DOI:
10.1039/D0RA10726C
(Paper)
RSC Adv., 2021,
11, 10309-10315
SrTi(IO3)6·2H2O and SrSn(IO3)6: distinct arrangements of lone pair electrons leading to large birefringences†
Received
22nd December 2020
, Accepted 3rd March 2021
First published on 10th March 2021
Abstract
Three new iodates SrTi(IO3)6·2H2O, (H3O)2Ti(IO3)6, and SrSn(IO3)6 have been synthesized via a facile hydrothermal method. The three compounds have zero-dimensional crystal structures composed of one [MO6]8− (M = Ti, Sn) octahedron connected with six [IO3]− trigonal pyramids. However, the particular coordination of Sr2+ cations results in distinct arrangements of lone pair electrons in an [IO3]− trigonal pyramid, which leads to large birefringences. More importantly, this work enriches the species crystal chemistry for [M(IO3)6]2− (M = Ti, Sn) clusters-containing iodates.
Introduction
For a compound, the structure determines its physicochemical properties and the fields of potential applications.1,2 And a lot of high-performance iodate compounds have been reported owing to the unique arrangements of I–O anion groups, such as NaI3O8, Bi(IO3)F2, Cs2I4O11, ABi2(IO3)2F5 (A = K, Rb, Cs), LiMII(IO3)3 (MII = Zn and Cd), and AgI3O8.3 There are two types of coordination modes for I5+ cations ([IO3]− and [IO4]3−) since the weak I–O interactions will be neglected if the I–O bond lengths are larger than 2.4 Å, which result in the limited structural diversity of metal iodates.3f
Therefore, some metal cations (Ti4+, V5+, Cr6+, Pt4+, Ge4+, Sn4+, Mo6+, Mo4+, Zr4+, and Nb5+) and nonmetal cations (Se4+ and Te4+) have been introduced into iodates to enrich the structural chemistry and enhance the properties.4 And interestingly, through introducing homo-valence Mo4+, Zr4+, Ge4+, Ti4+, Pt4+, and Sn4+ metal cations into iodates, the similar [M(IO3)6]2− clusters (M = Mo, Zr, Ge, Ti, Pt, and Sn) can be formed, which possess [MO6]8− (M = Mo, Zr, Ge, Ti, Pt, and Sn) octahedron connected with six [IO3]− trigonal pyramids. The [M(IO3)6]2− clusters (M = Mo, Zr, Ge, Ti, Pt, and Sn) can be isostructural when they have the same arrangement of [IO3]− trigonal pyramids, which will be isostructural for metal iodates.4a,e,h,5
Moreover, the birefringence of iodates has also been comprehensively studied due to the stereochemical activity of the lone pair (SCALP) of I5+ in the [IO3]− group. The SCALP should be considered as a vital factor to influence the birefringence, such as Sn2+ with SCALP enhancing the birefringence in BaSn2(PO4)2 and Sn2B5O9Cl.6 While for iodates, the I5+ cation with SCALP in the [IO3]− group can also influence the birefringence apparently when the [IO3]− groups are arranged regularly. And introducing [IO3]− groups into borates and phosphates can significantly increase the birefringence such as metal borate-iodates Sr[B(OH)4](IO3) and Li4Sr5[B12O22(OH)4](IO3)2, as well as the metal iodate-phosphate Pb2(IO3)(PO4).7
Herein, three new Ti4+ or Sn4+-containing iodates, SrTi(IO3)6·2H2O (1), (H3O)2Ti(IO3)6 (2), and SrSn(IO3)6 (3) were synthesized through the mild hydrothermal method. And all of them possess zero-dimensional crystal structures which are composed of [M(IO3)6]2− (M = Ti, Sn) clusters and cations filling in voids among the [M(IO3)6]2− (M = Ti, Sn) clusters. The [M(IO3)6]2− (M = Ti, Sn) cluster consists of [MO6]8− (M = Ti, Sn) octahedra connected with six [IO3]− trigonal pyramids through sharing vertex O atoms. They are not isostructural although crystalizing in centrosymmetric space group R
(No. 148). Whereas, the particular coordination of Sr2+ cations filling in voids leads to the distinct arrangements of lone pair electrons in the [IO3]− trigonal pyramids and results in large birefringences. More importantly, compounds 1 and 2 are enriching the species of [Ti(IO3)6]8− groups and crystal water molecules-containing iodates. Compound 3 is the first alkaline-earth metal and [Sn(IO3)6]8− groups-containing iodate.
Experimental section
Crystal growth
Sr(OH)2·8H2O (0.034 g) (Aladdin Industrial Co., Ltd., 99.5%), HIO3 (0.446 g) (Aladdin Industrial Co., Ltd., 99.5%), TiO2 (Tianjin Fuchen Chemical Co., Ltd., 99%), and SnO (Aladdin Industrial Co., Ltd., 99.5%) were used as received from commercial sources without any further purification.
The starting reagents are Sr(OH)2·8H2O (0.034 g), TiO2 (0.020 g), HIO3 (0.446 g) for compound 1, TiO2 (0.022 g), HIO3 (0.478 g) for compound 2 and Sr(OH)2·8H2O (0.056 g), SnO (0.059 g), HIO3 (0.383 g) for compound 3, which are mixed respectively and put into PTFE pouches. Then these pouches were enclosed in 120 mL autoclaves with PTFE inner linings and added 15 mL of deionized water and heated at 190 °C for 3 days. The pouches were cooled at a rate of 0.6 °C h−1 to 130 °C and then 1 °C h−1 to room temperature. After cleaned with deionized water, the colorless block crystals were obtained. Crystal samples can also be obtained based on the following reaction:
Sr(OH)2·8H2O + TiO2 + 6HIO3 → SrTi(IO3)6 + 12H2O |
TiO2 + 6HIO3 → (H3O)2Ti(IO3)6 |
2Sr(OH)2·8H2O + 2SnO + 12HIO3 + O2 → 2SrSn(IO3)6 + 24H2O |
Fig. 1 shows the photos of grown crystal grains and the simulated morphologies of the compounds. On the basis of the crystal structure, the theoretical morphology can be calculated through the Bravais–Friedel–Donnay–Harker (BFDH) theory.8 The grown crystals are in line with the simulated morphologies.
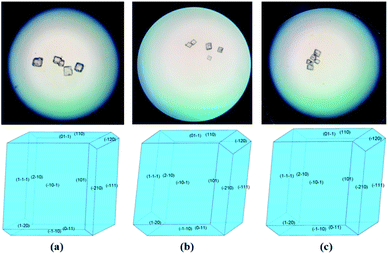 |
| Fig. 1 The grown crystal grains and simulated morphologies of (a) SrTi(IO3)6·2H2O, (b) (H3O)2Ti(IO3)6, and (c) SrSn(IO3)6, respectively. | |
Single-crystal X-ray crystallography
The single crystals selected manually are mounted on thin glass fibers using epoxy for data collection. The diffraction data collection of these compounds was conducted on a Bruker SMART APEX II CCD diffractometer with a Mo Kα radiation (λ = 0.71073 Å) at room temperature. And the data were integrated via SAINT, and then the crystal structures were solved with the direct method and refined by full-matrix least-squares fitting on F2 using the SHELX.9 The PLATON software verifies no other missed symmetry existed.10 The crystal information and structure refinements are recorded in Table 1. The atomic coordinates, equivalent isotropic displacement parameters, bond valence sum (BVS), and selected bond lengths and angles are given in Tables S1 and S2.†
Table 1 Crystal data and structure refinement for SrTi(IO3)6·2H2O, (H3O)2Ti(IO3)6, and SrSn(IO3)6, respectively
Empirical formula |
SrTi(IO3)6·2H2O |
(H3O)2Ti(IO3)6 |
SrSn(IO3)6 |
R1 = Σ‖Fo| − |Fc ‖/Σ|Fo| and wR2 = [Σw(Fo2 − Fc2)2/ΣwFo4]1/2 for Fo2 > 2σ(Fo2). |
Formula weight |
1220.95 |
1135.35 |
1255.71 |
Crystal system |
Trigonal |
Space group |
R![[3 with combining macron]](https://www.rsc.org/images/entities/char_0033_0304.gif) |
a/Å |
11.3720(11) |
11.31(3) |
10.807(7) |
b/Å |
12.5321(19) |
11.36(3) |
11.098(7) |
Volume/Å3 |
1403.6(3) |
1258(7) |
1122.5(16) |
Z, ρ calcd/g cm−3 |
3, 4.334 |
3, 4.495 |
3, 5.573 |
Theta range for data collection/° |
2.630 to 27.318 |
2.746 to 27.189 |
2.847 to 27.422 |
Reflections collected/unique |
2867/704 [R(int) = 0.0390] |
2567/628 [R(int) = 0.0501] |
2340/576 [R(int) = 0.0354] |
Completeness |
99.90% |
100.00% |
100.00% |
Goodness-of-fit on F2 |
1.053 |
0.977 |
1.095 |
Final R indices [I > 2sigma(I)]a |
R1 = 0.0191, wR2 = 0.0380 |
R1 = 0.0259, wR2 = 0.0360 |
R1 = 0.0172, wR2 = 0.0366 |
R Indices (all data)a |
R1 = 0.0221, wR2 = 0.0390 |
R1 = 0.0361, wR2 = 0.0382 |
R1 = 0.0196, wR2 = 0.0374 |
Extinction coefficient |
0.00224(7) |
0.00038(4) |
0.00128(7) |
Largest diff. Peak and hole/e Å−3 |
0.808 and −0.596 |
0.740 and −0.877 |
0.870 and −0.707 |
Powder X-ray diffraction
X-ray diffraction (XRD) measurements were carried out on a Bruker D2 PHASER diffractometer equipped with a diffracted beamed monochromator set for Cu Kα (λ = 1.54056 Å) radiation at room temperature in the 2θ range from 10 to 70° with a scan step width of 0.02° and a fixed counting time of 1 s per step. The experimental powder XRD patterns are well in agreement with the simulated ones (Fig. 2).
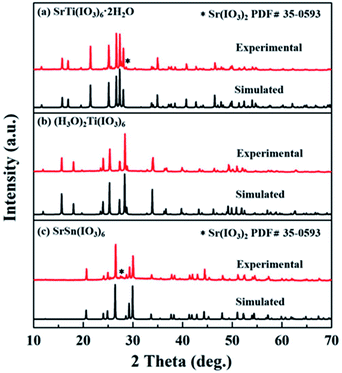 |
| Fig. 2 Experimental and calculated powder X-ray diffraction patterns of (a) SrTi(IO3)6·2H2O, (b) (H3O)2Ti(IO3)6, and (c) SrSn(IO3)6, respectively. | |
Infrared and Raman spectrum
The samples and dried KBr were mixed completely in the molar ratio of 1
:
100 and then pressed into a thin wafer to collect the infrared (IR) spectra through a Shimadzu IRAffinity-1 spectrometer. The samples were ground thoroughly and pressed to flat for collecting the Raman spectra on a LabRAM HR evolution equipment.
UV-vis-NIR transmission and photoluminescence spectrum
The UV-vis-NIR diffuse reflectance spectra were characterized via a Shimadzu SolidSpec-3700DUV spectrophotometer. The function F(R) = (1 − R)2/2R is applied to convert the reflectance spectra, where R is the reflectance and F(R) is the Kubelka–Munk remission function.11 Photoluminescence (PL) spectra were performed via FLS1000-Edinburgh Instruments.
Thermal and magnetically analysis
Using a NETZSCH STA 449C simultaneous thermal analyzer, compounds 1, 2, and 3 were placed in the platinum crucibles and heated from 40 to 1000, 500, and 800 °C at a rate of 5 °C min−1 under a flow of N2 to get TG and DSC data, respectively. Magnetic properties of the samples were performed by the DC magnetization measurements using a vibrating sample magnetometer (VSM, QUANTUM DESIGN).
Birefringent measurement
A polarizing microscope (ZEISS Axio Scope. A1) was used to study the birefringences (Δn) with a visible light filter at 546 nm. The optical path difference (R) was quantified under the cross-polarized light based on the maximum interference color.12 The formula for calculating the birefringence is
R = |Ne − No| × d = Δn × d, |
where Ne and No represent the refractive indexes of light through a crystal.
Theoretical calculations
To deeply explore the origin of optical properties, the first-principles calculation was carried out to simulate electron and band structures along with birefringence by the plane-wave pseudopotential method embedding in the CASTEP package.13 The Perdew–Burke–Ernzerhof functional within the generalized gradient approximation was applied for the exchange–correlation potential.14 The plane-wave cutoff energy was set at 830 eV with a separation of Monkhorst–Pack k-point sampling of 0.04 Å−1 for the aim of energy convergence.
Results and discussion
Crystal structure
Compound 1 crystallizes in a centrosymmetric trigonal space group R
(No. 148) with a = 11.3720(11) Å, c = 12.5321(19) Å, and Z = 3, however, it is not isostructural to BaTi(IO3)6, and A2Ti(IO3)6 (A = K, Rb, Cs, and Tl).5b,d There is one unique Sr atom, one unique Ti atom, one unique I atom, four unique O atoms, and two H atoms in the asymmetric unit. The Ti4+ cation is coordinated to six O atoms, forming the [TiO6]8− octahedron which is linked to six [IO3]− trigonal pyramids, and further forms a [Ti(IO3)6]2− group (Fig. 3a). The Ti–O bonds have a unique distance of 1.915(3) Å. The I5+ cations are coordinated to three O atoms in a distorted trigonal pyramidal with I–O bond lengths ranging from 1.790(3) to 1.843(3) Å. The Sr2+ cations are coordinated with eight O atoms to form a hexagonal bipyramid with six equatorial oxygen atoms (Fig. S2a†). The pyramid with Sr–O distances of 2.433(8)–2.664(3) Å, resides in the site formed by eight [Ti(IO3)6]2− groups. Bond valence sum (BVS) values were calculated to check the reliability of each atom (Table S1†).15 Only the bond valence of O1 (0.42) is not regular, so two hydrogen atoms should be coordinated to O1 to balance the bond valence. It should be noted that the one H+ cation is disordered over three sites with 66.7% occupancy on each site resulting from the three-fold axis through the O1 atom (Fig. 3b). The two [Ti(IO3)6]2− units are separated by one Sr2+ cation and two H2O molecules (Fig. 3c), and further form the zero-dimensional structure of compound 1 (Fig. 3d).
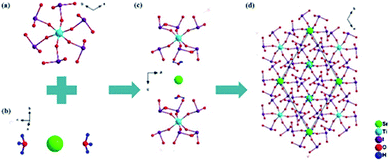 |
| Fig. 3 Ball and stick structure of SrTi(IO3)6·2H2O. (a) The basic building unit [Ti(IO3)6]2− of SrTi(IO3)6·2H2O. (b) The Sr2+ cation and H2O molecules. (c) Two [Ti(IO3)6]2− units separated by one Sr2+ cation and two H2O molecules. (d) The final zero-dimensional structure of SrTi(IO3)6·2H2O. | |
Compounds 2 and 3 also crystallize in centrosymmetric trigonal space groups R
(No. 148) and have similar structures to compound 1 (Fig. S1 and S2†), so the detailed structures are not demonstrated here. And compound 2 is isostructural to β-(H3O)2Pt(IO3)6 reported by Mao's group.5c And compound 3 is not isostructural to A2Sn(IO3)6 (A = K, Rb, Cs) and Sn2+Sn4+(IO3)6.
Compounds 1, 2, and 3 possess the different arrangements of [M(IO3)6]2− (M = Ti, Sn) groups even though all crystallize in the same space group R
. Owing to the unique M–O (M = Ti, Sn) bond distances in each compound, the [MO6]8− (M = Ti, Sn) octahedra have no out of center distortion.16 So the main difference is the condition of [IO3]− trigonal pyramid. Each I5+ cation in these compounds is bonded to three oxygen atoms, and owing to its lone electron pair, an [IO3]− trigonal pyramidal coordination environment is observed. For compound 1, the planes formulated via three oxygen atoms in [IO3]− trigonal pyramid have two angle values (41.264 and 48.736°) with the c-axis in each compound (Fig. 4a). However, for compounds 2 and 3, the corresponding unique angle values are 8.296 and 30.384° with the c-axis, respectively (Fig. 4b and c). From Fig. S3,† except hydrogen atoms, there are two oxygen atoms and one strontium atom between the two adjacent [M(IO3)6]2− (M = Ti, Sn) groups for compound 1, while there are two oxygen atoms for compound 2 as well as one strontium atom for compound 3. The particular hexagonal bipyramid coordination of Sr2+ in compound 1 (Fig. S4a†) should result in taking up the place between the two H2O molecules (Fig. S3a†). And in order to maintain this coordination for compound 1, the arrangements of [IO3]− should rotate with respect to the [TiO6]8− octahedron, in which the rotation aligns the polarization from the [IO3]− groups in opposite directions. Alike to compound 1, in order to sustain special Sr2+ bipyramid coordination in compound 3 (Fig. S4b†), the arrangements of [IO3]− should rotate taking account of the [SnO6]8− octahedron (Fig. S3b†). So the angles of compounds 1 and 3 mentioned above are larger than that of compound 2.
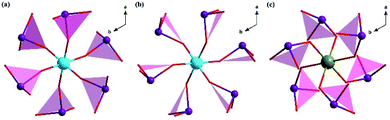 |
| Fig. 4 From the c-axis direction, the condition of planes formed by three O atoms in [IO3]− trigonal pyramid of a [Ti(IO3)6]2− group for (a) SrTi(IO3)6·2H2O, (b) (H3O)2Ti(IO3)6 and (c) SrSn(IO3)6, respectively. | |
IR and Raman spectroscopy
The IR and Raman spectra are shown in Fig. S5.† For compounds 1, and 2, IR spectra are similar except for the absorption bands at 2358 cm−1 in compound 2, which are caused by bending modes of H–O–H units.17 The IR absorption bands at 3500–2800 cm−1 can be assigned to the stretching vibrations of the H2O band, the absorption bands at 1850–1000 cm−1 are consistent with the bending and stretching vibrations of the H–O–H units.17 The absorption bands in the range of 890–750 cm−1 and 490–400 cm−1 are consistent with stretching and bending vibrations of the I–O groups.18 The IR absorption bands at 700, 704, and 671 cm−1 in the three compounds are caused by the [MO6]8− (M = Ti, Sn) units stretching vibrations.5b The Raman spectra were used to further verify the existence of the [M(IO3)6]2− (M = Ti, Sn) groups. The proposed assignments of observed positions of the Raman bands are illustrated in Table S4,† which are consistent with the reported results.19
UV-vis-NIR transmission and photoluminescence spectrum
Fig. 5a–c displays the UV-vis-NIR diffuse reflectance spectra. The cut-off edges of compounds 1, 2, and 3 are 318, 330, and 250 nm (inset of Fig. 5a–c), and the corresponding energy gaps are 3.38, 3.35, and 4.08 eV, respectively, which are close to those of the previously reported iodate crystals.5f,20 Fig. 5d shows the photoluminescence emission spectrum of the three compounds. The bands around 300 nm (4.13 eV) can be in corresponding with a result of ground state to their excited state electronic transitions. Photoluminescence in two broad bands at 360 nm (3.44 eV) for compound 3 and 370 nm (3.35 eV) for compounds 1 and 2 are associated with electronic transitions from the ground state to the conduction band selftrapped excitons, and the bands around 470 nm (2.64 eV) and 560 nm (2.21 eV) are excited most effectively at short wavelengths.21
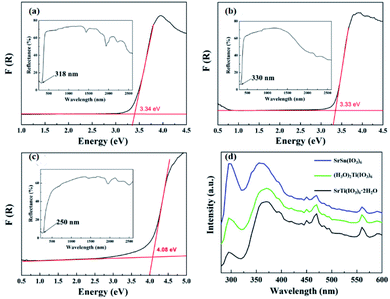 |
| Fig. 5 Optical absorption spectra of (a) SrTi(IO3)6·2H2O, (b) (H3O)2Ti(IO3)6, and (c) SrSn(IO3)6 as well as (d) PL spectra of the compounds. | |
Thermal and magnetic properties
The TG-DSC curves of the three compounds are shown in Fig. S6.† There are four remarkably endothermic peaks at 350, 450, 477, and 595 °C for compound 1, respectively (Fig. S6a†). In the range of 220–370 °C, there is a mass loss of 2.9%, which is consistent with the loss of two molecules of H2O with a calculated value of 3.0%.22 The TG curve in the range of 370–1000 °C shows a total mass loss of 80.9%, which corresponds to the decomposition process of three I2O5 molecules.23 The loss is consistent with the calculated value of 82.0%.
There are three distinct endothermic peaks at 220, 409, and 458 °C for compound 2 (Fig. S6b†). In the range of 120–227 °C it has a mass loss of 4.8%, which is consistent with the loss of three molecules of H2O with a calculated value of 4.7%.22 And there is a mass loss of 90.7% in the range of 227–500 °C, which is consistent with the decomposition process of three I2O5 molecules.23 The loss is consistent with the calculated value of 88.3%.
There are two distinct endothermic peaks at 492 and 566 °C for compound 2 (Fig. S6c†). And there is a mass loss of 80.8% in the range of 440–800 °C, which is consistent with the decomposition process of three I2O5 molecules.23 The loss is consistent with the calculated value of 79.8%.
The field-dependent magnetization plots are displayed in Fig. S7,† which shows that the three compounds are a para/antimagnetic nature at a low and high field.19g It has been reported that the SrTiO3 and SrSnO3 are diamagnetic.19g,24 Whereas, the para/antimagnetism of these compounds may come from introducing [IO3]− anion groups and further compensating the diamagnetism of inner electrons.
Theoretical calculations and birefringent analysis
The electronic structures of the three compounds are shown in Fig. 6. Compounds 1, 2, and 3 possess indirect band gaps of 2.50, 2.62, and 3.19 eV, respectively, which are always smaller than the experimental values.25 And from the PDOS of compounds 1 and 2, the bottom of conduction bands is mainly contributed by Ti-3d, I-5p, and O-2p states and the top of the valence bands mainly comes from I-5p, O-2p states. Moreover, for compound 3, the I-5p, O-2s, and O-2p states mainly determine both the bottom of conduction bands and the top of the valence bands. In other words, the [Ti(IO3)6]2− groups determine the Fermi level position of compounds 1 and 2, while the [IO3]− groups for compound 3.
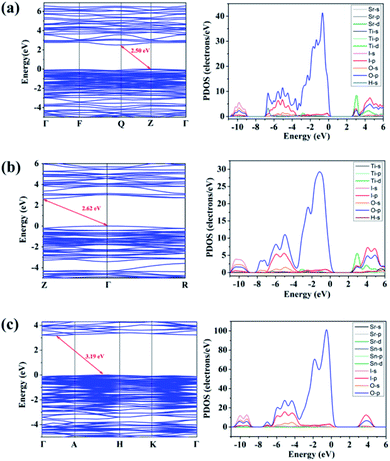 |
| Fig. 6 Band gaps, the partial density of states (PDOS) of (a) SrTi(IO3)6·2H2O, (b) (H3O)2Ti(IO3)6, and (c) SrSn(IO3)6. | |
The calculated birefringence values of compounds 1, 2, and 3 are shown in Fig. S8a.† Thereinto, compounds 1 and 3 possess larger birefringences compared to compound 2. Furthermore, the Δn values of compounds 1, 2, and 3 were measured by a ZEISS Axio Scope polarizing microscope (Table 2). According to the results, the retardations are about 1331, 906, and 2690 nm for compounds 1, 2, and 3, respectively. So the approximate experimental values of 0.093, 0.045, and 0.116 at 546 nm for these three compounds are consistent with the calculated results and verify the rationality of the calculations.
Table 2 Calculated and experimental birefringences at 546 nm of the three compounds and the contributions for birefringence of each functional group of SrTi(IO3)6·2H2O, and (H3O)2Ti(IO3)6 at the static limit derived from the cut-TiO group wave functions and cut-IO group wave functions
Birefringence |
SrTi(IO3)6·2H2O |
(H3O)2Ti(IO3)6 |
SrSn(IO3)6 |
Experimental |
0.093 |
0.045 |
0.116 |
Calculated |
0.109 |
0.038 |
0.128 |
IO group |
0.081 |
0.04 |
— |
TiO group |
0.018 |
0.035 |
— |
It is well known that the optical properties depend on the hybridization of electron orbitals around Femi level, which states that the birefringence is mainly affected by the response electron distribution of the [Ti(IO3)6]2− and [IO3]− groups for compounds 1, 2 and 3, respectively. Moreover, the [TiO6]8− octahedra in each compound are not out of center distortion, and therefore, the difference of [IO3]− in [Ti(IO3)6]2− groups plays a dominant role on the different birefringence, as the influence of [TiO6]8− octahedra can be in a little scale. To further analyze the [IO3]− groups to be the dominant factor on the amplification of birefringence, a real-space atom-cutting method was adopted for compounds 1 and 2 (Fig. S8(b) and (c)†). And for compound 3, we have tried many times and failed to get a reasonable result. From the PDOS of compound 3, the hybridization of [IO3]− trigonal pyramid is the main contribution to optical properties as well as birefringence. The condition of lone pair electrons in [IO3]− trigonal pyramid can be associated with the orientation of the plane formulated by three oxygen atoms. Meanwhile, it is known that the c-axis of the three compounds is the optical principle axis because they all crystalize in R
space group. For compounds 1 and 3, half of the lone pair electrons can be regarded as inclining to the positive c-axis in a large degree, and the rest to negative (Fig. S9a and c†). While, from the c-axis direction, the lone pair electrons of compound 2 are inclined to the positive or negative c-axis in a tiny magnitude (Fig. S9b†). These different arrangements of lone pair electrons may induce large birefringences for compounds 1 and 3 compared to compound 2. Besides, the higher density of [IO3]− in compound 3 may lead to a larger birefringence than compound 1 (the unit cell volume of compound 1 (1403.6(3) Å3) is larger than that of compound 3 (1122.5(16) Å3)).
Conclusion
In summary, three iodates, SrTi(IO3)6·2H2O, (H3O)2Ti(IO3)6, and SrSn(IO3)6, have been obtained successfully via the facile hydrothermal method. They crystallize in centrosymmetric space groups R
and possess zero-dimensional structures with isolated [M(IO3)6]2− (M = Ti, Sn) that are composed of [MO6] octahedra connected to six [IO3]− trigonal pyramids. The introduction of the Sr2+ cation results in the different arrangements of [IO3]− trigonal pyramids. The distinct arrangements of lone pair electrons of the I5+ cation lead to larger birefringences in SrTi(IO3)6·2H2O and SrSn(IO3)6 than that in (H3O)2Ti(IO3)6. And this work enriches the species crystal chemistry for [M(IO3)6]2− (M = Ti, Sn) clusters-containing iodates.
Conflicts of interest
There are no conflicts of interest to declare.
Acknowledgements
This work was financially supported by the West Light Foundation of the Chinese Academy of Sciences (2020-XBQNXZ-002), National Natural Science Foundation of China (U2003131, 51972336, 61835014, 11774414), Natural Science Foundation of Xinjiang, China (2019D01A93), Tianshan Youth Program of Xinjiang (2018Q035), and Tianshan Innovation Team Program (2018D14001).
References
-
(a) B. C. Wu, D. Y. Tang, N. Ye and C. T. Chen, Opt. Mater., 1996, 5, 105–109 CrossRef CAS;
(b) W. J. Yao, R. He, X. Y. Wang, Z. S. Lin and C. T. Chen, Adv. Opt. Mater., 2014, 2, 411–417 CrossRef CAS;
(c) Y. Wang and S. L. Pan, Coord. Chem. Rev., 2016, 323, 15–35 CrossRef CAS;
(d) G. Q. Shi, Y. Wang, F. F. Zhang, B. B. Zhang, Z. H. Yang, X. L. Hou, S. L. Pan and K. R. Poeppelmeier, J. Am. Chem. Soc., 2017, 139, 10645–10648 CrossRef CAS PubMed;
(e) B. B. Zhang, G. Q. Shi, Z. H. Yang, F. F. Zhang and S. L. Pan, Angew. Chem., Int. Ed., 2017, 56, 3916–3919 CrossRef CAS PubMed;
(f) G. Y. Yang and K. C. Wu, Inorg. Chem., 2018, 57, 7503–7506 CrossRef CAS PubMed;
(g) M. Mutailipu and S. L. Pan, Angew. Chem., Int. Ed., 2020, 59, 20302–20317 CrossRef CAS PubMed;
(h) H. P. Wu, S. L. Pan, K. R. Poeppelmeier, H. Y. Li, D. Z. Jia, Z. H. Chen, X. Y. Fan, Y. Yang, J. M. Rondinelli and H. S. Luo, J. Am. Chem. Soc., 2011, 133, 7786–7790 CrossRef CAS PubMed.
-
(a) Y. Wang, B. B. Zhang, Z. H. Yang and S. L. Pan, Angew. Chem., Int. Ed., 2018, 57, 2150–2154 CrossRef CAS PubMed;
(b) X. F. Wang, Y. Wang, B. B. Zhang, F. F. Zhang, Z. H. Yang and S. L. Pan, Angew. Chem., Int. Ed., 2017, 56, 14119–14123 CrossRef CAS PubMed;
(c) X. L. Chen, B. B. Zhang, F. F. Zhang, Y. Wang, M. Zhang, Z. H. Yang, K. R. Poeppelmeier and S. L. Pan, J. Am. Chem. Soc., 2018, 140, 16311–16319 CrossRef CAS PubMed;
(d) H. P. Wu, H. W. Yu, Z. H. Yang, X. L. Hou, X. Su, S. L. Pan, K. R. Poeppelmeier and J. M. Rondinelli, J. Am. Chem. Soc., 2013, 135, 4215–4218 CrossRef CAS PubMed;
(e) H. Q. Wu, P. Ju, H. He, B. F. Yang and G. Y. Yang, Inorg. Chem., 2013, 52, 10566–10570 CrossRef CAS PubMed;
(f) J. H. Huang, C. C. Jin, P. L. Xu, P. F. Gong, Z. S. Lin, J. W. Cheng and G. Y. Yang, Inorg. Chem., 2019, 58, 1755–1758 CrossRef CAS PubMed;
(g) M. Mutailipu, M. Zhang, B. B. Zhang, L. Y. Wang, Z. H. Yang, X. Zhou and S. L. Pan, Angew. Chem., Int. Ed., 2018, 57, 6095–6099 CrossRef CAS PubMed;
(h) Z. Z. Zhang, Y. Wang, B. B. Zhang, Z. H. Yang and S. L. Pan, Angew. Chem., Int. Ed., 2018, 57, 6577–6581 CrossRef CAS PubMed.
-
(a) D. Phanon and I. Gautier-Luneau, Angew. Chem., Int. Ed., 2007, 46, 8488–8491 CrossRef CAS PubMed;
(b) F. F. Mao, C. L. Hu, X. Xu, D. Yan, B. P. Yang and J. G. Mao, Angew. Chem., Int. Ed., 2017, 56, 2151–2155 CrossRef CAS PubMed;
(c) K. M. Ok and P. S. Halasyamani, Angew. Chem., Int. Ed., 2004, 43, 5489–5491 CrossRef CAS PubMed;
(d) H. M. Liu, Q. Wu, X. X. Jiang, Z. S. Lin, X. G. Meng, X. G. Chen and J. G. Qin, Angew. Chem., Int. Ed., 2017, 56, 9492–9496 CrossRef CAS PubMed;
(e) Y. J. Jia, Y. G. Chen, Y. Guo, X. F. Guan, C. Li, B. Li, M. M. Liu and X. M. Zhang, Angew. Chem., Int. Ed., 2019, 58, 17194–17198 CrossRef CAS PubMed;
(f) X. Xu, C. L. Hu, B. X. Li, B. P. Yang and J. G. Mao, Chem. Mater., 2014, 26, 3219–3230 CrossRef CAS.
-
(a) C. F. Sun, C. L. Hu and J. G. Mao, Chem. Commun., 2012, 48, 4220–4222 RSC;
(b) Z. G. Xia and K. R. Poeppelmeier, Acc. Chem. Res., 2017, 50, 1222–1230 CrossRef CAS PubMed;
(c) K. M. Ok, Acc. Chem. Res., 2016, 49, 2774–2785 CrossRef CAS PubMed;
(d) J. J. Zhang, Z. H. Zhang, W. G. Zhang, Q. X. Zheng, Y. X. Sun, C. Q. Zhang and X. T. Tao, Chem. Mater., 2011, 23, 3752–3761 CrossRef CAS;
(e) H. S. Ahn, D. W. Lee and K. M. Ok, Dalton Trans., 2014, 43, 10456–10461 RSC;
(f) C. F. Sun, C. L. Hu, X. Xu, J. B. Ling, T. Hu, F. Kong, X. F. Long and J. G. Mao, J. Am. Chem. Soc., 2009, 131, 9486–9487 CrossRef CAS PubMed;
(g) T. A. Sullens, P. M. Almond, J. A. Byrd, J. V. Beitz, T. H. Bray and T. E. Albrecht-Schmitt, J. Solid State Chem., 2006, 179, 1192–1201 CrossRef CAS;
(h) T. C. Shehee, S. F. Pehler and T. E. Albrecht-Schmitt, J. Alloys Compd., 2005, 388, 225–229 CrossRef CAS;
(i) Z. Qian, H. P. Wu, H. W. Yu, Z. G. Hu, J. Y. Wang and Y. C. Wu, Dalton Trans., 2020, 49, 8443–8447 RSC;
(j) C. Y. Meng, P. Zhang, R. F. Wang and L. Geng, Dalton Trans., 2017, 46, 12320–12327 RSC.
-
(a) C. F. Sun, C. L. Hu, F. Kong, B. P. Yang and J. G. Mao, Dalton Trans., 2010, 39, 1473–1479 RSC;
(b) K. M. Ok and P. S. Halasyamani, Inorg. Chem., 2005, 44, 2263–2271 CrossRef CAS PubMed;
(c) B. P. Yang, C. L. Hu, X. Xu and J. G. Mao, Inorg. Chem., 2016, 55, 2481–2487 CrossRef CAS PubMed;
(d) H. Y. Chang, S. H. Kim, K. M. Ok and P. S. Halasyamani, J. Am. Chem. Soc., 2009, 131, 6865–6873 CrossRef CAS PubMed;
(e) H.-Y. Chang, S.-H. Kim, P. S. Halasyamani and K. M. Ok, J. Am. Chem. Soc., 2009, 131, 2426–2427 CrossRef CAS PubMed;
(f) Y. H. Kim, T. T. Tran, P. S. Halasyamani and K. M. Ok, Inorg. Chem. Front., 2015, 2, 361–368 RSC.
-
(a) Y. Yang, Y. Qiu, P. F. Gong, L. Kang, G. M. Song, X. M. Liu, J. L. Sun and Z. S. Lin, Chem.–Eur. J., 2019, 25, 5648–5651 CrossRef CAS PubMed;
(b) J. Y. Guo, A. Tudi, S. J. Han, Z. H. Yang and S. L. Pan, Angew. Chem., Int. Ed., 2019, 58, 17675–17678 CrossRef CAS PubMed.
-
(a) G. Peng, C. S. Lin, D. Zhao, L. L. Cao, H. X. Fan, K. C. Chen and N. Ye, Chem. Commun., 2019, 55, 11139–11142 RSC;
(b) X. H. Zhang, B. P. Yang, J. Chen, C. L. Hu, Z. Fang, Z. J. Wang and J. G. Mao, Chem. Commun., 2020, 56, 635–638 RSC.
-
(a) C. F. Macrae, I. J. Bruno, J. A. Chisholm, P. R. Edgington, P. McCabe, E. Pidcock, L. Rodriguez-Monge, R. Taylor, J. Van De Streek and P. A. Wood, J. Appl. Crystallogr., 2008, 41, 466–470 CrossRef CAS;
(b) P. R. Edgington, P. McCabe, C. F. Macrae, E. Pidcock, G. P. Shields, R. Taylor, M. Towler and J. Van De Streek, J. Appl. Crystallogr., 2006, 39, 453–457 CrossRef.
-
(a) G. M. Sheldrick, Instruction of Bruker Analytical X-ray Instruments (version 7.60A), Bruker Inc., Madison, 2008 Search PubMed;
(b) G. M. Sheldrick, Acta Crystallogr., Sect. C: Struct. Chem., 2015, 71, 3–8 CrossRef PubMed.
- A. L. Spek, J. Appl. Crystallogr., 2003, 36, 7–13 CrossRef CAS.
-
(a) P. Kubelka and F. Munk, Z. Tech. Phys., 1931, 12, 593–601 Search PubMed;
(b) J. Tauc, Mater. Res. Bull., 1970, 5, 721–729 CrossRef CAS.
-
(a) B. E. Sørensen, Eur. J. Mineral., 2012, 25, 5–10 CrossRef;
(b) X. F. Wang, F. F. Zhang, L. Gao, Z. H. Yang and S. L. Pan, Adv. Sci., 2019, 6, 1901679 CrossRef CAS PubMed.
-
(a) B. G. Pfrommer, M. Côté, S. G. Louie and M. L. Cohen, J. Comput. Phys., 1997, 131, 233–240 CrossRef CAS;
(b) S. J. Clark, M. D. Segall, C. J. Pickard, P. J. Hasnip, M. J. Probert, K. Refson and M. C. Payne, Z. Kristallogr. - Cryst. Mater., 2005, 220, 567–570 CrossRef CAS.
-
(a) J. P. Perdew, K. Burke and M. Ernzerhof, Phys. Rev. Lett., 1996, 77, 3865–3868 CrossRef CAS PubMed;
(b) A. Rappe, K. Rabe, E. Kaxiras and J. Joannopoulos, Phys. Rev. B: Condens. Matter Mater. Phys., 1990, 41, 1227–1230 CrossRef PubMed.
-
(a) N. E. Brese and M. O'Keeffe, Acta Crystallogr., Sect. B: Struct. Sci., 1991, 47, 192–197 CrossRef;
(b) F. F. Zhang, K. Y. Li, H. Ratajczak and D. F. Xue, J. Mol. Struct., 2010, 976, 69–72 CrossRef CAS.
- P. S. Halasyamani, Chem. Mater., 2004, 16, 3586–3592 CrossRef CAS.
-
(a) G. Niedner-Schatteburg, Angew. Chem., Int. Ed., 2008, 47, 1008–1011 CrossRef CAS;
(b) N. I. Hammer, E. G. Diken, J. R. Roscioli, M. A. Johnson, E. M. Myshakin, K. D. Jordan, A. B. McCoy, X. Huang, J. M. Bowman and S. Carter, J. Chem. Phys., 2005, 122, 244301 CrossRef PubMed.
- Y. H. Li, G. P. Han, H. W. Yu, H. Li, Z. H. Yang and S. L. Pan, Chem. Mater., 2019, 31, 2992–3000 CrossRef CAS.
-
(a) J. R. Durig, O. D. Bonner and W. H. Breazeale, J. Phys. Chem., 1965, 69, 3886–3892 CrossRef CAS;
(b) A. Gajović, I. Friščić, M. Plodinec and D. Ivekovic, J. Mol. Struct., 2009, 924, 183–191 CrossRef;
(c) W. G. Nilsen and J. G. Skinner, J. Chem. Phys., 1968, 48, 2240–2248 CrossRef CAS;
(d) R. Ma, K. Fukuda, T. Sasaki, M. Osada and Y. Bando, J. Phys. Chem. B, 2005, 109, 6210–6214 CrossRef CAS PubMed;
(e) B. Muthukutty, R. Karthik, S.-M. Chen and M. Abinaya, New J. Chem., 2019, 43, 12264–12274 RSC;
(f) B. X. Huang, P. Tornatore and Y.-S. Li, Electrochim. Acta, 2001, 46, 671–679 CrossRef;
(g) A. Manoharan, M. Munusamy, A. Pradeep, S. Sellaiyan, S. Hussain and S. Krishnan, Appl. Phys. A: Mater. Sci. Process., 2020, 126, 874 CrossRef CAS;
(h) S. Sharma, H. Pandey, M. Kumar and S. Chhoker, Superlattices Microstruct., 2018, 120, 161–169 CrossRef CAS.
-
(a) Z. B. Cao, Y. C. Yue, J. Y. Yao, Z. S. Lin, R. He and Z. G. Hu, Inorg. Chem., 2011, 50, 12818–12822 CrossRef CAS PubMed;
(b) R. E. Sykora, K. M. Ok, P. S. Halasyamani and T. E. Albrecht-Schmitt, J. Am. Chem. Soc., 2002, 124, 1951–1957 CrossRef CAS PubMed;
(c) T. Abudouwufu, M. Zhang, S. C. Cheng, Z. H. Yang and S. L. Pan, Chem.–Eur. J., 2018, 25, 1221–1226 CrossRef PubMed;
(d) Y. W. An, Y. Zhong, T. Q. Sun, H. J. Wang, Z. P. Hu, H. D. Liu, S. G. Liu, Y. F. Kong and J. J. Xu, Dalton Trans., 2019, 48, 13074–13080 RSC;
(e) Y. J. Jia, Y. G. Chen, T. Wang, Y. Guo, X. F. Guan and X. M. Zhang, Dalton Trans., 2019, 48, 10320–10326 RSC.
- A. P. Yelisseyev, L. I. Isaenko and M. K. Starikova, J. Opt. Soc. Am. B, 2012, 29, 1430 CrossRef CAS.
- Y. H. Li, H. P. Wu, B. B. Zhang, Z. H. Yang, G. P. Han and S. L. Pan, Inorg. Chem., 2018, 57, 9376–9384 CrossRef CAS PubMed.
-
(a) O. Mulamba and M. Pantoya, J. Nanopart. Res., 2014, 16, 2310 CrossRef;
(b) H. P. Wu, H. W. Yu, W. G. Zhang, J. Cantwell, K. R. Poeppelmeier, S. L. Pan and P. S. Halasyamani, Cryst. Growth Des., 2017, 17, 4405–4412 CrossRef CAS.
-
(a) U. S. Alaan, P. Shafer, A. T. N'Diaye, E. Arenholz and Y. Suzuki, Appl. Phys. Lett., 2016, 108, 042106 CrossRef;
(b) H. P. R. Frederikse and G. A. Candela, Phys. Rev., 1966, 147, 583–584 CrossRef CAS;
(c) J. M. D. Coey, M. Venkatesan and P. Stamenov, J. Phys.: Condens. Matter, 2016, 28, 485001 CrossRef CAS PubMed.
-
(a) M. K. Y. Chan and G. Ceder, Phys. Rev. Lett., 2010, 105, 196403 CrossRef CAS PubMed;
(b) P. J. Hasnip, K. Refson, M. I. J. Probert, J. R. Yates, S. J. Clark and C. J. Pickard, Philos. Trans. R. Soc., A, 2014, 372, 20130270 CrossRef PubMed.
Footnote |
† Electronic supplementary information (ESI) available: The final refined atomic positions, isotropic thermal parameters table and BVS; the selected bond distances and bond angles; experimental and calculated powder X-ray diffraction patterns, comparison of structures, TG-DSC curves, IR spectra, calculated birefringence and the contribution of the functional groups to birefringence; the arrangements diagrammatic drawing of lone pair electrons. CCDC 2014017, 2014302 and 2042263 for SrTi(IO3)6·2H2O, (H3O)2Ti(IO3)6 and SrSn(IO3)6, respectively. For ESI and crystallographic data in CIF or other electronic format see DOI: 10.1039/d0ra10726c |
|
This journal is © The Royal Society of Chemistry 2021 |
Click here to see how this site uses Cookies. View our privacy policy here.