DOI:
10.1039/D0RA10436A
(Paper)
RSC Adv., 2021,
11, 3134-3142
A more sustainable isothiocyanate synthesis by amine catalyzed sulfurization of isocyanides with elemental sulfur†
Received
11th December 2020
, Accepted 7th January 2021
First published on 14th January 2021
Abstract
Isothiocyanates (ITCs) are typically prepared using amines and highly toxic reagents such as thiophosgene, its derivatives, or CS2. In this work, an investigation of a multicomponent reaction (MCR) using isocyanides, elemental sulfur and amines revealed that isocyanides can be converted to isothiocyanates using sulfur and catalytic amounts of amine bases, especially DBU (down to 2 mol%). This new catalytic reaction was optimized in terms of sustainability, especially considering benign solvents such as Cyrene™ or γ-butyrolactone (GBL) under moderate heating (40 °C). Purification by column chromatography was further optimized to generate less waste by maintaining high purity of the product. Thus, E-factors as low as 0.989 were achieved and the versatility of this straightforward procedure was shown by converting 20 different isocyanides under catalytic conditions, while obtaining moderate to high yields (34–95%).
Introduction
Isothiocyanates (ITCs) are widely used in heterocycle1–10 and thiourea synthesis11–13 as well as for medicinal and biochemistry applications (e.g. in the Edman degradation14,15 for amino acid sequencing of peptides or as electrophiles in bioconjugates).16–23 Several representatives of this group are known to exhibit biological activity,24 e.g. anti-cancer,25–33 anti-inflammatory,34–38 anti-microbial,39 antibiotic,39 antibacterial,40 fungicidal41 or insecticidal activity.42,43 Commonly, they are synthesized by using thionyl transfer agents (thiophosgene, its derivatives,2,44–48 or, more recently, fluorinated agents)49–54 or via the formation of dithiocarbamate salts with carbon disulfide under basic conditions, followed by desulfurization (Scheme 1a).44,55–57 These approaches are usually chosen due to the easy access of the respective amines, which can be transferred quickly to the ITC in one or two steps. However, these reagents are highly toxic (thiophosgene and carbon disulfide, the latter additionally being volatile) and their synthesis typically involves noxious compounds (i.e. considering the synthesis of thiophosgene, perchloromethyl mercaptan,58 chlorine and carbon disulfide are used).59 Moreover, ITCs are in general noxious compounds (GHS05 and GHS08) and must be handled with care.
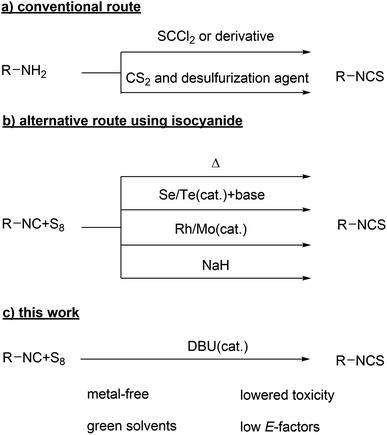 |
| Scheme 1 Overview of reported synthesis protocols towards isothiocyanates. | |
As chemists, we should avoid such problematic functional groups and their synthesis whenever possible, but we cannot stop using these important functional groups completely. Thus, applying in situ approaches to minimize their risks is a typical strategy, but only applicable if such compounds can be used as intermediates. Here, we focused our work on a more sustainable synthesis route toward ITCs by considerably reducing the toxicological impact of the used synthesis reagents as well as minimizing the amount of waste (E-factor), two very important aspects in terms of overall sustainability. The above described inherent toxicity of the ITC functional group does of course remain unchanged and thus, these compounds have to be handled with caution. Besides the aforementioned synthesis starting from amines, several other functional groups2,44,45,60–62 can be converted to ITCs, of which isocyanides, commonly used in multicomponent reactions (MCRs),63–65 are particularly interesting as they are generally less toxic.63 The sulfurization of isocyanides with elemental sulfur was previously reported in literature for a few compounds,66,67 however this reaction was reported to proceed only at high temperatures (80 °C and 130 °C)66,67 and thus, several catalysts have since been reported to allow milder reaction conditions (Scheme 1b). Most commonly, selenium68–70 (or tellurium)71,72 in the presence of an amine base efficiently catalyzes the formation of ITCs even at room temperature. Employing several metal catalysts (i.e. molybdenum and rhodium based) led to excellent yields as well.73,74 However, selenium and tellurium are highly toxic, and thus we sought to investigate the use of sulfur as suitable sulfurization agent and simultaneously avoiding the use of toxic metal catalyst in this approach. The use of elemental sulfur is highly desired considering the current storage issues of millions of tons of sulfur produced as waste product from the petroleum industry (almost 80 million metric tons in 2019)75 due to safety reasons76 as well as acidification of soil and water.77 Furthermore of importance for the herein described new approach, we recently reported a more sustainable synthesis of non-sterically demanding aliphatic isocyanides,78 avoiding toxic compounds such as phosgene, its derivatives or POCl3. This reaction relies on p-toluenesulfonyl chloride, which is less toxic than otherwise required reagents and a waste product of the industrial saccharin synthesis.79,80 With this in hand, we had all tools we need to pursue the synthesis of ITCs via isocyanides in a more sustainable fashion (Scheme 1c).
In 2019, Ábrányi-Balogh and co-workers reported on a mechanistic investigation of a MCR between isocyanides, elemental sulfur and alcohols or thiols under basic conditions (sodium hydride, 2.00 eq.), obtaining (di)thio carbamates. They showed that ITCs are intermediates in this reaction, which could be isolated in excellent yields for one compound (2,6-dimethylphenyl isothiocyanate).81 In an earlier work from Al-Mourabit and co-workers, a similar MCR was reported82 using primary or secondary amines instead of alcohols, leading to thioureas.
This strategy was later on applied for the synthesis of organocatalysts.83 In this previous work, ITCs were not confirmed as intermediates, but hypothesized as one possibility of three postulated pathways. In this reaction, no external base was required, as an excess of amine (1.20 eq.) was suggested to catalyze the reaction sufficiently. The then postulated mechanistic pathway, involving the ITC intermediate for this MCR (Scheme 2), entails the nucleophilic attack of an amine to the sulfur ring, thus forming polysulfur chains, which then attack the isocyanide to yield an ITCs after eliminating a Sx−1 sulfur chain. Since primary or secondary amines were used, the possibly present ITC intermediate was immediately converted to the respective thiourea. Thus, we hypothesized that the use of tertiary amines would prevent further reaction of the ITC and thus allow a catalytic synthesis of ITCs.
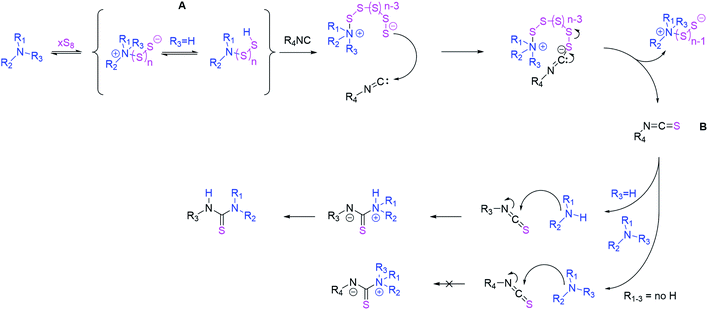 |
| Scheme 2 Possible mechanistic pathway of the MCR between isocyanide (black), elemental sulfur (purple) and an amine (blue), whereby the amine acts as reactant as well as catalyst to form polysulfur chains A. Applying primary or secondary amines leads to thioureas, while tertiary amines lead to isothiocyanates B. | |
We thus started investigating the use of tertiary amines as organocatalysts for the sulfurization of isocyanides to yield ITCs in a more sustainable fashion. We further optimized the reaction conditions (temperature, solvent, stoichiometry) as well as the purification (normally flash column chromatography) to minimize the resulting E-factor by maintaining high purity.
Results and discussion
Mechanistic investigation of sulfur activation
We initially evaluated the reactivity of various amines in the reaction of a model isocyanide (n-dodecyl isocyanide 1a) with elemental sulfur by reacting them in stoichiometric amounts in a suspension of DMSO, while monitoring the formation of the respective ITC 3a (Table 1, entries 1–6). In every case, the instant formation of the polysulfur chains was visible, since the reaction mixture turned dark brown (same color change was observed in literature)84,85 as soon as the base was added. As the reaction proceeded, the consumption of the suspended sulfur was also visually observed. GC monitoring of the test reactions revealed that equimolar amounts 1,8-diazabicyclo[5.4.0]undec-7-en (DBU) or 1,5,7-triazabicyclo[4.4.0]dec-5-en (TBD) led to quantitative conversion after 2 hours of reaction time (Table 1, entries 3 and 6, respectively). Nucleophilic aromatic amines, like N,N-dimethylamino pyridine (DMAP) and N-methyl imidazole (NMI), were less efficient, both resulting in 38% of conversion, which was attributed to their lower basicity. In general, steric hindrance was deemed less important for the formation of the polysulfur chains, since the conversion of isocyanide 1a observed using the sterically hindered triethylamine (TEA) and 1,4-diazabicyclo[2.2.2]octane (DABCO) were also high, 76% and 84%, respectively.
Table 1 GC-screening of various amine bases (bold) for the activation of elemental sulfur, leading to the formation of n-dodecylisothiocyanate 3a

|
Entry |
Amine (pKa-value in H2O) |
Eq. of amine base |
Reaction time/h |
Conversiona/% |
1 mmol of n-dodecyl isocyanide 1a was reacted with elemental sulfur (2.00 eq. of sulfur atoms) and the respective amount of amine in 1 mL of DMSO at room temperature (r.t.). GC samples were taken after the respective time and conversions were calculated using biphenyl (0.25 eq.) as internal standard (IS). |
1 |
DMAP (9.2)86 |
1.00 |
2 |
38 |
2 |
NMI (7.1)87 |
1.00 |
2 |
38 |
3 |
DBU (11.5)86 |
1.00 |
2 |
99 |
4 |
DABCO (8.9)86 |
1.00 |
2 |
84 |
5 |
TEA (10.7)88 |
1.00 |
2 |
76 |
6 |
TBD (14.5)89 |
1.00 |
2 |
100 |
7 |
DBU |
0.10 |
2 |
57 |
8 |
DBU |
0.10 |
22 |
67 |
9 |
TBD |
0.10 |
2 |
36 |
10 |
TBD |
0.10 |
22 |
69 |
Taking into account the basicity of the evaluated amines (Table 1, entries 1–6), it was noted that the most basic amine (TBD) led to the highest conversion, which is consistent with previous reports in which elemental sulfur was activated by strong bases like NaH81 or K2CO3.85 However, DABCO, exhibiting the second lowest pKa-value, also showed good performance. Assuming both tertiary amine groups of DABCO are able to activate sulfur in this reaction, the amount of active groups present is doubled compared to the other bases, which might lead to higher conversions in this case. These results show that not only basicity defines the reactivity of the applied amine base, albeit a certain degree of basicity is certainly crucial to activate elemental sulfur.
In a second step, we sought to apply the two best performing amines, i.e. DBU and TBD, in a sub-stoichiometric/catalytic amount, as no side products were detected, while monitoring the reaction with GC (Table 1, entries 7–10). As a highly remarkable result, 10 mol% of DBU or TBD were able to convert isocyanide 1a after 22 hours at room temperature with high conversion (67% or 69%, respectively) and the corresponding ITC 3a was obtained. DBU showed better results, i.e. 57% conversion after only two hours, compared to 36% conversion for TBD after the same time.
Optimization of the reaction conditions
Having identified two amine bases (TBD and DBU) that can be applied in substoichiometric amounts, we optimized the reaction conditions addressing as many of the Twelve Principles of Green Chemistry90 as possible and further minimized the E-factor91 (not yet taking the purification steps into account). Initially, when screening the reaction temperature, higher conversions were obtained at higher temperatures, but in the context of sustainability a less energy-consuming procedure is desired and we thus considered moderate temperatures (40 °C) for further experiments. Next, we altered the solvents focusing on greener alternatives for commonly used solvents according to solvent selection guides92–94 (for the complete screening, please see the ESI†). A clear trend was observed, i.e. polar aprotic solvents like DMSO, GBL and Cyrene™ (dihydrolevoglucosenone) favored product formation, probably due to their ability to dissolve and stabilize polysulfur chains. Amongst them, Cyrene™ allowed full conversion of isocyanide 1a after two hours reaction time, while GBL and DMSO also led to high conversions (92 and 85%, respectively). Both GBL and Cyrene™ are greener alternatives if compared to DMSO and are synthesized from renewable resources (butane-1,4-diol95 and cellulose,96 respectively), thus decreasing the overall environmental impact. However, more volatile compounds would allow an easier removal and recycling of the solvent. Among the more volatile and sustainable solvents, only acetone was able to achieve good, yet lower conversions (69%). The contribution of the solvent for the proceeding of this reaction was further underpinned by testing the reaction in absence of any solvent, resulting in a low conversion of ∼8% after 30 minutes (Table 2, entry 1).
Table 2 Optimization of reaction conditions (concentration) for the sulfurization of isocyanide 1a via GC-screening to decrease the E-factor

|
Entry |
Solvent |
Concentrationa/M |
Conversionb/% |
E-Factorc |
Concentration of isocyanide 1a in the solvent. 1.00 mmol of n-dodecylisocyanide 1 was reacted with elemental sulfur (2.00 eq. of sulfur atoms) and TBD (10 mol%) in the Cyrene™ at 40 °C. GC-samples were taken after 30 minutes of reaction time and conversions were calculated using biphenyl (0.25 eq.) as IS. E-Factor was calculated assuming conversion equals the yield, as no side-reaction were observed, not taking the purification steps into account. |
1 |
— |
— |
6.7 |
16.9 |
2 |
Cyrene™ |
1.0 |
85 |
5.71 |
3 |
Cyrene™ |
2.0 |
99 |
2.95 |
3 |
Cyrene™ |
4.0 |
100 |
1.58 |
4 |
Cyrene™ |
6.0 |
100 |
1.12 |
To decrease both reaction time and waste, we increased the concentration of isocyanide 1a up to 6 M in Cyrene™ (Table 2, entries 2–4), which was the upper limit due to practical reasons (since elemental sulfur was only partially dissolved at the beginning of the reaction, a certain amount of solvent was needed to facilitate stirring). Thus, the E-factor could be reduced to 1.12 maintaining full conversion after 30 minutes (Table 2, entry 4). Furthermore, we decreased the excess of elemental sulfur to 1.12 eq. of sulfur atoms, while maintaining high conversion (98% after 30 minutes). Since 10 mol% of catalyst performed excellent under the optimized conditions, we then evaluated lower catalyst loadings of DBU (Table 3), since (i) higher conversions in less amount of time were obtained in prior tests and (ii) the stirring of the reaction is facilitated by DBU being a liquid.
Table 3 Optimization of reaction conditions (catalyst loading of DBU, bold) for the sulfurization of isocyanide 1a via GC-screening

|
Entry |
Catalysts loading/mol% |
Concentrationa/M |
Reaction time/h |
Conversionb/% |
Concentration of isocyanide 1a in Cyrene™. 1.00 mmol of n-dodecylisocyanide 1 was reacted with elemental sulfur (1.12 eq. of sulfur atoms) and DBU (respective amount) in Cyrene™ at 40 °C. GC-samples were taken after the respective time and conversions were calculated using biphenyl (0.25 eq.) as IS. |
1 |
5 |
2.0 |
0.5 |
59 |
2 |
5 |
2.0 |
4 |
100 |
3 |
2 |
2.0 |
0.5 |
29 |
4 |
2 |
2.0 |
20 |
99 |
5 |
2 |
6.0 |
4 |
100 |
6 |
1 |
6.0 |
4 |
78 |
7 |
1 |
6.0 |
20 |
96 |
We note however that the toxicity of DBU is higher compared to TBD (DBU is labeled with GHS05, while TBD is labelled as GHS07). As a result of the high concentration of isocyanide 1a in the solvent (6 M), the catalyst loading of DBU could be further reduced to 1 mol% while still achieving nearly quantitative conversion after prolonged reaction times of 20 hours. Using 2 mol% of DBU at the same concentration of 1a resulted in complete conversion after only four hours. For more diluted reaction mixtures (c(isocyanide) = 2 M), the amount of DBU had to be increased to 5 mol% to obtain full conversion after four hours, which is still a low catalyst-loading. The final optimized reaction conditions are depicted in Scheme 3. We note that when a liquid isocyanide was used, the conditions were 2 mol% of DBU in 6 M Cyrene™ solution, while for solid isocyanides 5 mol% of DBU in 2 M Cyrene™ was used, predominantly to facilitate stirring.
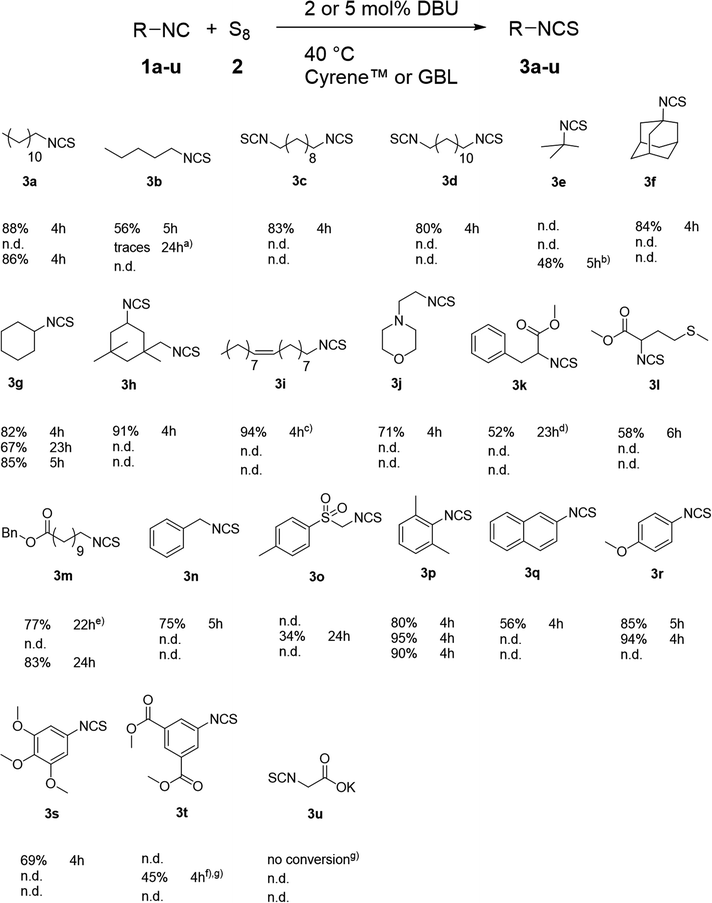 |
| Scheme 3 Herein synthesized ITCs 3a–3t using the new procedure in a 2.5 mmol scale using elemental sulfur (1.12 eq. of sulfur atoms). If the isocyanide was a liquid at 40 °C, 2 mol% DBU and 417 μL solvent was used, if it was a solid, 5 mol% DBU and 1.25 mL solvent were used. For each compound, the yield and the reaction time are displayed. The first line corresponds to the reaction using Cyrene™ as solvent, the second line using GBL. The third line displays the reaction in Cyrene™ in a 15.5 mmol scale. GC-purities were in general >95% (see ESI†). (a) GC-purity was not determined (n.d.); (b) pressure vial was used; (c) GC-purity of the starting material 1i was 80% and of ITC 3i 83%; (d) 2.31 mmol isocyanide 1k was used; (e) 5 mol% DBU was used; (f) 2.00 mmol isocyanide 1t was used; (g) starting material was not/less soluble. | |
Evaluating the scope of the sulfurization of isocyanides
Having the optimized reaction conditions in hand, we synthesized several mono- and diisothiocyanates to evaluate the substrate scope of our new procedure (Scheme 3). Aliphatic, benzylic as well as aromatic isocyanides were successfully converted to the respective isothiocyanates. Comparing aliphatic isothiocyanates, steric hindrance was found to have minor impact on the yield, which varied from 34 to 94%. In the case of aromatic isothiocyanates, electron-rich and deficient as well as condensed aromatic isocyanides were successfully converted obtaining yields from 45 to 95%, whereby no trend related to electron density was observed. In addition, the reaction had a high degree of chemoselectivity, since several functional groups (double bond (internal), ether (aliphatic and aromatic), thioether (aliphatic), tertiary amine, p-toluenesulfonyl, ester) were tolerated. However, the carboxylic acid salt 3u (85% purity) could not be converted, probably due to solubility issues. Considering the tertiary amine of 2-morpholinoethyl isocyanide 3j, an (auto-)catalytic effect might be anticipated, however it was ruled out by performing the reaction in absence of DBU (no yield after 3 hours). Since some isocyanide compounds are volatile (3e), a pressure tube was crucial to obtain the respective product. Besides Cyrene™, GBL performed very similar as solvent in this reaction, converting isocyanide 1a to ITC 3a and thus, we hypothesized that GBL could be a more suitable solvent for some ITCs. Therefore, we tested six substrates and as a result, better yields were obtained for aromatic ITCs 3p and 3r, while the yield of ITC 3g was lower. Synthesis of ITC 3b in GBL only resulted in minor traces of the product. Nevertheless, in the case of 3o and 3t, Cyrene™ exhibited similar retention time on silica gel and thus GBL was found to be more feasible for this reaction in the light of fast and more sustainable purification. These results showed that GBL can be superior to Cyrene™ in some cases, depending on the applied substrate.
Purification of all herein reported compounds was performed by a modified flash column chromatography to reduce waste (vide infra). Furthermore, multigram scale reactions (15.5 mmol) were easily performed, generally resulting in similar or higher yields (i.e. 3a, 3g, 3m and 3p in Scheme 3). This increased yield was likely due to small amounts of sulfur sticking to the wall of the reaction flask, which is a negligible effect for larger scale reactions. All herein newly synthesized compounds were fully characterized by proton and carbon NMR-spectroscopy, IR-spectroscopy and high-resolution mass spectrometry. Purities of all synthesized ITCs were determined by gas chromatography and, for several substrates, elemental analysis (vide infra). Furthermore, E-factors were calculated for every ITC (see the ESI† for characterization, purity and E-factor data).
Improving sustainability of purification
Purification of ITCs is commonly performed by (flash) column chromatography, thus adding a considerable amount of waste and resulting in poor E-factors. Therefore, we sought to optimize the purification procedure as well. Initial attempts to avoid column chromatography by applying several washing steps of the organic layers led to lower purities. Furthermore, excessive washing with aqueous layers was needed and thus, column chromatography was the purification method of choice, resulting in high purity and less waste (GC-purities in general over 95%, see ESI†). Ultimately, a dry loaded small column (5–8 cm, see Fig. S1 in ESI†), similar to a silica plug, was applied as sole purification step directly at the end of the reaction. The amount of used silica, solvent and time was therefore minimized. Residual elemental sulfur was thus also removed, as confirmed by elemental analysis (elemental analysis was performed for several non-volatile ITCs, see ESI†).
To further underpin the high purity of this less waste producing purification, we performed a polyaddition reaction of diisothiocyanates 3c, 3d and 3h with renewable 1,5-diamino pentane (98% purity) in an equimolar stoichiometry. Since high purities of the monomers, here of the ITCs, are crucial to obtain high molecular weights via step-growth polymerization, a successfully polymerization is a good indication of the suitability of this more sustainable purification (Scheme 4). In all three cases, high molecular weights (Mn of P1–3 were 18.6 kDa, 55.1 kDa and 58.1 kDa, respectively, for SEC-graphs see ESI†) were obtained, proving that this optimized purification step resulted in excellent purities. However, for compounds 3o and 3t, applying a classic common column chromatography could not be avoided, since the retention time of the substrate and the solvent were similar to the solvent (vide supra).
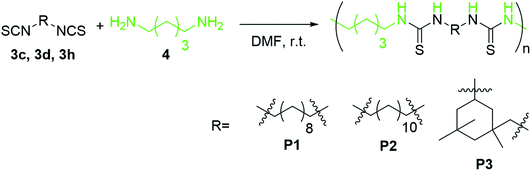 |
| Scheme 4 Synthesis of polythioureas P1–3 with 1,5-diaminopentane 4 (green) and herein synthesized ITCs 3c, 3d and 3h (black). | |
Overall sustainability
Combining the herein reported DBU catalyzed sulfurization of isocyanides with elemental sulfur and the optimized purification step, very low E-factors were obtained and the toxicity of the overall reaction was minimized by avoiding highly toxic starting materials. This is underpinned by comparison of the synthesis of compound 3q starting from the respective isocyanide 1q with several literature known procedures (Table 4). For a better comparison of the synthesis protocols, we calculated the E-factor with and without the amount of solvent used (E-factor calculated without respect to solvent was herein called synthetic E-factor), since some of these procedures would decrease their E-factors drastically by conducting the reaction in higher concentrations. Obviously, the use of a catalyzed reaction, compared to reactions using stoichiometric amounts of reagents, resulted in lower E-factors. Using the herein reported protocol with Cyrene™ resulted in the lowest yield of 80%, for which the synthetic E-factor was comparable to that of procedures using transition metal catalysts offering higher yields (RhH(Ph3)4 and Mo(O)(S2CNEt2)2 obtaining 96 and 91%, respectively). On the other hand, the lowest synthetic E-factor of 0.129 was obtained using our procedure with GBL as solvent, obtaining an excellent yield as well. Taking the solvent into account, the E-factor of our new protocol for the synthesis of 3q remained very low with 3.84 and 5.30 for GBL and Cyrene™, respectively. Only the procedure reported by Bargon using a molybdenum catalyst resulted in a lower E-factor of 2.10 due to the use of acetone. Nevertheless, even lower E-factors, down to 0.989 (for 3m), were achieved with our method converting liquid isocyanides (for an overview of all E-factors see ESI†). In addition, this new procedure does not require inert atmosphere, while only moderate energy consumption is needed (40 °C for ca. 4 hours). Comparing the purification methods, we minimized the amount of waste produced by purification using an optimized flash column chromatography step as sole purification step. Furthermore, this procedure is metal-free and the overall toxicity of the reaction is minimized by using catalytic amounts of DBU. Nevertheless, the reader has to be reminded that ITCs intrinsically bear a certain degree of toxicity, which must be addressed by applying adequate safety measures.
Table 4 Comparison of sulfurization approaches of isocyanide 3q considering E-factors, energy consumption and purification methods
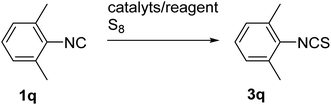
|
Catalyst/reactant |
Conditions |
Yield/% |
E-Factora (syn.) |
E-Factorb |
Purification |
Ref. |
Synthetic E-factor involving reactants, catalysts and remaining starting material. E-Factor taking the used reaction solvent into account. GBL was used as solvent. Cyrene™ was used as solvent. Reference mentioned that high purity of isocyanide was very important. cc = column chromatography. |
5 mol% DBU |
40 °C, 4 h |
95 |
0.129 |
3.84 |
(1) Optimized flash cc |
This workc |
5 mol% DBU |
40 °C, 4 h |
80 |
0.349 |
5.30 |
(1) Optimized flash cc |
This workd |
2.00 eq. NaH |
40 °C, 2 h, Ar-atm |
85 |
0.982 |
20.2 |
(1) Dilution with EA, (2) filtration, (3) flash cc |
81 |
1 mol% RhH(Ph3)4 |
56 °C, 2.5 h, Ar-atm |
96e |
0.353 |
10.4 |
(1) Flash cc |
74 |
1 mol% Rh(acac)(CH2 = CH2)2 |
56 °C, 2.5 h, Ar-atm |
91e |
0.412 |
11.0 |
(1) Flash cc |
74 |
1 mol% Mo(O)(S2CNEt2)2 |
56 °C, 72 h, Ar-atm |
91 |
0.342 |
2.10 |
(1) Dilution with petroleum ether, (2) filtration, (3) distillation |
73 |
5 mol% Se, 2.40 eq. TEA |
66 °C, 1 h |
74 |
2.28 |
17.0 |
(1) Filtration, (2) distillation |
70 |
Conclusion
Mechanistic investigations of a MCR between isocyanide, amines and elemental sulfur, leading to thioureas, confirmed isothiocyanates as intermediates, which could be trapped herein by using tertiary amines. As a result, a more sustainable synthesis protocol for the sulfurization of isocyanides towards isothiocyanates was established using DBU as greener catalyst in low loading down to 2 mol% and the green solvents Cyrene™ and GBL. E-Factors down to 0.989 were achieved while toxicity, energy and time consumption of the reaction was minimized obtaining moderate to excellent yields. The sulfurization method tolerates several functional groups and is easily applicable yielding the desired product in high purity. We hope that this procedure can pave the way for greener syntheses of isothiocyanates starting from the respective isocyanides and further the formamides and amines.
Experimental
General synthesis of isothiocyanates (solid isocyanide)
The corresponding solid isocyanide (1.00 eq., mp >40 °C) was dissolved in dihydrolevoglucosenone (Cyrene™; c(isocyanide) = 2 M) and elemental sulfur (1.12 eq. of sulfur atoms) was added. After addition of 5 mol% of 1,8-diazabicyclo[5.4.0] undec-7-ene (DBU) the reaction mixture was stirred for 4 hours at 40 °C. After completion of the reaction, purification by optimized flash column chromatography applying a dry loaded small column (typically height of silica loading around 5–8 cm, width was 3 cm, see Fig. S1 in ESI†) and a mixture of cyclohexane and ethyl acetate yielded the pure product.
General synthesis of isothiocyanates (liquid isocyanide)
The corresponding liquid isocyanide (1.00 eq., mp ≤40 °C) was dissolved in dihydrolevoglucosenone (Cyrene™; c(isocyanide) = 6 M) and elemental sulfur (1.12 eq. of sulfur atoms) was added. After addition of 2 mol% of 1,8-diazabicyclo[5.4.0]undec-7-ene (DBU), the reaction mixture was stirred for 4 hours at 40 °C. After completion of the reaction, purification by optimized flash column chromatography applying a dry loaded small column (typically height of silica loading around 5–8 cm width was 3 cm, see Fig. S1 in ESI†) and a mixture of cyclohexane and ethyl acetate yielded the pure product.
Conflicts of interest
There are no conflicts of interest to declare.
Notes and references
- A. K. Mukerjee and R. Ashare, Chem. Rev., 1991, 91, 1–24 CrossRef CAS.
- S. Sharma, Sulfur Rep., 1989, 8, 327–454 CrossRef CAS.
- H. Stephensen and F. Zaragoza, J. Org. Chem., 1997, 62, 6096–6097 CrossRef CAS.
- J.-W. Qiu, X.-G. Zhang, R.-Y. Tang, P. Zhong and J.-H. Li, Adv. Synth. Catal., 2009, 351, 2319–2323 CrossRef CAS.
- R. Yella, N. Khatun, S. K. Rout and B. K. Patel, Org. Biomol. Chem., 2011, 9, 3235–3245 RSC.
- S. Guin, S. K. Rout, A. Gogoi, S. Nandi, K. K. Ghara and B. K. Patel, Adv. Synth. Catal., 2012, 354, 2757–2770 CrossRef CAS.
- J.-J. Chu, B.-L. Hu, Z.-Y. Liao and X.-G. Zhang, J. Org. Chem., 2016, 81, 8647–8652 CrossRef CAS.
- Y. He, J. Li, S. Luo, J. Huang and Q. Zhu, Chem. Commun., 2016, 52, 8444–8447 RSC.
- S. Khan and C. M. R. Volla, Chem.–Eur. J., 2017, 23, 12462–12466 CrossRef CAS.
- M. Feng, P. Yang, G. Yang, W. Chen and Z. Chai, J. Org. Chem., 2018, 83, 174–184 CrossRef CAS.
- D. C. Schroeder, Chem. Rev., 1955, 55, 181–228 CrossRef CAS.
- A. Shakeel, A. A. Altaf, A. M. Qureshi and A. Badshah, J. Drug Des. Med. Chem., 2016, 2, 10–20 Search PubMed.
- F. Steppeler, D. Iwan, E. Wojaczyńska and J. Wojaczyński, Molecules, 2020, 25, 401 CrossRef.
- P. Edman, Acta Chem. Scand., 1950, 4, 283–293 CrossRef CAS.
- P. Edman and G. Begg, Eur. J. Biochem., 1967, 1, 80–91 CrossRef CAS.
- G. Barbarella, Chem.–Eur. J., 2002, 8, 5072–5077 CrossRef CAS.
- D. Wang, S. Liu, B. J. Trummer, C. Deng and A. Wang, Nat. Biotechnol., 2002, 20, 275–281 CrossRef CAS.
- S. Dong and M. Roman, J. Am. Chem. Soc., 2007, 129, 13810–13811 CrossRef CAS.
- S. Heckl, A. Sturzu, M. Regenbogen, A. Beck, G. Feil, A. Gharabaghi and H. Echner, Med. Chem., 2008, 4, 348–354 CrossRef CAS.
- F. Meng, B. N. Manjula, P. K. Smith and S. A. Acharya, Bioconjugate Chem., 2008, 19, 1352–1360 CrossRef CAS.
- E.-M. Kim, H.-J. Jeong, I.-K. Park, C.-S. Cho, C.-G. Kim and H.-S. Bom, J. Nucl. Med., 2005, 46, 141–145 CAS.
- Y. S. Zhang, R. H. Kolm, B. Mannervik and P. Talalay, Biochem. Biophys. Res. Commun., 1995, 206, 748–755 CrossRef CAS.
- X. Guo and M. E. Meyerhoff, Appl. Biochem. Biotechnol., 1997, 68, 41–56 CrossRef CAS.
- K. Srivastava, A. Bhatt, N. Singh, R. Khare, R. Shukla, D. Chaturvedi and R. Kant, Chem. Biol. Interface, 2020, 10, 34–50 CAS.
- E.-S. Hwang and E. H. Jeffery, Food Chem. Toxicol., 2003, 41, 1817–1825 CrossRef CAS.
- Y. Zhang, L. Tang and V. Gonzalez, Mol. Cancer Ther., 2003, 2, 1045–1052 CAS.
- A. P. Lawson, M. J. C. Long, R. T. Coffey, Y. Qian, E. Weerapana, F. El Oualid and L. Hedstrom, Cancer Res., 2015, 75, 5130–5142 CrossRef CAS.
- D. Xiao, V. Vogel and S. V. Singh, Mol. Cancer Ther., 2006, 5, 2931–2945 CrossRef CAS.
- A. Melchini, P. W. Needs, R. F. Mithen and M. H. Traka, J. Med. Chem., 2012, 55, 9682–9692 CrossRef CAS.
- IARC Handbooks of Cancer Prevention, Cruciferous Vegetables, Isothiocyanates and Indoles, IARC Press, Lyon, 9th edn, 2004 Search PubMed.
- X. Wu, Y. Zhu, H. Yan, B. Liu, Y. Li, Q. Zhou and K. Xu, BMC Cancer, 2010, 10, 269 CrossRef.
- C. Ioannides and N. Konsue, Drug Metab. Rev., 2015, 47, 356–373 CrossRef CAS.
- M. Traka and R. Mithen, Phytochem. Rev., 2009, 8, 269–282 CrossRef CAS.
- S. Giacoppo, M. Galuppo, G. R. De Nicola, R. Iori, P. Bramanti and E. Mazzon, Bioorg. Med. Chem., 2015, 23, 80–88 CrossRef CAS.
- C. Waterman, D. M. Cheng, P. Rojas-Silva, A. Poulev, J. Dreifus, M. A. Lila and I. Raskin, Phytochemistry, 2014, 103, 114–122 CrossRef CAS.
- M. Galuppo, S. Giacoppo, G. R. De Nicola, R. Iori, M. Navarra, G. E. Lombardo, P. Bramanti and E. Mazzon, Fitoterapia, 2014, 95, 160–174 CrossRef CAS.
- T. Uto, D.-X. Hou, O. Morinaga and Y. Shoyama, Adv. Pharmacol. Sci., 2012, 2012, 614046 Search PubMed.
- J. V. Cross, J. M. Rady, F. W. Foss Jr, C. E. Lyons, T. L. Macdonald and D. J. Templeton, Biochem. J., 2009, 423, 315–321 CrossRef CAS.
- D. Li, Y. Shu, P. Li, W. Zhang, H. Ni and Y. Cao, Med. Chem. Res., 2013, 22, 3119–3125 CrossRef CAS.
- C.-M. Lin, J. F. Preston III and C.-I. Wei, J. Food Prot., 2000, 63, 727–734 CrossRef CAS.
- H. S. Mayton, C. Olivier, S. F. Vaughn and R. Loria, Phytopathology, 1996, 86, 267–271 CrossRef CAS.
- V. Borek, L. R. Elberson, J. P. McCaffrey and M. J. Morra, J. Econ. Entomol., 1997, 90, 109–112 CrossRef CAS.
- L. Williams, M. J. Morra, P. D. Brown and J. P. McCaffrey, J. Chem. Ecol., 1993, 19, 1033–1046 CrossRef CAS.
- K. Eschliman and S. H. Bossmann, Synthesis, 2019, 51, 1746–1752 CrossRef CAS.
- Z. Fu, W. Yuan, N. Chen, Z. Yang and J. Xu, Green Chem., 2018, 20, 4484–4491 RSC.
- S. Kim and K. Y. Yi, J. Org. Chem., 1986, 51, 2613–2615 CrossRef CAS.
- C. Larsen and D. N. Harpp, J. Org. Chem., 1981, 46, 2465–2466 CrossRef CAS.
- C. Larsen, K. Steliou and D. N. Harpp, J. Org. Chem., 1978, 43, 337–339 CrossRef CAS.
- Y.-Y. Liao, J.-C. Deng, Y.-P. Ke, X.-L. Zhong, L. Xu, R.-Y. Tang and W. Zheng, Chem. Commun., 2017, 53, 6073–6076 RSC.
- W. Feng and X.-G. Zhang, Chem. Commun., 2019, 55, 1144–1147 RSC.
- J. Yu, J.-H. Lin and J.-C. Xiao, Angew. Chem., Int. Ed., 2017, 56, 16669–16673 CrossRef CAS.
- T. Scattolin, A. Klein and F. Schoenebeck, Org. Lett., 2017, 19, 1831–1833 CrossRef CAS.
- J. Wei, S. Liang, L. Jiang and W. Yi, J. Org. Chem., 2020, 85, 12374–12381 CrossRef.
- L. Zhen, H. Fan, X. Wang and L. Jiang, Org. Lett., 2019, 21, 2106–2110 CrossRef CAS.
- S. B. Tsogoeva, D. A. Yalalov, M. J. Hateley, C. Weckbecker and K. Huthmacher, Eur. J. Org. Chem., 2005, 2005, 4995–5000 CrossRef.
- P. Molina, M. Alajarin and A. Arques, Synthesis, 1982, 1982, 596–597 CrossRef.
- Ł. Janczewski, A. Gajda and T. Gajda, Eur. J. Org. Chem., 2019, 2019, 2528–2532 CrossRef.
- C. H. Hoyt and K. Jonas, Manufacture of perchloromethyl mercaptan, US3014071A, 1961.
- W. Autenrieth and H. Hefner, Ber. Dtsch. Chem. Ges., 1925, 58, 2151–2156 CrossRef.
- Z. Shusheng, Z. Tianrong, C. Kun, X. Youfeng and Y. Bo, Eur. J. Med. Chem., 2008, 43, 2778–2783 CrossRef.
- J. N. Kim, J. H. Song and E. K. Ryu, Synth. Commun., 1994, 24, 1101–1105 CrossRef CAS.
- M. Baumann and I. R. Baxendale, Beilstein J. Org. Chem., 2013, 9, 1613–1619 CrossRef.
- A. Dömling and I. Ugi, Angew. Chem., Int. Ed., 2000, 39, 3168–3210 CrossRef.
- A. Llevot, A. C. Boukis, S. Oelmann, K. Wetzel and M. A. R. Meier, Top. Curr. Chem., 2017, 375, 66 CrossRef.
- R. C. Cioc, E. Ruijter and R. V. A. Orru, Green Chem., 2014, 16, 2958–2975 RSC.
- J. H. Boyer and V. T. Ramakrishnan, J. Org. Chem., 1972, 37, 1360 CrossRef CAS.
- W. Bao, C. Chen, N. Yi, J. Jiang, Z. Zeng, W. Deng, Z. Peng and J. Xiang, Chin. J. Chem., 2017, 35, 1611–1618 CrossRef CAS.
- K. Nishikawa, T. Umezawa, M. Garson and F. Matsuda, J. Nat. Prod., 2012, 75, 2232–2235 CrossRef CAS.
- N. A. Weires, E. D. Styduhar, E. L. Baker and N. K. Garg, J. Am. Chem. Soc., 2014, 136, 14710–14713 CrossRef CAS.
- S. Fujiwara, T. Shink-Ike, N. Sonoda, M. Aoki, K. Okada, N. Miyoshi and N. Kambe, Tetrahedron Lett., 1991, 32, 3503–3506 CrossRef CAS.
- H. Mitome, N. Shirato, H. Miyaoka, Y. Yamada and R. W. M. van Soest, J. Nat. Prod., 2004, 67, 833–837 CrossRef CAS.
- S. Fujiwara, T. Shin-Ike, K. Okada, M. Aoki, N. Kambe and N. Sonoda, Tetrahedron Lett., 1992, 33, 7021–7024 CrossRef CAS.
- W. Adam, R. M. Bargon, S. G. Bosio, W. A. Schenk and D. Stalke, J. Org. Chem., 2002, 67, 7037–7041 CrossRef CAS.
- M. Arisawa, M. Ashikawa, A. Suwa and M. Yamaguchi, Tetrahedron Lett., 2005, 46, 1727–1729 CrossRef CAS.
- National Mineral Information Center, Mineral Commodity Summaries, 2020 Search PubMed.
- T. A. Rappold and K. S. Lackner, Energy, 2010, 35, 1368–1380 CrossRef CAS.
- F. Crescenzi, A. Crisari, E. D'Angel and A. Nardella, Environ. Sci. Technol., 2006, 40, 6782–6786 CrossRef CAS.
- K. A. Waibel, R. Nickisch, N. Möhl, R. Seim and M. A. R. Meier, Green Chem., 2020, 22, 933–941 RSC.
- C. Fahlberg and I. Remsen, Ber. Dtsch. Chem. Ges., 1879, 12, 469–473 CrossRef.
- D. J. Ager, D. P. Pantaleone, S. A. Henderson, A. R. Katritzky, I. Prakash and D. E. Walters, Angew. Chem., Int. Ed., 1998, 37, 1802–1817 CrossRef CAS.
- A. G. Németh, G. M. Keserű and P. Ábrányi-Balogh, Beilstein J. Org. Chem., 2019, 15, 1523–1533 CrossRef.
- T. B. Nguyen, L. Ermolenko and A. Al-Mourabit, Synthesis, 2014, 46, 3172–3179 CrossRef CAS.
- R. Nickisch, S. M. Gabrielsen and M. A. R. Meier, ChemistrySelect, 2020, 5, 11915–11920 CrossRef CAS.
- T. B. Nguyen, M. Q. Tran, L. Ermolenko and A. Al-Mourabit, Org. Lett., 2014, 16, 310–313 CrossRef CAS.
- W. Cao, F. Dai, R. Hu and B. Z. Tang, J. Am. Chem. Soc., 2020, 142, 978–986 CrossRef CAS.
- F. Ravalico, S. L. James and J. S. Vyle, Green Chem., 2011, 13, 1778–1783 RSC.
- J. A. Dean, Lange's Handbook of Chemistry, McGraw-Hill, Inc., New York, 15th edn, 1999 Search PubMed.
- I. Kaljurand, A. Kütt, L. Sooväli, T. Rodima, V. Mäemets, I. Leito and I. A. Koppel, J. Org. Chem., 2005, 70, 1019–1028 CrossRef CAS.
- K. M. K. Yu, I. Curcic, J. Gabriel, H. Morganstewart and S. C. Tsang, J. Phys. Chem. A, 2010, 114, 3863–3872 CrossRef CAS.
- P. Anastas and J. C. Warner, Green Chemistry: Theory and Practice, Oxford Univeristy Press, Oxford, 1998 Search PubMed.
- R. A. Sheldon, Chem. Ind., 1992, 903–906 CAS.
- K. Alfonsi, J. Colberg, P. J. Dunn, T. Fevig, S. Jennings, T. A. Johnson, H. P. Kleine, C. Knight, M. A. Nagy, D. A. Perry and M. Stefaniak, Green Chem., 2008, 10, 31–36 RSC.
- R. K. Henderson, C. Jimenez-Gonzalez, D. J. C. Constable, S. R. Alston, G. G. A. Inglis, G. Fisher, J. Sherwood, S. P. Binks and A. D. Curzons, Green Chem., 2011, 13, 854–862 RSC.
- D. Prat, A. Wells, J. Hayler, H. Sneddon, C. R. McElroy, S. Abou-Shehada and P. J. Dunn, Green Chem., 2016, 18, 288–296 RSC.
- W. Schwarz, J. Schossig, R. Rossbacher, R. Pinkos and H. Höke, in Ullmann's Encyclopedia of Industrial Chemistry, Wiley-VCH, Weinheim, 2019 Search PubMed.
- J. Sherwood, M. De bruyn, A. Constantinou, L. Moity, C. R. McElroy, T. J. Farmer, T. Duncan, W. Raverty, A. J. Hunt and J. H. Clark, Chem. Commun., 2014, 50, 9650–9652 RSC.
Footnote |
† Electronic supplementary information (ESI) available. See DOI: 10.1039/d0ra10436a |
|
This journal is © The Royal Society of Chemistry 2021 |
Click here to see how this site uses Cookies. View our privacy policy here.