was calculated by use of eqn (1). We collected the precipitate, then added 5% HCl to observe whether there were any bubbles visible, preliminarily to determine whether there was any CaCO3 present, or not. The sample was dropped on a clean cover glass and then observed by field emission scanning electron microscopy and energy dispersive spectrometry (FESEM-EDS, Zeiss Supra55) to analyse its morphology and elemental composition. Then, we determined the mineral structure by X-ray diffraction (XRD-526, Olympus, USA, using Co as the cathode) and transmission electron microscope-selected area electron diffraction (TEM-SAED, JEOL JEM-2000FX II). At the same time, the change in mineral structure was detected after the minerals were preserved at room temperature or shaken in deionised water for one year. The extraction method of organic matter in the CaCO3 refers to Lv et al.39. Analysis of organic matter was undertaken by Fourier transform infrared spectrophotometer (FTIR, Nexus670, Thermo Nicolet), and the content of organic matter in biogenic CaCO3 was investigated by TGA (Perkin-Elmer, USA). The elemental and organic molecules of biogenic CaCO3 were determined by X-ray photoelectron spectroscopy (XPS, ESCALAB Xi+).
To assess the stability of the structure of CaCO3, the precipitates were heated for 2 hours in a high-temperature resistance furnace at 200 °C and 300 °C, and then the changes to the mineral phase structure before and after heating were detected by XRD.
2.5 Bio-mimetic mineralisation of CaCO3
The bio-mimetic mineralisation experiments were conducted to explore the effect of organic matter on the formation of biogenic CaCO3. Salt solution A consisted of 0.2 mol L−1 NaHCO3 and 0.2 mol L−1 CaCl2. The pH of solution A was adjusted to 3.0 using 0.5 mol L−1 HCl, and then it was filtered through a 0.22 μm filter. Solution B is a series of 5 mL solution with different organic components, which are bacterial extracellular protein, extracellular polysaccharide (EPS), fermentation supernatant, glucose, sucrose, or bovine serum albumin. The B. subtilis was cultured in LB liquid medium at 30 °C and 180 rpm for 3 days. The methods for extraction of bacterial crude extracellular protein and EPS were based on those of Sánchez et al.40 and Du et al.,41 respectively. The culture solution was centrifuged at 8000 rpm for 10 min and the supernatant was filtered through a 0.22 μm filter to remove cells. Solution B was added to 15 mL of solution A to form a series of mineralisation systems. The control group was set with 15 mL solution A and 5 mL distilled water. The mineralisation experimental device referred to that used in Lian et al.3 All the experiments were run in triplicate. The precipitation products were collected by centrifugation (8000 rpm, 15 min) after 14 days and dried at 55 °C for XRD analysis.
3 Results and discussion
3.1 Bacterial culture and related indicators of CaCO3 bio-mineralisation
B. subtilis grew rapidly in the LB medium, and on the third day, the OD600nm value had reached 1.30 (Fig. 1a). The rapid growth of the bacterium lead to the change of the pH value in the bacterial culture: the maximum pH value was 8.77 on the third day in the experimental group (added 2 mL bacterial seed liquid), which increased by 2 compared with the initial value (p < 0.01). However, the pH value in the control group (added 2 mL inactivated bacterial seed liquid) remained at 6.8 (its original value, Fig. 1a). It can be concluded that the pH increase is due to the ammonia released by the bacterial growth to degrade the proteins in the mediu.42 The HCO3− concentration of the experimental group first increased up to a maximum (5.4 × 10−4 mol L−1) at the second day, and then fell to 2.38 × 10−4 mol L−1 at the fifth day, whereas the HCO3− concentration in the control group remained quasi-constant (Fig. 1b). The Ca2+ concentration in the experimental group remained almost unchanged in the first two days and continuously decreased in the following 3 days: the Ca2+ concentration decreased to about 240 mg L−1 (p < 0.01), while the concentration of Ca2+ in the control group was unchanged (Fig. 1c). The E1 group (added 2 mL bacterial seed liquid) had obvious precipitation, and bubbled after adding 5% HCl (Fig. S1a†), which suggested that the precipitation in the E1 group contained a lot of CaCO3. While there was no precipitation in the CK group (added 2 mL inactivated bacterial seed liquid) as shown in Fig. S1b.† The dry mass of the precipitation was also significantly higher than that of the E2 group without CaCl2 (p < 0.01), and we found that the dry mass of the precipitate significantly decreased after adding 5% HCl (Fig. 1d). To sum up, we can deduce that the bacteria can induce the formation of CaCO3, and Ca2+ concentration decreased owing to the formation of CaCO3 by precipitation.
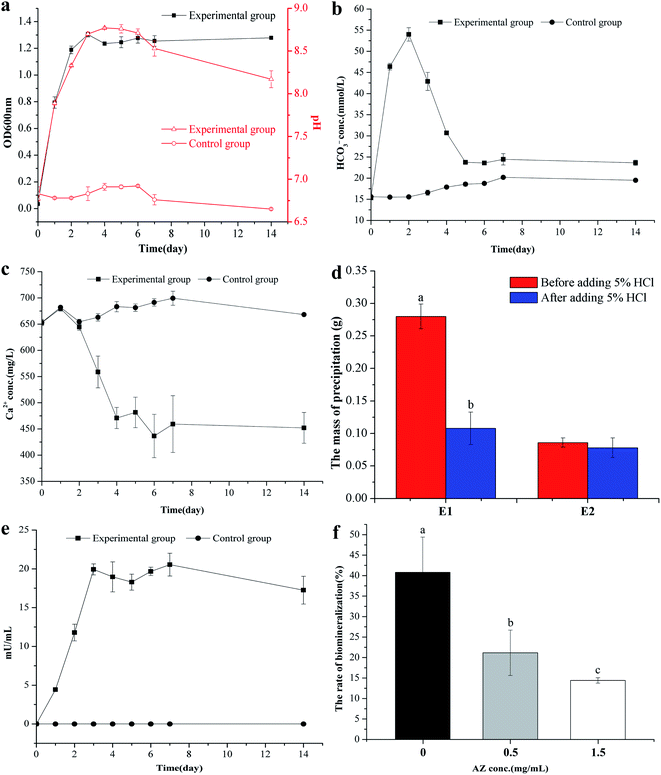 |
| Fig. 1 The dynamic changes of physiological and chemical indexes in the process of calcium carbonate biomineralisation. (a) Change in OD600nm and pH with time. (b) Change in HCO3− concentration with time. (c) Change in Ca2+ concentration with time. (d) The precipitated mass of E1 and E2 group (E1 group: with 0.2% (m/v) CaCl2, E2 group: without CaCl2) (bars with different letters indicate statistically significant differences, t-test, p < 0.01). (e) Change in CA activity with time. (f) The biomineralisation efficiency of Ca2+ after adding CA inhibitors (AZ) (bars with different letters represent statistically significant differences, one-way ANOVA, Duncan's multiple range test, p < 0.05). Data represent mean ± standard deviation (s.d.) of three independent experiments. | |
By measuring the activity of CA, we found that the CA activity in the experimental group increased over time and reached a maximum on the third day, thereafter, it remained almost constant (Fig. 1e), however, there was no microbial metabolism in the control group, so the activity of CA was not detected. For the role of CA on the formation of CaCO3, Fig. 1f showed that the biomineralisation efficiency of Ca2+ decreased after adding CA inhibitor (AZ), and the biomineralisation efficiency of Ca2+ decreased with the increase of AZ concentration, which suggested that bacterial CA secretion is essential for the formation of CaCO3.
3.2 The effect of Ca2+ concentration on CaCO3 bio-mineralisation
The above research demonstrates that B. subtilis can induce biogenic CaCO3 formation in an environment containing Ca2+, but the concentration of Ca2+ in the environment is quite different, which may affect CaCO3 bio-mineralisation. To clarify the mechanism of biogenic CaCO3 mineralisation, experimental groups with different added amounts of CaCl2 [0, 0.1, 0.2, 0.4, and 0.8% (m/v)] were established to identify the differences in CaCO3 bio-mineralisation in bacterial systems containing different concentrations of Ca2+. Fig. 2a showed that the Ca2+ mineralisation rate decreased with increasing Ca2+ increasing. However, the amount of mineralisation increased with the increase of Ca2+ concentration. The mineralisation reached a maximum (45.96 ± 6.95 mg) when the added amount of CaCl2 was 0.8% (m/v). The OD600nm gradually decreased with the increase in amount of CaCl2, and the number of bacterial cell decreased from (9.52 ± 0.86) × 107 cfu mL−1 to (6.22 ± 0.93) × 107 cfu mL−1, which was due to excessive Ca2+ inhibiting bacterial growth (Fig. 2b). 5% HCl was used to test the sediment in each group, and the results showed that the group without added CaCl2 was free of bubbles, while groups with 0.1%, 0.2%, and 0.4% (m/v) CaCl2 produced a few bubbles. Groups with 0.8% (m/v) CaCl2 bubbled copiously, indicating the presence of different amounts of biogenic CaCO3 at different doses of CaCl2 (Fig. 2c). In addition, the XRD result shows that, with the increase of CaCl2 dosing, the CaCO3 induced by this strain can gradually be transformed from ACC to vaterite (ICDD pdf no. 72-0506) (Fig. 2d). To sum up, the morphism and structure of biogenic CaCO3 will be affected by different Ca2+ concentration.
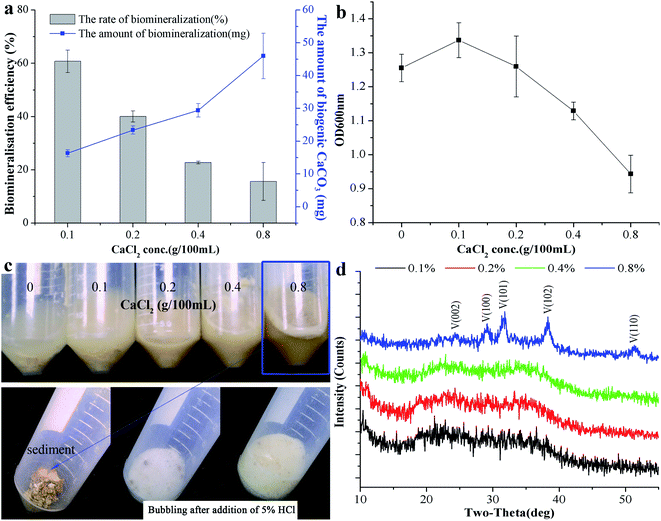 |
| Fig. 2 The effect of different Ca2+ concentrations on CaCO3 mineralisation. (a) The biomineralisation efficiency and amount of biogenic CaCO3. (b) The OD600nm of the bacterial culture at different amounts of added CaCl2 after 7 days. (c) The precipitated reaction phenomenon after adding 5% HCl. (d) XRD results for the precipitate of different doses of CaCl2. V: vaterite, ICDD pdf no. 72-0506. Data represent mean ± s.d. of three independent experiments. | |
3.3 The morphology and mineralogical composition of biogenic CaCO3
FESEM and TEM were utilized to observe the sediment after culturing for 7 days. We could observe some micro and nano-sized CaCO3 with different morphologies, such as irregular, scaly aggregates, dumbbell-shaped, and spherical: their surfaces were mainly porous or corner-incomplete (Fig. 3a and b). From the enlarged FESEM image (Fig. 3b), the quasi-spherical CaCO3 particles were rough-edged and were mainly formed by stacking nano-particles. The diameters of CaCO3 particles or their aggregates were between 100 nm and 14 μm from 10 different recorded SEM and TEM visual images. The CaCO3 in the culture system containing 0.2% (m/v) CaCl2 revealed poorly resolved diffuse rings under TEM-SAED (Fig. 3c), and the XRD analysis shows no obvious diffraction peak (Fig. 2d), which demonstrates that the CaCO3 was formed as ACC.25,43,44 However, the CaCO3 in the group containing 0.8% (m/v) CaCl2 shows evident diffraction rings under TEM-SAED (Fig. 3d), which suggests that the CaCO3 had formed a polycrystalline ring structure with a greater degree of crystallisation;43 and the XRD result showed that the CaCO3 was mainly composed of vaterite (Fig. 2d).
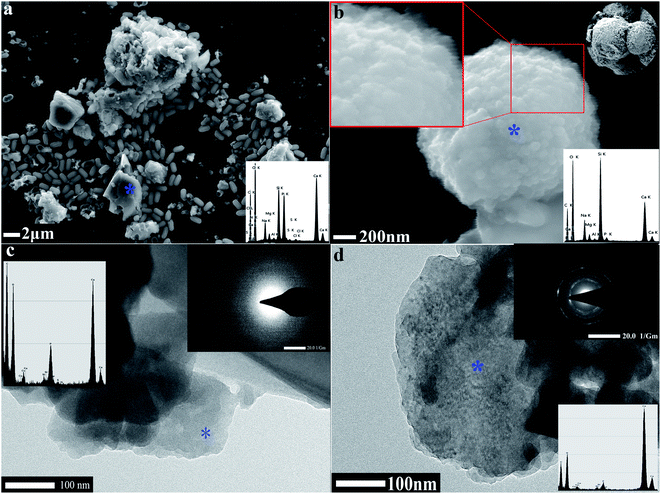 |
| Fig. 3 Morphology and mineralogical analyses of CaCO3. (a) FESEM-EDS result of CaCO3 in 0.2% (m/v) CaCl2 group. (b) FESEM-EDS result of CaCO3 in 0.8% (m/v) CaCl2 group. (c) TEM-SAED result of CaCO3 in 0.2% (m/v) CaCl2 group. (d) TEM-SAED result of CaCO3 in 0.8% (m/v) CaCl2 group. | |
3.4 The stability analysis of biogenic ACC and vaterite structures
ACC serves as a precursor in the biomineralisation of almost all types of biogenic calcium carbonate.45 Generally, ACC and vaterite will transform into a stable phase of calcite under certain conditions.39,46,47 However, the XRD patterns of biogenic ACC or vaterite have no change in either air or deionised water over one year (Fig. 4a), or even heated to 200 °C or 300 °C for 2 h (Fig. 4b and c). Obviously, the biogenic ACC here is very different with the reported laboratory-produced ACC that needs to be stored in a desiccator below 10 °C for avoiding crystallization48 or stable for half year at room temperature.25 In addition, FTIR results (Fig. 4d) of the ACC and vaterite extracts suggest that there are some absorption peaks corresponding to diverse organic functional groups (including: –OH, –CH3, –CH2, –CO/NH, C
O, –COOH, and –NH).39,49–51 Thus, we can infer that there are some organic compounds combined with the ACC and vaterite, forming an organic–inorganic complex structure. The TGA results show that, the calcium carbonate induced by the bacteria undergoes a mass-loss stage caused by organic matter combustion (within the range of 209–579 °C), compared to AR (Analytical Reagent)-CaCO3 (purchased from Shanghai Sangon Biotech Co., Ltd) and limestone (provided by the Institute of Geochemistry, Chinese Academy of Sciences). And the contents of organic matter in biogenic ACC and vaterite are 39.67 wt% and 28.47 wt%, respectively (Fig. 5a and b), which are markedly higher than that in biogenic calcium carbonate (1.87 wt% and 6.82 wt%) induced by other strains.26,27
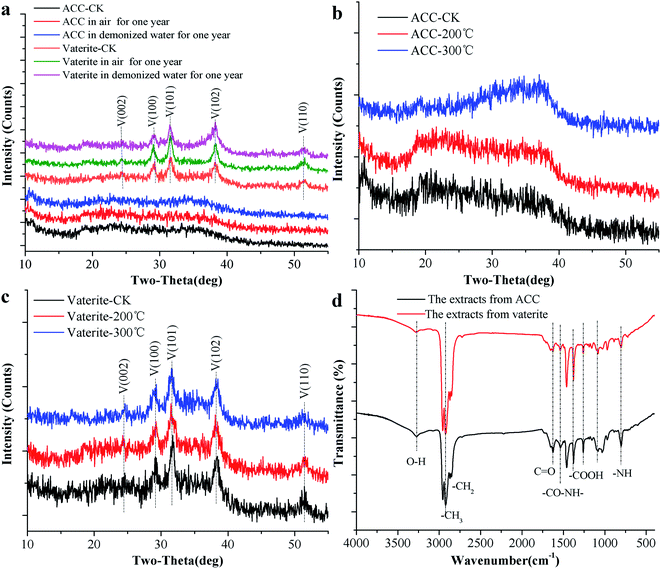 |
| Fig. 4 The stability analysis of ACC and vaterite. (a) XRD patterns of ACC and vaterite under different conditions. (b) FTIR spectra of extracts from ACC and vaterite. (c) XRD patterns of ACC at different temperatures. (d) XRD patterns of vaterite at different temperatures. | |
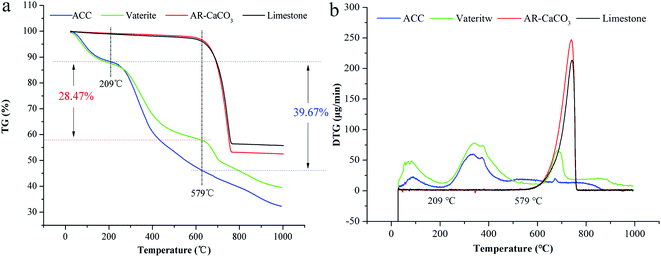 |
| Fig. 5 TGA (a) and DTG (b) results: ACC, vaterite, AR-CaCO3, and limestone. | |
Moreover, the surface elementary compositions of the biogenic vaterite were determined by XPS over the energy range of 0–1200 eV. As showed in Fig. S2a,† the typical XPS survey spectrum shows that the core level peaks are C 1s (284.8 eV), O 1s (531.8 eV), Ca 2p (346.8 eV), and N 1s (399.8 eV). The mass fractions of C, O, Ca, and N were estimated about 62.76%, 27.59%, 5.12%, and 4.53%, respectively. Here, all three elements comprising CaCO3, i.e. Ca, C, and O, as well as N which should come from organic matter were observed. The high-resolution XPS Ca 2p core-level spectrum has two peaks, identified as Ca 2p3/2 (347.1 eV) and Ca 2p1/2 (350.6 eV) (Fig. S2b†), and is in agreement with the reported value of vaterite.52 To clarify the organic molecules involved in vaterite mineralisation, high resolution scans of C 1s and O 1s were deconvoluted, and the corresponding functional groups were recognized as C–(C/H), C–OH, C–O–C, C
O and O–C
O. Fig. S2c and d† depict the presence of three C (1s) and two O (1s). To clarify the organic molecules involved in vaterite mineralisation, high resolution scans of C 1s, and O 1s were deconvoluted, and the corresponding functional groups were recognized. The C 1s peak was resolved into three component peaks, i.e., the peak at 284.6 eV (58.89%) can correspond to the C–(C/H) from lipids or amino acid side chains, the peak at 285.9 eV (24.76%) to C–OH or C–O–C from alcohol, ether, or phenol, the peak at 288.3 eV (16.35%) to CaCO3, respectively. The O 1s peak at 531.5 eV (82.19%) can be assigned to CaCO3, or C
O and O–C
O from carboxylic acid, carboxylate, carbonyl, or amide.26,53,54 The second O 1s peak at 533.2 eV (17.81%) is associated with C–OH or C–O–C from alcohol, acetal, and hemiacetal.53,55 These organic matters are enwrapped by ACC and vaterite and can restrain their transformation into stable calcite.2,3,26,39,56 The XRD patterns of products obtained by bio-mimetic mineralisation further confirm our speculation. From the XRD patterns (Fig. S3†), vaterite can be collected in most systems except those containing bovine serum albumin and distilled water. In other words, vaterite can only form in the presence of carbohydrate (crude extracellular protein contains some polysaccharide). Interestingly, the diffraction peaks of calcite harvested in systems with proteins were weaker than those in the control group. The results suggest that protein may be inclined to inhibit the formation of calcite, while polysaccharide may be inclined to promote the formation of vaterite. Hence, extracellular polysaccharide and protein secreted by bacterial metabolism maintain the stability of vaterite.
3.5 The micro-mechanism underpinning the formation of biogenic CaCO3
The bio-mineralisation site of CaCO3 synthesis has always a focus among researchers in this discipline.3,39,57 We found that the bacteria were inserted in the CaCO3 particles and left obvious moulages on their surface (Fig. 6a and b), which suggested that the extracellular structural substances of the bacteria play an important role in CaCO3 formation or growth. Meanwhile, we observed many calcified bacterial cells with some irregular micro-grooves on the bacterial surfaces by FESEM, and the EDS results showed the presence of CaCO3 on the bacterial surfaces (Fig. 6c). Moreover, the TEM image showed that the CaCO3 not only forms in the regions surrounding the bacterial cells, but also forms directly on the surface of the bacterial cells (Fig. 6d). It is possible that Ca2+ was attracted by the negatively charged groups on the surface of bacteria especially at each of the two ends of the bacterium, then CO32− was attracted through the cationic bridge58–60 thus leading to the formation of various types of CaCO3 (dumbbell type being the most common). CA was to catalyze the hydration of carbon dioxide, and this process released carbonate and bicarbonate ions that not only increased pH but also elevated carbonate supersaturation.42 Therefore, we can infer that the bacterial exterior may be a good natural mineralising site, and the alkaline environment formed by bacteria can enhance the mineralisation on the bacterial surface. A spherical form of CaCO3 can be grown by the transformation of dumbbell type CaCO3 using a bacterial cell as the nucleation site.61,62 In summary, the mechanism and process of CaCO3 mineralisation are illustrated in Fig. 7.
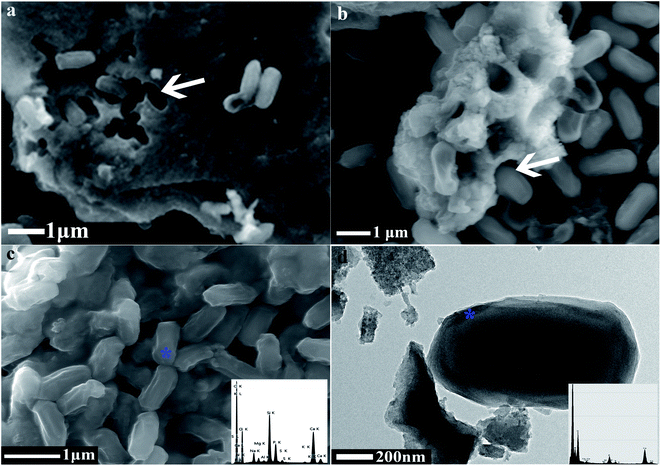 |
| Fig. 6 The moulage caused by the bacteria on the CaCO3 surface and calcified bacterial cells. (a and b) The FESEM images of moulage left by the bacteria and embedded bacterial cells on the surface of CaCO3. (c) The FESEM-EDS of calcified bacterial cells. (d) The TEM-EDS of calcified bacterial cells. | |
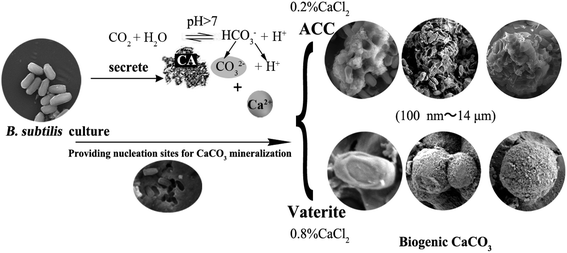 |
| Fig. 7 The possible schematic mechanisms of CaCO3 bio-mineralisation. | |
4 Conclusions
This research found that B. subtilis could induce ACC or polycrystalline vaterite formation according to different Ca2+ concentration, which has long-term water stability and thermal stability. The stability of ACC and vaterite is closely related to the protein and extracellular polysaccharide secreted by the bacterium. The protein may be inclined to inhibit the formation of calcite, and the polysaccharide may be inclined to promote the formation of vaterite. The final morphology and structure of carbonate depends on the interaction between the protein and extracellular polysaccharide. Meanwhile, the bacterial cells, alkaline metabolites, and CA are important to the CaCO3 bio-mineralisation process. The discovery of ACC and vaterite, their formation pathways, and the determination of their organic–inorganic structures broadens our knowledge of the mechanism of bio-mineralisation and provides reference for the exploitation and application of biogenic CaCO3.
Conflicts of interest
There are no con conflicts to declare.
Acknowledgements
This work was supported by the National Key Technology R&D Program of China (Grant no. 2019YFC180510303), the Jiangsu Provincial Marine Science and Technology Innovation Special Project (Grant no. HY2019-3), the National Natural Science Foundation of China (Grant no. 41867032) and the Jiangxi Provincial Natural Science Foundation (Grant no. 20202BABL215028).
References
- C. Cao, J. Jiang, H. Sun, Y. Huang, F. Tao and B. Lian, Carbonate mineral formation under the influence of limestone-colonizing Actinobacteria: morphology and polymorphism, Front. Microbiol., 2016, 7, 366 Search PubMed.
- M. Kawano and J. Hwang, Roles of microbial acidic polysaccharides in precipitation rate and polymorph of calcium carbonate minerals, Appl. Clay Sci., 2011, 51, 484–490 CrossRef CAS.
- B. Lian, Q. Hu, J. Chen, J. Ji and H. H. Teng, Carbonate biomineralization induced by soil bacterium Bacillus megaterium, Geochim. Cosmochim. Acta, 2006, 70, 5522–5535 CrossRef CAS.
- M. Obst, B. Wehrli and M. Dittrich, CaCO3 nucleation by cyanobacteria: laboratory evidence for a passive, surface-induced mechanism, Geobiology, 2009, 7, 324–347 CrossRef CAS PubMed.
- R. Liu, Y. Guan, L. Chen and B. Lian, Adsorption and desorption characteristics of Cd2+ and Pb2+ by micro and nano-sized biogenic CaCO3, Front. Microbiol., 2018, 9, 41 CrossRef PubMed.
- F. Héctor, G. Mónica, A. Bonifacio, I. Aldo and A. Marisela, Novel method to achieve crystallinity of calcite by Bacillus subtilis in coupled and non-coupled calcium-carbon sources, AMB Express, 2020, 10, 1–20 CrossRef PubMed.
- F. Giuseppe, F. Simona, R. Michela, N. D. A. Branka and K. Damir, Evidence of structural variability among synthetic and biogenic vaterite, Chem. Commun., 2014, 50, 15370–15373 RSC.
- G. Zhou, Y. Guan, Q. Yao and S. Fu, Biomimetic mineralization of prismatic calcite mesocrystals: Relevance to biomineralization, Chem. Geol., 2010, 279, 63–72 CrossRef CAS.
- E. Bauerlein, Biomineralization of unicellular organisms: An unusual membrane biochemistry for the production of inorganic nano- and microstructures, Angew. Chem., Int. Ed., 2003, 42, 614–641 CrossRef CAS PubMed.
- N. K. Dhami, M. S. Reddy and A. Mukherjee, Biomineralization of calcium carbonates and their engineered applications: a review, Front. Microbiol., 2013, 4, 314 Search PubMed.
- Z. Zou, W. J. E. M. Habraken, G. Matveeva, A. C. S. Jensen, L. Bertinetti, M. A. Hood, C. Sun, P. U. P. A. Gilbert, I. Polishchuk, B. Pokroy, J. Mahamid, Y. Politi, S. Weiner, P. Werner, S. Bette, R. Dinnebier, U. Kolb, E. Zolotoyabko and P. Fratzl, A hydrated crystalline calcium carbonate phase: Calcium carbonate hemihydrate, Science, 2019, 363, 396–400 CrossRef CAS PubMed.
- R. Rautela and S. Rawat, Analysis and optimization of process parameters for in vitro biomineralization of CaCO3 by Klebsiella pneumoniae, isolated from a stalactite from the sahastradhara cave, RSC Adv., 2020, 10, 8470–8479 RSC.
- B. C. Tripp, K. Smith and J. G. Ferry, Carbonic anhydrase: new insights for an ancient enzyme, J. Biol. Chem., 2001, 276, 48615–48618 CrossRef CAS PubMed.
- I. M. Power, A. L. Harrison and G. M. Dipple, Accelerating mineral carbonation using carbonic anhydrase, Environ. Sci. Technol., 2016, 50, 2610–2618 CrossRef CAS PubMed.
- Q. Sun and B. Lian, The different roles of Aspergillus nidulans carbonic anhydrases in wollastonite weathering accompanied by carbonation, Geochim. Cosmochim. Acta, 2019, 244, 437–450 CrossRef CAS.
- S. Stocks-Fischer, J. K. Galinat and S. S. Bang, Microbiological precipitation of CaCO3, Soil Biol. Biochem., 1999, 31, 1563–1571 CrossRef CAS.
- F. Hammes, N. Boon, J. de Villiers, W. Verstraete and S. D. Siciliano, Strain-specific ureolytic microbial calcium carbonate precipitation, Appl. Environ. Microbiol., 2003, 69, 4901–4909 CrossRef CAS PubMed.
- T. O. Okyay, H. N. Nguyen, S. L. Castro and D. F. Rodrigues, CO2 sequestration by ureolytic microbial consortia through microbially-induced calcite precipitation, Sci. Total Environ., 2016, 572, 671–680 CrossRef CAS PubMed.
- M. Tepe, E. Arslan, T. Koralay and N. M. Doan, Precipitation and characterization of CaCO3 of Bacillus amyloliquefaciens U17 strain producing urease and carbonic anhydrase, Turk. J. Biol., 2019, 43, 198–208 CrossRef CAS PubMed.
- B. D. Lee, W. A. Apel and M. R. Walton, Calcium carbonate formation by Synechococcus sp. strain PCC 8806 and Synechococcus sp. strain PCC 8807, Bioresour. Technol., 2006, 97, 2427–2434 CrossRef CAS PubMed.
- R. Ramanan, K. Kannan, A. Deshkar, R. Yadav and T. Chakrabarti, Enhanced algal CO2 sequestration through calcite deposition by Chlorella sp. and Spirulina platensis in a mini-raceway pond, Bioresour. Technol., 2010, 101, 2616–2622 CrossRef CAS PubMed.
- W. Hou, B. Lian and X. Zhang, CO2 mineralization induced by fungal nitrate assimilation, Bioresour. Technol., 2011, 102, 1562–1566 CrossRef CAS PubMed.
- S. Masaphy, L. Zabari, J. Pastrana and S. Dultz, Role of fungal mycelium in the formation of carbonate concretions in growing media—an investigation by SEM and synchrotron-based X-ray tomographic microscopy, Geomicrobiol. J., 2009, 26, 442–450 CrossRef CAS.
- A. C. Mitchell and F. G. Ferris, The influence of Bacillus pasteurii on the nucleation and growth of calcium carbonate, Geomicrobiol. J., 2006, 23, 213–226 CrossRef CAS.
- N. T. Enyedi, J. Makk, L. Kótai, B. Berényi, S. Klébert, Z. Sebestyén, Z. Molnár, A. K. Borsodi, S. Leél-Őssy, A. Demény and P. Németh, Cave bacteria-induced amorphous calcium carbonate formation, Sci. Rep., 2020, 10, 8696 CrossRef CAS PubMed.
- H. Li, Q. Yao, F. Wang, Y. Huang, S. Fu and G. Zhou, Insights into the formation mechanism of vaterite mediated by a deep-sea bacterium Shewanella piezotolerans WP3, Geochim. Cosmochim. Acta, 2019, 256, 35–48 CrossRef CAS.
- Y. Y. Wang, Q. Z. Yao, H. Li, G. T. Zhou and Y. M. Sheng, Formation of vaterite mesocrystals in biomineral-like structures and implication for biomineralization, Cryst. Growth Des., 2015, 15, 1714–1725 CrossRef CAS.
- A. C. Mitchell, K. Dideriksen, L. H. Spangler, A. B. Cunningham and R. Gerlach, Microbially enhanced carbon capture and storage by mineral-trapping and solubility-trapping, Environ. Sci. Technol., 2010, 44, 5270–5276 CrossRef CAS PubMed.
- C. Dupraz, R. P. Reid, O. Braissant, A. W. Decho, R. S. Norman and P. T. Visscher, Processes of carbonate precipitation in modern microbial mats, Earth-Sci. Rev., 2009, 96, 141–162 CrossRef CAS.
- A. J. Phillips, E. Lauchnor, J. Eldring, R. Esposito, A. C. Mitchell, R. Gerlach, A. B. Cunningham and L. H. Spangler, Potential CO2 leakage reduction through biofilm-induced calcium carbonate precipitation, Environ. Sci. Technol., 2013, 47, 142–149 CrossRef CAS PubMed.
- R. Liu and B. Lian, Immobilisation of Cd(II) on biogenic and abiotic calcium carbonate, J. Hazard. Mater., 2019, 378, 120707 CrossRef CAS PubMed.
- Y. S. Kim, S. H. Kim, S. H. Cho and G. J. Lee, Effects of two formulation types of a microbial fertilizer containing Bacillus Subtilis on rowth of creeping bentgrass, J. Int. Med. Res., 2014, 43, 3–8 Search PubMed.
- F. O. T. S. Pérez Barrera and K. O. T. S. Akti, Bacterial strain and composition used to accelerate composting and as a fertilizer, EP1721966B1, 2009-10-28 Search PubMed.
- J. Han, B. Lian and H. Ling, Induction of calcium carbonate by Bacillus cereus, Geomicrobiol. J., 2013, 30, 682–689 CrossRef CAS.
- Y. Pocker and J. T. Stone, The catalytic versatility of erythrocyte carbonic anhydrase. III. kinetic studies of the enzyme-catalyzed hydrolysis of p-nitrophenyl acetate, Biochemistry, 1967, 6, 668–678 CrossRef CAS PubMed.
- W. Li, P. Zhou, L. Jia, L. Yu, X. Li and M. Zhu, Limestone dissolution induced by fungal mycelia, acidic materials, and carbonic anhydrase from fungi, Mycopathologia, 2009, 167, 37–46 CrossRef CAS PubMed.
- A. Moya, S. Tambutté, A. Bertucci, E. Tambutté, S. Lotto, D. Vullo, C. T. Supuran, D. Allemand and D. Zoccola, Carbonic anhydrase in the scleractinian coral Stylophora pistillata characterization, localization, and role in biomineralization, J. Biol. Chem., 2008, 283, 25475–25484 CrossRef CAS PubMed.
- X. Liu, W. Wang, M. Wang and P. Wang, Experimental study of CO2 mineralization in Ca2+-rich aqueous solutions using tributylamine as an enhancing medium, Energy Fuels, 2014, 28, 2047–2053 CrossRef CAS.
- J. J. Lv, F. Ma, F. C. Li, C. H. Zhang and J. N. Chen, Vaterite induced by Lysinibacillus sp. GW-2 strain and its stability, J. Struct. Biol., 2017, 200, 97–105 CrossRef CAS PubMed.
- B. Sánchez, S. Chaignepain, J. Schmitter and M. C. Urdaci, A method for the identification of proteins secreted by lactic acid bacteria grown in complex media, FEMS Microbiol. Lett., 2009, 295, 226–229 CrossRef PubMed.
- Y. Du, X. Zhou and B. Lian, The extracellular secretion of Bacillus mucilaginosus and its capability of releasing potassium from potassium-bearing minerals, Earth Sci. Front., 2008, 15, 107–111 CAS.
- Z. Han, J. Wang, H. Zhao, M. E. Tucker, Y. Zhao, G. Wu, J. Zhou, J. Yin, H. Zhang, X. Zhang and H. Yan, Mechanism of biomineralization induced by Bacillus subtilis J2 and characteristics of the biominerals, Minerals, 2019, 9, 218 CrossRef CAS.
- C. Rodriguez-Navarro, K. Kudłacz, Ö. Cizer and E. Ruiz-Agudo, Formation of amorphous calcium carbonate and its transformation into mesostructured calcite, Crystengcomm, 2015, 17, 58–72 RSC.
- A. Martignier, M. Pacton, M. Filella, J. M. Jaquet, F. Barja, K. Pollok, F. Langenhorst, S. Lavigne, P. Guagliardo and M. R. Kilburn, Intracellular amorphous carbonates uncover a new biomineralization process in eukaryotes, Geobiology, 2017, 15, 240–253 CrossRef CAS PubMed.
- R. S. Rani and M. Saharay, Molecular dynamics simulation of protein-mediated biomineralization of amorphous calcium carbonate, RSC Adv., 2019, 9, 1653–1663 RSC.
- A. Vecht and T. G. Ireland, The role of vaterite and aragonite in the formation of pseudo-biogenic carbonate structures: implications for Martian exobiology, Geochim. Cosmochim. Acta, 2000, 64, 2719–2725 CrossRef CAS.
- R. S. K. Lam, J. M. Charnock, A. Lennie and F. C. Meldrum, Synthesis-dependant structural variations in amorphous calcium carbonate, Crystengcomm, 2007, 9, 1226–1236 RSC.
- F. Konrad, F. Gallien, D. E. Gerard and M. Dietzel, Transformation of amorphous calcium carbonate in air, Cryst. Growth Des., 2016, 16, 6310–6317 CrossRef CAS.
- Y. Cheng, F. Zhou, S. Li and Z. Chen, Removal of mixed contaminants crystal violet and heavy metal ions by immobilized stains as the functional biomaterial, RSC Adv., 2016, 6, 67858–67865 RSC.
- C. Quintelas, Z. Rocha, B. Silva, B. Fonseca, H. Figueiredo and T. Tavares, Removal of Cd(II), Cr(VI), Fe(III) and Ni(II) from aqueous solutions by an E. coli biofilm supported on kaolin, Chem. Eng. J., 2009, 149, 319–324 CrossRef CAS.
- M. E. Argun and S. Dursun, A new approach to modification of natural adsorbent for heavy metal adsorption, Bioresour. Technol., 2008, 99, 2516–2527 CrossRef CAS PubMed.
- M. Ni and B. D. Ratner, Differentiation of calcium carbonate
polymorphs by surface analysis techniques-an XPS and TOF-SIMS study, Surf. Interface Anal., 2008, 40, 1356–1361 CrossRef CAS PubMed.
- S. Yuan, M. Sun, G. Sheng, Y. Li, W. Li, R. Yao and H. Yu, Identification of key constituents and structure of the extracellular polymeric substances excreted by Bacillus megaterium TF10 for their flocculation capacity, Environ. Sci. Technol., 2011, 45, 1152–1157 CrossRef CAS PubMed.
- C. Yin, F. Meng and G. Chen, Spectroscopic characterization of extracellular polymeric substances from a mixed culture dominated by ammonia-oxidizing bacteria, Water Res., 2015, 68, 740–749 CrossRef CAS PubMed.
- X. Sun, S. Wang, X. Zhang, J. Paul Chen, X. Li, B. Gao and Y. Ma, Spectroscopic study of Zn2+ and Co2+ binding to extracellular polymeric substances (EPS) from aerobic granules, J. Colloid Interface Sci., 2009, 335, 11–17 CrossRef CAS PubMed.
- C. Zhang, F. Li and J. Lv, Morphology and formation mechanism in precipitation of calcite induced by Curvibacter lanceolatus strain HJ-1, J. Cryst. Growth, 2017, 478, 96–101 CrossRef CAS.
- D. Ren, Q. Feng and X. Bourrat, Effects of additives and templates on calcium carbonate mineralization in vitro, Micron, 2011, 42, 228–245 CrossRef CAS PubMed.
- G. Aloisi, A. Gloter, M. Krüger, K. Wallmann, F. Guyot and P. Zuddas, Nucleation of calcium carbonate on bacterial nanoglobules, Geology, 2006, 34, 1017–1020 CrossRef CAS.
- S. Schultze-Lam, D. Fortin, B. S. Davis and T. J. Beveridge, Mineralization of bacterial surfaces, Chem. Geol., 1996, 132, 171–181 CrossRef CAS.
- Y. Politi, T. Arad, E. Klein, S. Weiner and L. Addadi, Sea urchin spine calcite forms via a transient amorphous calcium carbonate phase, Science, 2004, 306, 1161–1164 CrossRef CAS PubMed.
- L. Gránásy, T. Pusztai, G. Tegze, J. A. Warren and J. F. Douglas, Growth and form of spherulites, Phys. Rev. E: Stat., Nonlinear, Soft Matter Phys., 2005, 72, 11605 CrossRef PubMed.
- F. Li, H. Ma, N. Su, J. Wang, M. Liu, J. Wang and F. Teng, Clostridium sp. controlled morphology of Mg-bearing calcite and its implication for possible mechanism, Geol. J. China Univ., 2011, 17, 13–20 CAS.
Footnote |
† Electronic supplementary information (ESI) available. See DOI: 10.1039/d1ra00615k |
|
This journal is © The Royal Society of Chemistry 2021 |