DOI:
10.1039/D0RA10821A
(Paper)
RSC Adv., 2021,
11, 9608-9620
Moving-bed biofilm reactor combined with three-dimensional electrochemical pretreatment (MBBR–3DE) for 2,4-D herbicide treatment: application for real wastewater, improvement of biodegradability†
Received
24th December 2020
, Accepted 16th February 2021
First published on 4th March 2021
Abstract
2,4-Dichlorophenoxyacetic acid (2,4-D) is a herbicide that is considered as a carcinogenic and highly toxic contaminant, and due to its biological and chemical stability, its degradation is very difficult. Therefore, this study aimed to investigate a hybrid system's efficiency of three-dimensional electrochemical (3DE) process and a moving bed biofilm reactor (MBBR) in removing 2,4-D herbicides from aqueous solutions. In this experimental study, the electrochemical degradation of 2,4-D herbicide in a 3DE process with a G/β-PbO2 anode was first investigated as a pretreatment process. Then, in the post-treatment stage, MBBR with continuous flow was used. The amount of aeration in the MBBR reactor was 4 L min−1, and the amount of dissolved oxygen (DO) was in the range of 3–5 mg L−1. The effect of various parameters such as hydraulic retention time (HRT) and filling ratio were investigated. The amount of sewage injection was set between 0.001–0.004 L min−1. Routine microbiological biochemical tests were used to detect bacteria. BOD5/COD, COD/TOC, AOS, and COS ratio parameters were used to determine the biodegradability of 2.4-D due to the effluent of the 3DE process. The results showed that with increasing current density, decreasing pH, decreasing herbicide concentration and increasing electrolysis time, the herbicide degradation efficiency increased by 3DE pretreatment process. Based on the results of MBBR post-treatment process efficiency, with increasing HRT and filling ratio, the herbicide removal efficiency increased. According to the results, the highest removal efficiencies of 2,4-D and COD herbicides were obtained during HRT of 24 h, and the filling ratio of 70% were 97.33% and 88.95%, respectively. The consortium of 2,4-D degrading bacteria identified in this study included E. coli, Enterobacter spp., Bacillus spp., Alcaligenes spp., Proteus spp., Acinetobacter spp., Pseudomonas spp., Arthrobacter, and Brevundimonas vesicularis. In the MBBR biological process, the reaction kinetics followed the Grau second-order model (R2 = 0.98). In general, the results showed that the combined process of 3DE with G/β-PbO2 anode and MBBR biological process has relatively high efficiency in 2,4-D herbicide degradation and can be used as a suitable complementary treatment method in wastewater containing non-degradable compounds such as phenoxy herbicides, e.g., 2,4-D should be used.
1. Introduction
One group of the poisons used to control weeds in agriculture and rangelands is phenoxy herbicides and the most widely used is 2,4-dichlorophenoxyacetic acid (2,4-D). These compounds are used to control broadleaf weeds in corn, small grains, sorghum, rice and sugarcane fields, and pasture and rangeland and grass plants.1,2 Phenoxy herbicides are highly soluble in water; therefore, they are easily leached and found in surface and groundwater sources;3 so that in many areas, they can be determined even in drinking water.4 These herbicides have low biodegradability and toxicity (carcinogenic, teratogenic, mutagenic, adverse effects on the endocrine system, hepatotoxicity, and renal toxicity).5,6 Many attempts for the elimination of this herbicide using techniques, e.g., adsorption,4,7 biological degradation8 and advances oxidation process (AOPs)9 have been made, and different disadvantages have been observed for their application, some of which are listed as follows: high cost, incomplete pollutant removal, production of toxic by-products, need to add chemical compounds, sludge production and need for more treatment.10 Over the past few years, electrochemical technologies have been associated with significant progress in solving water and wastewater problems. Because these methods are environmentally friendly, have high versatility and efficiency, are responsive in terms of operation (automation) and safety, and are also cost-effective.11
In this treatment process, dissolving the metal and entering them into the solution occurs when the anode electrode and rising hydrogen gas occur at the cathode. Metal ions produced from the anode can be hydrolyzed to form a set of active intermediates that destabilize particles and contaminants in wastewater.12 The three-dimensional electrochemical (3DE) process has many similarities with its two-dimensional (2D) counterparts regarding electrode and treatment processes. But the presence of a third electrode in the 3DE process is their difference. In other words, in this process, a third electrode, also known as a particle or bed electrode, is attached to the system, which consists of granular or particle materials filled between two opposite electrodes.13 Removal of the target contaminant using a 3DE process is much higher due to its specific surface area and therefore much more electrons and active sites compared to the 2D types.14 The reason for this is that during the process, these particles are easily polarized due to the application of external electrical force, and as a result, a large number of charged microelectrodes has appeared in solution, so that one surface of each of them acts as an anode and the other surface acts as cathode. This improves the treatment efficiency.15 Although oxidation methods such as 3DE technology have good performance in removing degradable contaminants such as toxins, but the production of by-products and their release into the environment is one of the serious limitations of this method. Therefore, the use of complementary methods such as biological methods to eliminate this defect is mandatory. For this purpose, after the 3DE process, the moving bed biofilm reactor (MBBR) biological method was integrated. Since biological methods are not able to remove chlorinated aromatic compounds in a reasonable time, the use of pretreatment method reduces this limitation to some extent. In designing the MBBR process, the main idea has been to match the best tools and capabilities of the sludge and biofilter (BF) processes and apply them in parallel and eliminate each's disadvantages. In this process, media components with a high effective surface area are used for biofilm growth of microorganisms, and tanks are used for biomass growth. Unlike most biofilm reactors, the MBBR uses the entire volume of the tank to grow the biomass. In this system, biomass growth takes place on media. The media move easily in the aqueous environment and remain inside the reactor using the metal net embedded in the outlet.16,17 Therefore, this study aimed to investigate the combined system's efficiency consisting of 3DE and a MBBR in removing 2,4-D herbicides from aqueous solutions.
2. Materials and methods
2.1. Chemicals and reagents
Some of the chemicals, including 2,4-dichlorophenoxyacetic acid (the chemical formula of C8H6Cl2O3 and the purity of 99%) with a molecular weight of 221.04 g mol−1, lead nitrate (Pb(NO3)2, >99% purity) and nitric acid (HNO3, 95% purity) and sodium sulfate (Na2SO4, 99% purity) were provided by Sigma Aldrich (St. Louis, MO, USA). Other chemicals, i.e., CaCl2·2H2O, MgSO4·7H2O, KI, MnCl2·4H2O, FeCl3·6H2O, CuSO4·5H2O, H3BO3, CoCl2·6H2O, ZnSO4·7H2O, Na2MnO4·2H2O, EDTA, CaCl2·H2O and MgSO4·7H2O were bought from Merck Co, Germany. Sodium hydroxide (NaOH) and sulfuric acid (H2SO4) were also supplied by Merck Co (Darmstadt, Germany). The regulation of solution pH was carried out using 0.01 N H2SO4 and 0.01 N NaOH.
2.2. Study of combined processes
This experimental study was designed to evaluate the performance of combined system consisting of the three-dimensional electrochemical (3DE) process and moving bed biofilm reactor (MBBR) for the removal of the herbicide 2,4-dichlorophenoxy acetic acid (2,4-D) solution.
2.3. Set-up of electrochemical three-dimensional (3D) process
In this part of the present study, the electrochemical degradation of 2,4-D herbicide was investigated in a Plexiglas reactor with a useful volume of 250 cm3. The schematic of the reactor is shown in Fig. 1. In this reactor, lead dioxide electrode coated on graphite substrate (G/β-PbO2) with dimensions of 7.0 × 5.8 × 0.5 cm was utilized as the anode, and stainless steel 316 (SS316) with dimensions of 7.0 × 5.8 × 0.1 cm were used as cathodes; the distance between them was 4 cm and they were parallelly employed. To provide the third dimension of the 3D electrode, granular activated carbon (GAC, particle electrode) with a constant concentration of 32 g L−1 was used. The reactor's characteristics were such that GAC floated between the two electrodes due to mixing. Sodium sulfate (Na2SO4) was used as a supporting electrolyte to provide the desired potential. A direct current power supply with a voltage range of 0–40 V and an electric current 0–5 A was used to provide power for the degradation of 2,4-D herbicide.
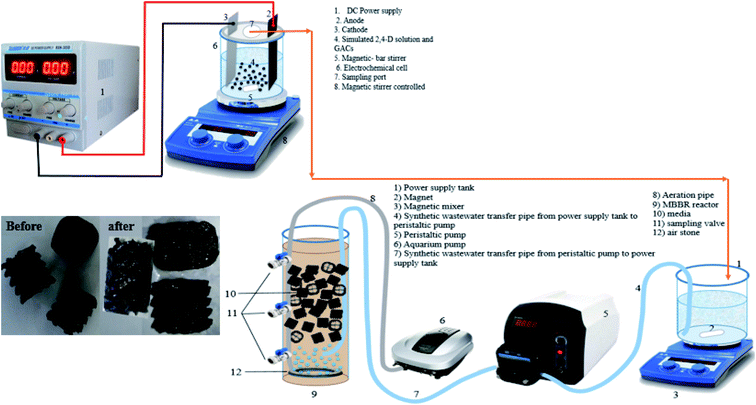 |
| Fig. 1 Schematic of reactor used in this study. | |
2.4. Preparation of the G/β-PbO2 electrode
Preparing the G/β-PbO2 anode was accomplished by electrochemically deposition of PbO2 layers on graphite sheets (dimensions of 7.0 × 5.8 × 0.5 cm). Its procedure was concisely as follows: the polished graphite sheets were sonicated in 40% NaOH solution for 15 min, and after that, they were sonicated in a 1
:
1 (v/v) HNO3/H2SO4 mixture for another 15 min. Electrochemical deposition was performed as a constant current in a simple cell containing 0.5 mol L−1 lead nitrate (Pb(NO3)2) and 0.1 mol L−1 nitric acid (MEGATEK, MP-3005, China). The prepared graphite sheet was utilized as the anode, and the cathode was stainless-steel sheet with the same dimensions. The process of deposition of PbO2 film on the anode was accomplished under a constant current of 7.5 mA cm−2 for 180 min and at room temperature.18
2.5. Set-up of MBBR system and wastewater characteristics
In this part of the study, the MBBR was made of Plexiglas (with a cylindrical shape) with a useful volume of about 2.0 L (diameter of 10.0 cm and height of 30.0 cm) (Fig. 1). In this study, media 2H-BCN 017 KL was used, the characteristics of which are presented in Table S1.† The Sonic 9908 aquarium pump was used to supply the air required to supply the oxygen needed for biological reactions and to mix the media in the MBBR. Aeration was performed at a rate of 4 L min−1. The aeration rate was adjusted so that gentle mixing was achieved inside the reactor during aeration, and the amount of dissolved oxygen was in the range of 3–5 mg L−1. To take the samples from the reactor, 3 valves were installed in the reactor at a distance of 10.0 cm from each other. During the operation period, the bioreactor's temperature was adjusted in the range of 21–25 °C. A peristaltic pump was used to regulate the inlet flow to the MBBR reactor (0.001–0004 L min−1).
For the production of synthetic wastewater, glucose as a source of carbon and potassium dihydrogen phosphate (KH2PO4) as a source of phosphorus and ammonium chloride (NH4Cl) as a source of nitrogen was used to adjust the C/N/P ratio of 1
:
5
:
100 with COD equal to 500.0 mg L−1. The micronutrient characteristics used in this study to feed the bacteria were presented in Table S2.† Seeding operation was used to activate the reactors. For seeding the MBBR reactor, the return sludge of Hamadan city wastewater treatment plant was used. In this study, about 30% of the bioreactor volume was filled with return sludge (TSS of sludge = 6200 mg L−1), and the rest of the reactor volume was filled with water up to a useful volume. During the activation time, the organic load of the synthetic wastewater with a C/N/P ratio of 100
:
5
:
1 was gradually added to grow microorganisms and the formation of biofilms on the media. Initially, the reactor was started in a sequencing batch mood, during which their aeration was stopped every 10.0 h and the sludge was allowed to settle. After about 20 min, 300.0 mL of the reactor effluent was discharged through the valves installed in the reactor and the synthetic wastewater containing glucose was re-injected into the system. This process was performed over three weeks and then the system was operated in continuous conditions. After 25 days of biological activity, a suitable biofilm layer was formed on the media.19–21 It should be noted that the hydraulic retention time (HRT) under continuous conditions was considered 24 h. After the growth of biofilm and compatibility of microorganisms with 2,4-D herbicide, different concentrations (1.0, 5.0, 10.0, 20.0, 30.0, 35.0, 40.0, 50.0 and 60.0 volume percentage of synthetic wastewater) solution containing 2,4-D herbicide releasing from the 3DE process was added to synthetic wastewater for adaption of microorganisms to the 2,4-D herbicide. The biological process was set up with a filling ratio of 70% and a retention time of 24 h, and at different retention times (8, 12 and 24 h), the reactor efficiency was measured for this filling ratio (70%). Then experiments were performed with a filling ratio of 50% and finally, with a 30% ratio for different retention times (8, 12 and 24 h). To discharge the effluent from the biological reactor, the topest outlet valve of the MBBR biological reactor was continuously opened.19,20
2.6. Kinetics of the process
The biological models is applicable to clarify the relationship between the variables (wastewater concentration (S0), output concentration (Se), volume and HRT), and to simplify the assessment of the empirical designs and results.22 Besides, these models can also control and predict the treatment's performance and can be employed for optimizing the units assembled on a laboratory scale.
In this study, Grau first order and second-order models were used to investigate the reaction kinetics. Thus, by changing the amount of COD of the inlet solution and the change in retention time (5, 10, 15, 21, and 24 h) in optimal process conditions (filling ratio of 70% and 60% 2,4-D herbicide solution), changes in organic loading were applied. The results of the data obtained from the initial COD values of 2,4-D and the outlet of each of the desired retention times were placed in the equations of each model (eqn (1) and (2)). It should be noted that all experiments in the above steps were repeated three times to reduce the error and achieve better results.
|
 | (1) |
In the first-order model, by plotting
vs. S, the value of
k1 was obtained from the slope of the drawn line. In the above relation,
S0 and
S are the concentration of inlet and outlet COD in terms of mg L
−1, respectively; HRT is the hydraulic retention time in terms of the day, and
k1 is the first-order kinetic constant in terms of 1 per day.
19,20 |
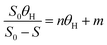 | (2) |
In Grau second-order kinetic,
n is the dimensionless slope;
m is the intercept in terms of the day, and
θH is the hydraulic retention time in terms of days.
19,20
2.7. Analytical methods
ICP-OES (Optima-8300) was used to evaluate the lead leakage in the effluent from the 3DE process (pre-treatment) after degradation of 2,4-D herbicide under optimal test conditions.
Electrochemical analysis of 2,4-D herbicide and electrolysis solution was performed using a cell containing three conventional electrodes including a glassy carbon electrode (8 mm2) as the working electrode, a platinum wire as the counter electrode and a saturated calomel electrode (SCE) was used as the reference electrode. The voltammograms were obtained by cyclic voltammetry (CV) using the Autolab PGSTAT20 potentiostat. Also, liquid chromatography-mass spectrometer (LC-MS) was prepared for conversion chromatographs in the effluent sample. The LC/MS system used in this research was the Shimadzu LCMS 2010 A equipped with a C18 column (2.1 × 100 × 100 mm) and its mobile phase consisted of 60% acetonitrile/40% pure water + 0.01 formic acid.
Besides, to determine the biodegradability of 2,4-D herbicide in the effluent of the three-dimensional electrochemical process (pretreatment), BOD5/COD, COD/TOC ratio, the average oxidation state (AOS) and carbon oxidation state (COS) was obtained under optimal test conditions. Eqn (3) and (4) were used to determine AOS and COS indices.23 All experiments were performed according to standard methods24 and at laboratory temperature.
|
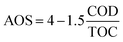 | (3) |
|
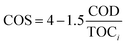 | (4) |
TOC is reported in moles of C per liter, and COD is expressed in mg L
−1 of O
2.
2.8. Identification of herbicide-degrading microorganisms
In this study, to detect bacteria in the MBBR reactor to remove 2,4-D herbicide, culture was first performed in microbial culture media. After than, Gram staining was used to identify the bacteria and finally, routine microbiological biochemical tests (catalase test, oxidase test, OF test, MR–VP test, TSI test, KIA test, Simmons citrate agar, urease test and gelatinase) were used (Table S3†).25 Merck Company provided materials required for initial isolation and identification of isolated bacteria. To normalize the pH of all media, 0.1 N HCl and 0.1 N NaOH were used.
2.9. Process efficiency on real wastewater
After optimizing the 3D electrode process, the real sample of agricultural drainage was prepared in Parsabad Moghan city (Ardabil province, Iran). At first, the typical properties of real wastewater such as electrical conductivity (EC), total dissolved solids (TDS), chemical oxygen demand (COD), total organic carbon (TOC), 2,4-D herbicide concentration and pH were determined. Experiments before and after treatment by the electrochemical process on the real sample were performed three times at normal pH of agricultural drainage water, electrolysis time of 100 min and current density of 9 mA cm−2.
3. Results and discussion
3.1. Morphology of G/β-PbO2 electrode
According to figures related to scanning electron microscopy (SEM), the particles' agglomeration with limited individual particle boundary was approved. The electrodeposited PbO2 microparticles on graphite indicated the crystalline structures β-PbO2 and its tetragonal structure (Fig. 2a and b). Based on the energy-dispersive X-rays (EDX), the oxygen (O) and lead (Pb) were the main elements existed in the β-PbO2. It is also detected that the weight percentages of O and Pb were 20.9% and 79.1%, respectively (Fig. 2c). The X-ray diffraction patterns (XRD) of the PbO2 layer deposited in the graphite interlayers were brought in Fig. 2d; based on this figure, the β-form diffraction peaks of PbO2 have been identified. The tetragonal structure of β-PbO2 could also be confirmed by XRD results. Unlike the orthorhombic α-PbO2, the tetragonal β-PbO2 was allied with good conductivity;26,27 this can amazingly help the electro-oxidation of contaminants in the aqueous solutions using anode studied. According to ICSD card number (89-2805) the foremost diffraction peaks appeared at 2θ of 25.4°, 32.0°, 36.2°, 49.1°, and 62.5° were related to the (110), (101), (200), (211), (220) plane of β-PbO2, respectively.28,29 It is imperative to mention that the existence of β-PbO2 in all samples has been proven. Moreover, the Debye–Scherrer formula's data revealed that the size of β-PbO2 crystals in the G/β-PbO2 electrode was 30.2 nm.
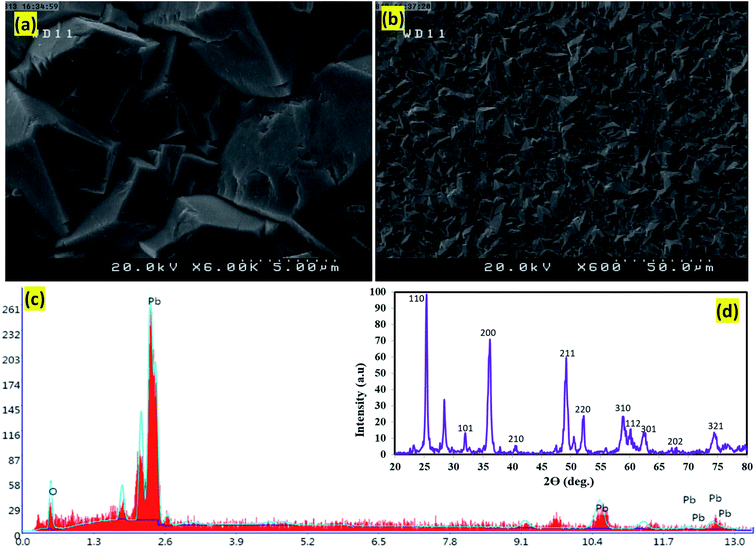 |
| Fig. 2 SEM images of the surface morphology of the β-PbO2 electrodeposited on graphite substrate, (a) magnification image 5 μm and (b) magnification image 50 μm of the β-PbO2 deposited film, (c) EDX spectrum of the β-PbO2 and (d) XRD diffractogram of the β-PbO2 electrodeposited on graphite substrate. | |
3.2. Three-dimensional electrochemical process
3.2.1. Effect of different parameters. Table 1 shows the results for each of the experiments. The highest and lowest 2,4-D removal efficiencies among the 16 experiments designed by Taguchi method are related to experiment No. 4 (92.05%) and experiment No. 13 (32.0%), respectively. Analysis of means (ANOM) values for determining the optimal conditions were presented in Table 2. The maximum values of M (mean) for each factor indicate the optimal state of that factor. According to Fig. 3, the optimal state for the three-dimensional electrochemical (3DE) process was obtained at pH of 5.0 and time of 100 min, initial concentration of 2,4-D herbicide of 100 mg L−1 and current density of 9 mA cm−2, and the highest efficiency in optimal conditions was observed to be 93.5% (Fig. 3).
Table 1 The condition for Taguchi design experiments and the results obtained for each experimenta
Run |
Factors |
R1 (%) |
R2 (%) |
M (%) |
SD |
pH |
Electrolysis time (min) |
2,4-D concentration (mg L−1) |
Current density (mA cm−2) |
R1 = removal for test 1; R2 = removal for test 2; M = mean; SD = standard deviation. |
1 |
3.0 |
30 |
50 |
3 |
55.35 |
55.15 |
55.25 |
0.14 |
2 |
3.0 |
50 |
100 |
5 |
72.31 |
71.15 |
71.73 |
0.82 |
3 |
3.0 |
80 |
150 |
7 |
84.50 |
82.65 |
83.57 |
1.31 |
4 |
3.0 |
100 |
200 |
9 |
91.87 |
92.23 |
92.05 |
0.25 |
5 |
5.0 |
30 |
100 |
7 |
63.14 |
64.05 |
63.59 |
0.64 |
6 |
5.0 |
50 |
50 |
9 |
88.44 |
86.77 |
87.60 |
1.18 |
7 |
5.0 |
80 |
200 |
3 |
72.88 |
72.19 |
72.53 |
0.48 |
8 |
5.0 |
100 |
150 |
5 |
82.65 |
83.95 |
83.30 |
0.92 |
9 |
6.0 |
30 |
150 |
9 |
57.69 |
56.15 |
56.92 |
1.08 |
10 |
6.0 |
50 |
200 |
7 |
56.36 |
55.73 |
56.04 |
0.44 |
11 |
6.0 |
80 |
50 |
5 |
61.53 |
63.75 |
62.64 |
1.57 |
12 |
6.0 |
100 |
100 |
3 |
63.65 |
65.82 |
64.73 |
1.53 |
13 |
10.0 |
30 |
200 |
5 |
31.76 |
32.25 |
32.00 |
0.34 |
14 |
10.0 |
50 |
150 |
3 |
39.19 |
41.80 |
40.49 |
1.84 |
15 |
10.0 |
80 |
100 |
9 |
72.59 |
72.81 |
72.70 |
0.15 |
16 |
10.0 |
100 |
50 |
7 |
67.58 |
67.97 |
67.77 |
0.27 |
Table 2 Results of ANOM analysis for determination of the optimal conditions for 3DE process
Factor |
Level 1 |
Level 2 |
Level 3 |
Level 4 |
M (removal, %) |
pH = 3.0 |
55.25 |
71.73 |
83.57 |
92.05 |
75.65 |
pH = 5.0 |
63.56 |
87.61 |
72.54 |
83.30 |
76.75 |
pH = 7.0 |
56.92 |
56.04 |
62.64 |
64.74 |
60.08 |
pH = 10.0 |
32.01 |
40.49 |
72.70 |
67.77 |
53.24 |
Time = 30 min |
55.25 |
63.59 |
56.92 |
32.01 |
51.94 |
Time = 50 min |
71.73 |
87.61 |
56.04 |
40.49 |
63.96 |
Time = 80 min |
83.57 |
72.54 |
62.64 |
72.70 |
72.86 |
Time = 100 min |
92.05 |
83.30 |
64.74 |
67.77 |
76.96 |
C0 = 50 mg L−1 |
55.25 |
87.61 |
62.64 |
67.77 |
68.31 |
C0 = 100 mg L−1 |
71.73 |
63.59 |
64.74 |
72.70 |
68.19 |
C0 = 150 mg L−1 |
83.57 |
83.30 |
56.92 |
40.49 |
66.07 |
C0 = 200 mg L−1 |
92.05 |
72.54 |
56.04 |
32.01 |
63.15 |
j = 3 mA cm−2 |
55.25 |
72.54 |
64.74 |
40.49 |
58.25 |
j = 5 mA cm−2 |
71.73 |
83.30 |
62.64 |
32.01 |
62.41 |
j = 7 mA cm−2 |
83.57 |
63.59 |
56.04 |
67.77 |
67.74 |
j = 9 mA cm−2 |
92.05 |
87.61 |
56.92 |
72.70 |
77.32 |
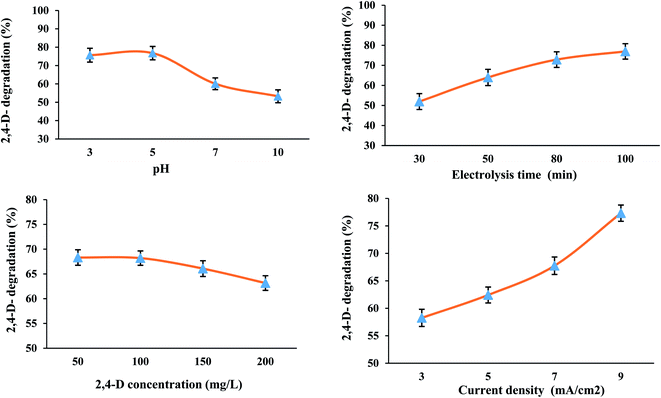 |
| Fig. 3 Effect of parameters different on the degradation of 2,4-D herbicide by 3D electrochemical process. | |
To determine the effect of pH, different pH values from 3.0 to 10.0 were analyzed for the degradation of 2,4-D herbicide by a 3DE process. The results showed that the degradation efficiency of 2,4-D herbicide increased with decreasing pH (Fig. 3); so that the maximum degradation of 2,4-D herbicide was obtained at pH of 3.0, which is consistent with the studies of Tran et al. (2017)30 and Ansari et al. (2018).28 As the pH of the solution decreases, the number of hydroxyl radicals produced increases, which have a higher potential for degradation of pollutants in an acidic environment, and therefore the oxidation of organic matter occurs more rapidly.3 Changes in the inlet current to the reactor are one of the effective parameters in the electrochemical process. To determine the effect of current density, the different current densities in the range of 3–9 mA cm−2 were investigated to degrade 2,4-D herbicide by a 3DE process. The results showed that with increasing current density, the degradation efficiency of the 3DE process also increases (Fig. 3). The reason for the increase in efficiency can be attributed to the production of more hydroxyl radicals by increasing the current density.31 Another parameter affecting the degradation rate of 2,4-D herbicide by the 3DE process is the different concentrations of 2,4-D herbicide. Accordingly, herbicide concentrations in the range of 50–200 mg L−1 were investigated. The results showed that the efficiency of the electrochemical process has an indirect relationship with herbicide concentration. By increasing the concentration of herbicide in the electrochemical process, the degradation efficiency decreases (Fig. 3). The results of other studies have also shown that in the electrochemical process under constant conditions in terms of electric current intensity and reaction time, when the initial concentration of the pollutant increases, its degradation efficiency decreases, which can be interpreted as follows: by keeping constant the retention time and the amount of current intensity, which are the main factors producing hydroxyl radicals in the electrochemical process, a specific and constant amount of hydroxyl radical is produced in the environment that can remove a certain amount of pollutant molecules. At low concentrations of herbicides, hydroxyl radicals are easily able to remove a high percentage of contaminants in the reaction chamber, but as the concentration of contaminants increases, the amount of hydroxyl ions produced is not sufficient for further degradation of the herbicide.32 In the present study, the electrolysis time was in the range of 30–100 min for the degradation of 2,4-D herbicide by the 3DE process. The results showed that the electrolysis time was directly related to the degradation efficiency of 2,4-D herbicide, so that with increasing the electrolysis time, the degradation efficiency of the herbicide also enhanced (Fig. 3). It is in agreement with the results of the study of Ansari et al.28 With increasing electrolysis time, the amount of hydroxyl radical produced is more and, as a result, the degradation efficiency of 2,4-D herbicide by 3DE process increases.
3.3. Determination of biodegradability of 3DE process effluent
In this study, to determine the biodegradability of 2,4-D herbicide in the effluent of the 3DE process (pretreatment), the parameters of BOD5/COD, COD/TOC, average oxidation state (AOS) and carbon oxidation state (COS) were examined under optimal test conditions (pH of 5.0, electrolysis time of 100 min, initial 2,4-D concentration of 100 mg L−1, and current density of 9 mA cm−2). The results showed that the parameters of BOD5/COD, AOS and COS in the effluent of the 3DE process increased from 0.0 to 0.45, from 0.83 to 1.5 and from 0.64 to 3.46, respectively, and the COD/TOC ratio decreased from 2.26 to 1.66 (Table 3), which shows the biodegradability of 2,4-D herbicide by the electrochemical process; obtained results are consistent with the study of Ferrag-Siagh et al.33 In the study of Ferrag-Siagh et al.,33 the electro-Fenton (EF) process as pretreatment for the biological process was used to treat tylosin tartrate, which is a resistant contaminant. In this study, BOD5/COD, COD/TOC and AOS ratio parameters in the effluent were used to determine the pretreatment process's biodegradability. The EF process was investigated. The results showed that the amount of BOD5/COD and AOS parameters in the effluent increased, and the COD/TOC ratio decreased, which indicates the biodegradability of the process; the amount of BOD5/COD and AOS parameters in the effluent of the electro-Fenton process increased from 0.0 to 0.6 and 0.52 to 2.9, respectively, and the COD/TOC ratio decreased from 2.32 to 0.73 (Table 3).
Table 3 The amount of different parameters in determining the biodegradability of 3DE process effluent
Parameter |
COD |
TOC |
COD/TOC |
BOD5/COD |
COS |
AOS |
Inlet |
113.0 ± 1.25 |
50.4 ± 0.83 |
2.26 |
0 |
0.64 |
0.84 |
Outlet |
18.0 ± 0.46 |
10.8 ± 1.46 |
1.66 |
0.45 |
3.46 |
1.50 |
Removal efficiency (%) |
84.0 ± 0.86 |
78.6 ± 1.21 |
— |
— |
— |
— |
Leaching levels of lead ions were evaluated using ICP-OES after the completion of the 2,4-D degradation process using G/β-PbO2 electrode. According to results, after completion of the process, the Pb2+ concentration was observed to be 0.0013 ppm; the observed value was less than WHO guideline (0.01 mg L−1) for drinking water.34
3.4. Biological process
3.4.1. Adaptation of microorganisms with 2,4-D herbicide. In this part of the study, after optimizing the electrochemical process, the MBBR biological reactor was first adapted to 2,4-D herbicide. The diagram for MBBR reactor adaptation and its efficiency in COD removal was presented in Fig. 4. According to Fig. 4, the MBBR reactor to adapt microorganisms to 2,4-D herbicide was first set up for 21 days in batch mode and then continuously until the 30th day, and the results in terms of COD removal was presented in Fig. S1.† As shown in the figure, the rate of COD removal was about 60% for the first 10 days, but after that, it had an increasing trend, so that during 30 days, the efficiency of the biological process in COD removal reached the values higher than 95%. It should be noted that the initial inlet COD to the MBBR reactor was adjusted to 500 mg L−1 during the adaptation period. After that for the continuous system, different concentrations (1%, 5%, 10%, 20%, 30%, 35%, 40%, 50% and 60% by volume of synthetic wastewater) of solution containing herbicide 2,4-D output from the 3DE process was added to synthetic wastewater for adaption of microorganisms to 2,4-D herbicide under continuous operation, the results of which are shown in Fig. 4 and Table 4. It should be noted that during the addition of 2,4-D herbicide from the effluent, the MBBR process was set up with a filling ratio of 70% and a retention time of 24 h. According to Table 4, the amount of COD entering the effluent containing 2,4-D herbicide to the MBBR reactor for different volumetric percentages of synthetic wastewater (1, 5, 10, 20, 30, 35, 40, 50, and 60 volume percentage) were 497.01, 476.26, 455.18, 405.87, 355.74, 305.21, 259.5 and 209.95 mg L−1, respectively. Also, the concentration of 2,4-D herbicide in the inlet effluent to the MBBR reactor for different volumetric percentages of synthetic wastewater (1, 5, 10, 20, 30, 35, 40, 50, and 60 volume percentage) were 0.09, 0.53, 1.15, 2.38, 3.41, 4.23, 5.37, and 6.21 mg L−1, respectively. Moreover, the volume percentages were obtained from the output of the 3DE process presented in Table 4. The results showed that with increasing the volume percentage of effluent containing 2,4-D herbicide, the removal efficiency of 2,4-D and COD decreased so that the lowest removal efficiencies of 2,4-D and COD herbicide (97% and about 90%) were obtained in 60 volume percentage of effluent containing herbicides. It should be noted that the pH of the MBBR process was set in the range of 6.5–8.5.
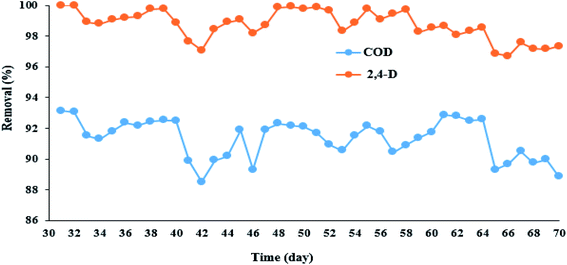 |
| Fig. 4 Efficiency of MBBR biological reactor in removing 2,4-D herbicide and COD during adaptation under continuous reactor operating conditions. | |
Table 4 How to adapt microorganisms to 2,4-D herbicide under continuous operation of MBBR process (COD0 = 500 mg L−1)
Volumetric percentage (%) |
1 |
5 |
10 |
20 |
30 |
40 |
50 |
60 |
HRT (day) |
31–32 |
33–36 |
37–40 |
41–45 |
46–52 |
53–58 |
59–64 |
65–70 |
COD (mg L−1) |
497.01 |
476.26 |
455.18 |
405.87 |
355.74 |
305.21 |
295.50 |
209.95 |
2,4-D (mg L−1) |
0.09 |
0.53 |
1.15 |
2.38 |
3.41 |
4.23 |
5.37 |
6.21 |
3.5. The effect of different parameters on MBBR performance
3.5.1. The effect of hydraulic retention time. The results of evaluating the effect of hydraulic retention time (HRT) on the removal efficiency of 2,4-D herbicide and COD in a media-containing reactor were presented in Fig. 6. The results showed that with decreasing HRT, the efficiency of MBBR process decreased so that the removal efficiencies of 2,4-D herbicide and COD were reduced from 97.33% and 88.95% in HRT of 24 h and filling ratio of 70% to (84.72% and 77.88%) in the HRT of 8 h; it can be concluded that HRT is an effective parameter on the reduction of 2,4-D herbicide and COD, which is probably due to providing more opportunities for the action of microbially secreted enzymes and increasing the bioavailability of biofilm to food. Similar to the above findings, there are similarities between the amount of COD removed and the HRT of other studies and researches; for example, in studies conducted by Nabizadeh and Mesdaghinia, increasing the HRT from 4 to 11 h (fixed inlet COD concentration of 500 mg L−1 (glucose carbon source)), the efficiency of the fixed bed biofilm reactor (FBBR) in COD removal increased, and the outlet COD concentration decreased from 39.88 mg L−1 to 22.45 mg L−1.35 Similarly, the study of Delnavaz et al. (2009) for removing aniline by a MBBR also exhibited that the maximum aniline removal efficiency was obtained at the highest HRT was 72 h.36 Also, the findings of Qaderi et al. (2011) showed that with increasing HRT and at equal organic loads, the efficiency of MBBR in formaldehyde removal increased well.37
3.5.2. Filling ratio. The results of the evaluation of the effect of filling ratio on the removal efficiency of 2,4-D herbicide and COD in a media-containing reactor were presented in Fig. 5. According to the results represented in Fig. 5, with increasing the filling ratio, the system efficiency has also developed. In biofilm systems, the treatment efficiency depends mainly on the availability level for biofilm growth, which depends on the carrier's characteristics and filling ratio. Therefore, it can be said that the filling ratio is directly affected by the biological activity of microbes in a bioreactor.35 This means that increasing the filling ratio increases the surface area for biofilm growth. In this study, with increasing the filling ratio from 30% to 50% and 70%, the removal efficiency of 2,4-D herbicide and COD increased and this variable in the present study is completely consistent with the studies conducted by Feng et al.38 and Shokoohi et al.39 Of course, the maximum filling ratio is 70% because it will not be possible for the media to rotate freely in the reactor.39 The results of the present study showed that the removal efficiencies of 2,4-D herbicide and COD at filling ratio of 70% and HRT of 24 h were 97.37% and 88.95%, respectively, and these values were obtained to be 78.36% and 69.48% at filling ratio of 30%.
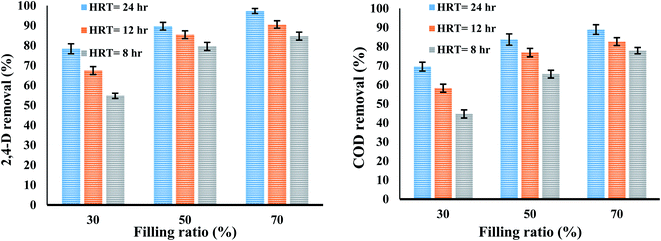 |
| Fig. 5 The effect of filling ratio and hydraulic retention time on the removal efficiency of 2,4-D and COD herbicide in a media-containing reactor. | |
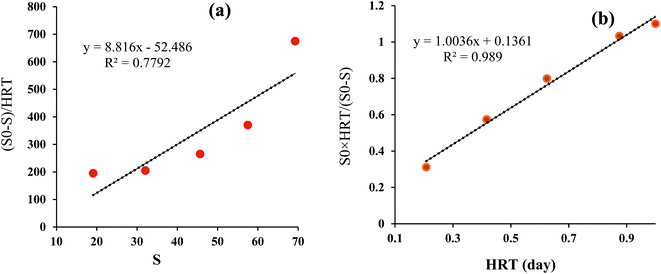 |
| Fig. 6 Degradation reaction kinetics of 2,4-D herbicide in terms of COD removal rate in MBBR process (a) first-order model and (b) second-order (Grau) model. | |
3.6. Reaction kinetics for the MBBR biological process
The purpose of studying the biological kinetics in wastewater treatment is to adapt the reactors and the reaction speed to determine the efficiency of the process. The Grau first and second-order kinetic model results were presented in Table S4† and Fig. 6. According to the results, the correlation coefficients for Grau first and second-order kinetic models were 0.7792 and 0.989, respectively. Also, the constant rate for Grau first and second-order kinetic models was 8.816 and 1.003, respectively. According to the obtained results, the kinetics of the degradation reaction of herbicide 2,4-D in terms of COD removal rate highly follows the second-order kinetic model (0.989) while to a lesser extent follows the first-order kinetics (0.7792). In the study conducted by Ahmadi et al. for the removal of diethyl phthalate and diallyl phthalate by the MBBR biological process, the reaction kinetics followed quadratic kinetics (Grau) with regression (R2) of 0.9883 and 0.9815, respectively.40 The results of a similar study by Delnavaz et al. showed that the treatment of aniline-containing wastewater by the MBBR biological process follows the Grau reaction kinetics with regression of 0.996.36 Also, in a study conducted by AghaBeiki et al. for the treatment of industrial wastewater by the MBBR biological process, the results showed that the MBBR biological process in the removal of wastewater COD follows the Grau second reaction kinetics with regression of 0.9973,19 which is consistent with the present study. Regarding the parameters m and n in the Grove model, it can be said that increasing each of these two parameters has a direct negative effect on efficiency, and by reducing these parameters, the removal efficiency in the biological process increases.
3.7. Identification of 2,4-D herbicide degrading bacteria in the MBBR process
Knowing that in biological treatment systems, microorganisms are responsible for the treatment of contaminants, and on the other hand, the effective microbial species in the treatment process vary according to the type of pollutant and the type of treatment system, thus, the identification and introduction of these microbes is useful for designers and operators of treatment systems as well as various researchers, especially environmental and biotechnology specialists. After isolation of microorganisms in general, differential and nutrient media, the isolated bacteria were identified using the available tests. The 2,4-D herbicide-degrading bacteria identified in this study were presented in Table 5. The results showed that the presence of E. coli, Enterobacter spp, Bacillus spp, Alcaligenes spp, Proteus spp, Acinetobacter spp, Pseudomonas spp, Arthrobacter and Brevundimonas vesicularis played a major role in the removal of 2,4-D herbicide from synthetic wastewater. This study's results are in line with similar results obtained from other studies on adherent growth systems and treatment systems in which the microorganisms that make up the biofilm are responsible for removing organic matter. The high ability of Pseudomonas to break down organic matter depends not only on their ability to produce catabolic enzymes, but also on their ability to regulate metabolic pathways.41 In another study by Bandyopadhyay et al., the ability of Pseudomonas putida MTCC 1194 was evaluated to degrade phenol at concentrations of 100–1000 mg L−1. Based on this study's results, the inhibitory effect of phenol on the phenol degradation process by the mentioned bacterium has prevailed in concentrations higher than 500 mg L−1.42 The results of Smejkal et al. showed that the bacterium Brevundimonas vesicularis can degrade herbicides called 4-(2,4-dichlorophenoxy)butyric acid and 4-(4-chloro-methylphenoxy)butyric acid,43 which is consistent with the results of the present study.
Table 5 Characteristics of 2,4-D herbicide-degrading bacteria isolated and identified in the biological layer formed on the substrate or media
Bacteria |
Morphology |
Catalase |
Oxidase |
TSI |
Urease |
Move |
Of |
Gelatinase |
Citrate |
MR |
VP |
Escherichia coli |
Gram-negative bacilli |
Pos |
Neg |
A/A |
Neg |
Mobile |
Aerobic – facultative anaerobe |
Neg |
Neg |
Pos |
Neg |
Pseudomonas alcaligenes |
Gram-negative bacilli |
Pos |
Pos |
A/A |
Neg |
Mobile |
Aerobic |
Neg |
Pos |
Neg |
Pos |
Acinetobacter |
Gram-negative bacilli |
Pos |
Neg |
K/K |
Neg |
Non-moving |
Highly aerobic |
Neg |
Pos |
— |
— |
Pseudomonas aeruginosa |
Gram-negative bacilli |
Pos |
Pos |
K/K |
Pos |
Mobile |
Aerobic |
Pos |
Pos |
— |
— |
Bacillus |
Gram positive coccobacilli https://www.ncbi.nlm.nih.gov/books/NBK470553/ |
Pos |
Neg |
Neg |
Neg |
Mobile |
Aerobic |
Pos |
Pos |
Neg |
Neg |
Enterobacter |
Gram-negative bacilli |
Pos |
Neg |
A/A |
Neg |
Mobile |
Aerobic – facultative anaerobe |
Neg |
Pos |
Neg |
Pos |
Brevundimonas vesicularis |
Gram-negative bacilli |
Pos |
Pos |
K/K |
Neg |
Pos |
— |
Pos |
Neg |
— |
— |
Morexella |
Gram negative coccobacilli |
Pos |
Pos |
K/K |
Neg |
Neg |
— |
Neg |
Neg |
— |
— |
3.8. The efficiency of combined process on actual agricultural drainage
The hybrid process's efficiency on real wastewater (actual agricultural drainage) was performed under optimal test conditions (electrolysis time of 100 minutes and current density of 9 mA cm−2) for the 3DE process. The characteristics of actual agricultural drainage before and after the electrochemical process are presented in Table 6. The results showed that the removal efficiencies of 2,4-D herbicides, TOC, and COD by this process was obtained to be 100%, 70%, and 89%, respectively, which specifies the high efficiency of this process in the treatment of wastewater containing moderate concentrations of herbicides. According to the results of this study, the 3DE process has a high ability to eliminate moderate concentrations of herbicides (<20 mg L−1) and if the concentration of herbicides is high, the hybrid system (3DE and MBBR biological processes) can be used. Jaafarzadeh et al. (2018)44 in a study examined the amount of 2,4-D herbicide in the effluent of agricultural drains in Haft Tappeh, Khuzestan province. The results showed that the amount of this type of herbicide in agricultural drainage effluent was about 4.0 mg L−1, which is much higher than the standard. However, although the amount of 2,4-D herbicide in the agricultural drainage effluent of Parsabad Moghan was about 4 times higher than the study of Jafarzadeh et al. (16.0 mg L−1), the 3DE process used in this study was able to remove this herbicide up to 100%.
Table 6 Characteristics of agricultural drainage sample quality before and after treatment by 3DE process
Parameter |
Unit |
Influent |
Effluent |
Removal efficiency (%) |
Not detected. |
TDS |
mg L−1 |
1386.0 ± 3.78 |
1284.0 ± 5.16 |
— |
EC |
μS cm−1 |
264.03 ± 3.66 |
2315.0 ± 4.89 |
— |
COD |
mg L−1 |
113.4 ± 1.51 |
12.6 ± 0.27 |
88.8 ± 0.49 |
TOC |
mg L−1 |
60.0 ± 2.12 |
18.0 ± 0.51 |
70.0 ± 0.58 |
2,4-D herbicide |
mg L−1 |
16.2 ± 0.86 |
NDa |
NDa |
pH |
— |
7.5 ± 0.45 |
7.4 ± 0.44 |
— |
3.9. Mechanism of 2,4-D herbicide degradation by hybrid process (electrochemical and biological)
Results related to the degradation mechanism of 2,4-D herbicide by hybrid process (3DE and biological MBBR processes) under optimal test conditions (pH of 5.0, HRT of 100 min, current density of 9 mA cm−2, and herbicide concentration 2,4-D of 100 mg L−1 for the 3DE process and filling ratio of 70% and HRT of 24 h for the MBBR biological process) were shown in Fig. 7. The results for LC-MS chromatograms of 2,4-D herbicides for conditions after 3DE decomposition and after biodegradation by MBBR biological process were shown in Fig. S2.† The analysis of each of the intermediates is presented in Table 7. In Fig. S2a and b,† the presence of the desired molar mass for each of the intermediates in the corresponding spectrum for the combined process of the 3DE and MBBR biological processes is evidence of the correct pathway of degradation of the herbicide 2,4-D. According to the analysis of each of the intermediates (Table 7), it is observed that due to the use of sodium salt in the reaction solution, the mass of sodium atoms is observed in the proposed structures. Then, during the degradation of the molecule, it becomes smaller components and the intermediates of the raw materials due to degradation are observed in the mass spectrum.
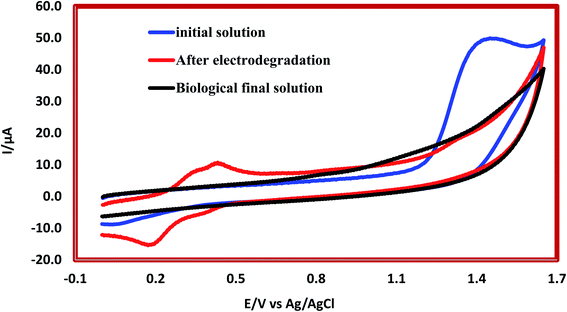 |
| Fig. 7 Cyclic voltammograms of herbicide 2,4-D by hybrid system of 3D electrochemical with graphite anode electrode coated (G/β-PbO2) and biological MBBR system. | |
Table 7 Intermediates produced during 2,4-D degradation in the MBBR–3DE processes by LC-MS chromatograms
No. |
Intermediates identified in the positive mass spectrum |
Structure name |
m/z |
1 |
Sodium 2-(2,4-dichloro-3,5,6-trioxidophenoxy)acetate |
353–355 |
2 |
Sodium 2-(6-hydroxy-2,3,4-trioxidophenoxy)acetate |
304–307 |
3 |
Sodium 2-(4-chloro-2-trioxidophenoxy)acetate |
223 |
4 |
2-(2,4-Dichlorophenoxy)acetic acid |
221–223 |
5 |
Sodium 2-(2,4-dichlorophenoxy)acetate |
206 |
6 |
2(2,4-Dichlorophenoxy)acetaldehyde |
205–206 |
7 |
3,6-Dichloro-benzene-1,2,4-triol |
195 |
8 |
2,4-Dichlorophenol |
161–163 |
9 |
1-Chloropropane |
78 |
10 |
2-Chlorobenzene-1,4-diol |
143–144 |
11 |
1,3,5-Trimethylbenzene |
120 |
12 |
Benzylideneoxonium |
105 |
13 |
Benzene |
79 |
14 |
Cyclopenta-2,4-dien-1-ylium |
64 |
4. Conclusion
The results showed that by increasing the hydraulic retention time and increasing the filling ratio, the 2,4-dichlorophenoxyacetic acid (2,4-D) herbicide and COD removal efficiencies were increased; so that the highest removal efficiencies were obtained in filling ratio of 70% and hydraulic retention time (HRT) of 24 h. The biological reaction kinetics followed the Grau second-order model (0.989). Also, according to the results, the 2,4-D herbicide-degrading bacteria identified in this study included E. coli, Enterobacter spp, Bacillus spp, Alcaligenes spp, Proteus spp, Acinetobacter spp, Pseudomonas spp, Arthrobacter and Brevundimonas vesicularis. In general, it can be concluded that the three-dimensional electrochemical (3DE) process can completely degrade the herbicide at lower concentrations, but in the presence of herbicides with higher concentrations, the combined 3DE processes with the biological system should be used.
Conflicts of interest
There are no conflicts to declare.
Acknowledgements
This research has been extracted from a Ph.D. thesis at Hamadan University of Medical Sciences. The study was supported by Hamadan University of Medical Sciences (No. 9611177276).
References
- M. R. Samarghandi, D. Nemattollahi, G. Asgari, R. Shokoohi, A. Ansari and A. Dargahi, Sep. Sci. Technol., 2019, 54, 478–493 CrossRef CAS.
- N. Kamarudin, R. Jusoh, A. Jalil, H. Setiabudi and N. Sukor, Chem. Eng. Res. Des., 2020, 159, 300–314 CrossRef CAS.
- A. Dargahi, A. Ansari, D. Nematollahi, G. Asgari, R. Shokoohi and M. R. Samarghandi, RSC Adv., 2019, 9, 5064–5075 RSC.
- M. Pirsaheb, A. Dargahi, S. Hazrati and M. Fazlzadehdavil, Desalin. Water Treat., 2014, 52, 4350–4355 CrossRef CAS.
- C. Viriato, F. M. França, D. S. Santos, A. S. Marcantonio, C. Badaró-Pedroso and C. M. Ferreira, Chemosphere, 2020, 129018 Search PubMed.
- M. R. Samarghandi, M. Mohammadi, A. Karami, L. Tabandeh, A. Dargahi and F. Amirian, Pol. J. Environ. Stud., 2017, 26, 2189–2195 CrossRef CAS.
- Q. A. Binh and H.-H. Nguyen, Bioresource Technology Reports, 2020, 11, 100520 CrossRef.
- A. F. E. González, H. Zúñiga-Benítez and G. A. Peñuela, Emerg. Contam., 2019, 5, 303–307 CrossRef.
- W. Yang, M. Zhou, N. Oturan, Y. Li, P. Su and M. A. Oturan, Electrochim. Acta, 2019, 297, 582–592 CrossRef CAS.
- X. Bian, J. Chen and R. Ji, Materials, 2013, 6, 1530–1542 CrossRef CAS PubMed.
- S. Pulkka, M. Martikainen, A. Bhatnagar and M. Sillanpää, Sep. Purif. Technol., 2014, 132, 252–271 CrossRef CAS.
- K. Thirugnanasambandham, V. Sivakumar and J. P. Maran, J. Taiwan Inst. Chem. Eng., 2015, 46, 160–167 CrossRef CAS.
- W. Kong, B. Wang, H. Ma and L. Gu, J. Hazard. Mater., 2006, 137, 1532–1537 CrossRef CAS PubMed.
- X. Li, W. Zhu, C. Wang, L. Zhang, Y. Qian, F. Xue and Y. Wu, Chem. Eng. J., 2013, 232, 495–502 CrossRef CAS.
- W. Liu, Z. Ai and L. Zhang, J. Hazard. Mater., 2012, 243, 257–264 CrossRef CAS PubMed.
- A. D. Santos, R. C. Martins, R. M. Quinta-Ferreira and L. M. Castro, Energy Reports, 2020, 6, 340–344 CrossRef.
- A. Shitu, S. Zhu, W. Qi, M. A. Tadda, D. Liu and Z. Ye, J. Environ. Manage., 2020, 275, 111264 CrossRef CAS PubMed.
- M. R. Samarghandi, A. Dargahi, A. Shabanloo, H. Z. Nasab, Y. Vaziri and A. Ansari, Arabian J. Chem., 2020, 13, 6847–6864 CrossRef CAS.
- S. AghaBeiki, A. S. Rad and A. Shokrolahzadeh, RSC Adv., 2016, 6, 113737–113744 RSC.
- A. Azizi, A. Dargahi and A. Almasi, Toxin Rev., 2019, 1–11 CrossRef.
- R. Shokoohi, A. J. Jafari, A. Dargahi and Z. Torkshavand, Desalin. Water Treat., 2017, 77, 256–263 CrossRef CAS.
- M. Faridnasr, B. Ghanbari and A. Sassani, Bioresour. Technol., 2016, 208, 149–160 CrossRef CAS PubMed.
- M. Molla Mahmoudi, R. Khaghani, A. Dargahi and G. Monazami Tehrani, Int. J. Environ. Anal. Chem., 2020, 1–26 CrossRef.
- APHA, AWWA and WPCF, Standard method for the examination of water and wastewater, Washington DC, 21st edn, 2012 Search PubMed.
- D. H. Bergey and H. J. G. Williams, 2000, pp. 106–180.
- L. Wang, F. Pan and L. Wang, Int. J. Electrochem. Sci., 2014, 9, 3628–3636 Search PubMed.
- Q. Zhuo, Q. Xiang, H. Yi, Z. Zhang, B. Yang, K. Cui, X. Bing, Z. Xu, X. Liang and Q. Guo, J. Electroanal. Chem., 2017, 801, 235–243 CrossRef CAS.
- A. Ansari and D. Nematollahi, Water Res., 2018, 144, 462–473 CrossRef CAS PubMed.
- X. Hao, S. Dan, Z. Qian, Y. Honghui and W. Yan, RSC Adv., 2014, 4, 25011–25017 RSC.
- N. Tran, P. Drogui, T. L. Doan, T. S. Le and H. C. Nguyen, Environ. Technol., 2017, 38, 2939–2948 CrossRef CAS PubMed.
- A. Rahmani, G. Azarian, S. Valadbeigi and S. Shanesaz, J. Health, 2018, 9, 204–214 CrossRef.
- Q. Dai, J. Zhou, M. Weng, X. Luo, D. Feng and J. Chen, Sep. Purif. Technol., 2016, 166, 109–116 CrossRef CAS.
- F. Ferrag-Siagh, F. Fourcade, I. Soutrel, H. Aït-Amar, H. Djelal and A. Amrane, Environ. Sci. Pollut. Res., 2014, 21, 8534–8542 CrossRef CAS PubMed.
- T. Tokimoto, N. Kawasaki, T. Nakamura, J. Akutagawa and S. Tanada, J. Colloid Interface Sci., 2005, 281, 56–61 CrossRef CAS PubMed.
- R. Nabizadeh and A. Mesdaghinia, J. Chem. Technol. Biotechnol., 2006, 81, 1209–1217 CrossRef CAS.
- M. Delnavaz, B. Ayati and H. Ganjidoust, Iranian Journal of Health and Environment, 2009, 2, 76–87 Search PubMed.
- F. Qaderi, B. Ayati and H. Ganjidoust, Iran. J. Environ. Health Sci. Eng., 2011, 8, 295–306 CAS.
- Q. Feng, Y. Wang, T. Wang, H. Zheng, L. Chu, C. Zhang, H. Chen, X. Kong and X.-H. Xing, Bioresource Technology Reports, 2012, 117, 201–207 CrossRef CAS PubMed.
- R. Shokoohi, Z. Torkshavand, H. Zolghadnasab, M. Y. Alikhani and M. S. Hemmat, Water Sci. Technol., 2018, 2017, 1–7 CrossRef PubMed.
- E. Ahmadi, M. Gholami, M. Farzadkia, R. Nabizadeh and A. Azari, Bioresour. Technol., 2015, 183, 129–135 CrossRef CAS PubMed.
- R. Shokoohi, M. Hajia, A. Jonaidi, H. M. Attar and A. Parvaresh, J. Water Wastewater, 2005, 55, 24–30 Search PubMed.
- K. Bandyopadhyay, D. Das and B. Maiti, Bioprocess Eng., 1998, 18, 373–377 CAS.
- C. Smejkal, F. Seymour, S. Burton and H. Lappin-Scott, J. Ind. Microbiol. Biotechnol., 2003, 30, 561–567 CrossRef CAS PubMed.
- N. Jaafarzadeh, F. Ghanbari and A. Zahedi, Journal of Water Process Engineering, 2018, 22, 203–209 CrossRef.
Footnote |
† Electronic supplementary information (ESI) available. See DOI: 10.1039/d0ra10821a |
|
This journal is © The Royal Society of Chemistry 2021 |