DOI:
10.1039/D0RA09441B
(Paper)
RSC Adv., 2021,
11, 2616-2623
Enhanced ferroelectric and piezoelectric performance of (Ba0.85Ca0.15)(Zr0.1Ti0.9)O3 lead-free ceramics upon Ce and Sb co-doping
Received
6th November 2020
, Accepted 15th December 2020
First published on 12th January 2021
Abstract
(Ba0.85Ca0.15)(Zr0.1Ti0.9−xCex)O3+ySb (abbreviated as BCZTCxSy) ceramics were prepared by the conventional solid-state reaction. The effect of the co-doping of Ce and Sb on the phase structure, microstructure, dielectric, and ferroelectric and piezoelectric performances of BCZTCxSy ceramics were investigated systematically. The results indicated that the obtained ceramics are composed of coexisting rhombohedral and tetragonal phases. Significant improvements were also observed in the growth of grain, relative density, as well as electrical performance. After co-doping 0.05 mol% Ce and 0.10 mol% Sb, the sizes of crystalline grains reached the maximum. The following optimal properties were obtained: εr = 2353, tan
δ = 0.026, Tc = 109.4 °C, Pmax = 17.65 μC cm−2, Pr = 10.43 μC cm−2, Ec = 2.88 kV cm−1, Smax = 0.125%,
= 417 pm V−1, d33 = 372 pC N−1. With the increase in dopants, the diffuseness γ increased from 1.663 to 1.733, indicating a more relaxed ferroelectric characteristic. All improvements show that BCZT ceramics co-doped with Ce and Sb could be promising candidates in lead-free devices.
Introduction
Piezoelectric materials are considered as one of the essential functional materials and have been widely applied in transducers, sensors and acoustic wave devices.1–3 However, currently commercial piezoelectric materials contain a large amount of lead element, such as Pb(Zr0.3Ti0.7)O3 (PZT) and 0.67Pb(Mg1/3Nb2/3)O3–0.33PbTiO3 (PMNT), which intrinsically limited by their toxicity.4,5 Therefore, lead-free piezoelectric materials are urgently required for the advocation of environmentally-friendly concepts.
In the past decade, tremendous efforts have been devoted to fabricating lead-free ceramic systems, such as K0.5Na0.5NbO3 (KNN), Bi0.5Na0.5TiO3 (BNT), BaTiO3 (BT) and BiFeO3 (BFO).6–10 Among them, BT-based ceramics have received widespread attention due to their stable chemical properties, low preparation cost, large dielectric permittivity (εr) and low leakage current.11,12 However, in actuality, the BT-based ceramics are not the ideal piezoelectric materials, and the low piezoelectric coefficient (d33 = ∼190 pC N−1) compromises the commercialization of their applications.13,14 In order to enhance the piezoelectric properties, numerous strategies have been adopted such as sintering with new technology, modifying ceramics with dopants, or providing new ways to facilitate the poling process.13–19 The ultrahigh piezoelectric effect is generally derived from the morphotropic phase boundary (MPB) in the BT-based system.20 As BaTiO3 has rich phase transition and unit cell structure types, the modification of BT to form MPB to improve piezoelectric performance is a promising strategy.21 Ren et al. have found an MPB region by utilizing Ca2+ and Zr4+ ions to modify the BaTiO3 ceramic. (Ba0.85Ca0.15)(Zr0.1Ti0.9)(BCZT) shows fascinating piezoelectric performance (d33 ∼ 600 pC N−1), which is comparable to that of the commercial PZT ceramics. According to the results from the Landau–Devonshire phenomenological theory and ab initio calculations, the origin of the piezoelectric enhancement is the flattening of a free-energy profile.22–24
Although BCZT has not been widely applied in industry, high piezoelectric response and low cytotoxicity make it a promising system for electronic devices, biomedical applications and energy harvesters for the wearable.25,26 In order to improve the comprehensive electrical performance of BCZT ceramics, extensive efforts have been devoted to the modification of BCZT ceramics. For example, Wang et al. investigated the effects of numerous rare-earth elements (including Dy, Er, Eu, Ho, Pr and Sm) on the electrical properties of ceramics, and enhanced piezoelectric properties are varied from 475 to 521 pC N−1.27 Compared with other rare-earth elements, Ce is an attractive one that exhibits a variety of valences, which can be widely used to improve the electrical properties of ceramics. Bijalwan et al. obtained a large piezoelectric response (d33 = 501 pC N−1) and a higher Curie temperature (Tc = 108.1 °C) when 0.13 mol% Ce was doped into BCZT ceramics.28 Raziye et al. obtained the highest converse piezoelectric coefficient (
= 1189 pm V−1) in BCZT–0.05 mol% Ce.29 For ABO3-type perovskite piezoelectric materials, the spontaneous polarization mainly originates from the displacement of the B-site cations within the [BO6] octahedra.30 Therefore, it is a potential strategy to enhance the electrical performance of BCZT ceramics by utilizing Ce4+ ions to replace B-site Ti4+ ions. Nevertheless, some Ce4+ will turn to Ce3+ during the sintering process, then occupy the A-site, and B-site vacancies appear in the ceramic due to the stoichiometric ratio.
In order to avoid the degradation of electrical performance, compensation for the B-site vacancies is urgently required. In the modification of KNN-based ceramics, Sb with high Pauling electronegativity leads to significant improvements in the piezoelectric performance.31,32 When donor ions were added to ceramics, the oxygen vacancy concentration was restricted. As a result, the pinning of domains is reduced and the domain wall motion is promoted, which improves the electrical properties of ceramics.
In this study, we utilized the Ce synergizing with Sb to enhance the electrical properties of BCZT ceramics.
First, (Ba0.85Ca0.15)(Zr0.1Ti0.9−xCex)O3+ySb (abbreviated as BCZTCxSy, 0 ≤ x ≤ 0.15 mol% and 0 ≤ y ≤ 0.10 mol%) ceramics were prepared by the conventional solid solution processing route. The effects of Ce and Sb on the microstructure, dielectric, ferroelectric and piezoelectric of ceramics were studied, systematically. The diffusion behavior of the samples was analyzed through the correctional Curie–Weiss law. Benefiting from the grain coarsening and the restriction of the oxygen vacancy, the BCZTCxSy ceramics exhibited improved ferroelectric and piezoelectric performance. This study can provide an experimental basis for further modification of BCZT ceramics. The enhanced piezoelectric performance makes BCZT a potential candidate for the fabrication of lead-free devices.
Experimental procedures
Raw materials
Analytical-grade carbonate and metal oxide powders of BaCO3 (99.00%), CaCO3 (99.00%), TiO2 (98.00%) and ZrO2 (99.00%) were purchased from Sinopharm Chemical Reagent Co., Ltd. Sb2O5 (99.00%) was purchased from Shanghai Macklin Biochemical Co., Ltd. CeO2(99.00%) was purchased from Tianjin Guangfu Fine Chemical Research Institute.
Preparation of BCZT
(Ba0.85Ca0.15)(Zr0.1Ti0.9−xCex)O3+ySb (abbreviated as BCZTCxSy, 0 ≤ x ≤ 0.15 mol% and 0 ≤ y ≤ 0.1 mol%) ceramics were prepared by the conventional solid solution processing route. First, BaCO3, CaCO3, TiO2, ZrO2, Sb2O5 and CeO2 were mixed according to the stoichiometric ratio through planetary ball-milling in ethanol for 24 h. Then, the corresponding mixtures were calcined at 1200 °C for 4 h in an oxygen atmosphere, followed by another ball-milling for 24 h, and then dried at 60 °C for 12 h. The dried mixtures were granulated with appropriate polyvinyl alcohol (PVA) as the binder and pressed into disks of 13 mm in diameter and 1 mm thickness. Finally, the pellets were sintered at 1430 °C for 4 h in air. After cooling naturally, the as-sintered pellets were polished using a silicon carbide paper, both the surfaces of ceramics were coated with silver as electrodes.
Characterization and electrical property tests
X-ray diffraction (XRD) data were collected by using an X-ray diffractometer (DX-2700B, Haoyuan Instrument, China) with Cu Kα radiation of wavelength λ = 0.1540 nm. The optical micrograph (OLYMPUS BX51) was employed to identify the microstructure of materials. X-ray photoelectron spectroscopy (XPS) data were recorded using an Escalab 250Xi X-ray photoelectron spectrometer (Thermo Fisher Scientific). The volume density of the sample was measured by the Archimedes' method. The room-temperature dielectric properties were measured with a precision impedance tester (Agilent E4294A, Agilent Technologies, CA, USA). The dielectric temperature spectra of the sample were recorded using an LCR meter (Agilent E4980A, Agilent Technologies, CA, USA) under an increasing temperature range from 40 to 180 °C. The ferroelectric hysteresis loops (P–E) and electric field-induced strain loops (S–E) were measured using a ferroelectric tester (Radiant Multiferroic, USA). Then, the converse piezoelectric coefficient (
) was calculated based on the S–E spectrum. The piezoelectric constant d33 was obtained with a quasi-static d33 meter (ZJ-4AN, Institute of Acoustics, Beijing, China) after the samples were poled under a DC field of 3.5 kV mm−1 for 30 min at 60 °C.
Results and discussion
In attempts to detect the crystal structure of the obtained samples, (Ba0.85Ca0.15)(Zr0.1Ti0.9−xCex)O3 +ySb ceramics (abbreviated as BCZTCxSy, 0 ≤ x ≤ 0.15 mol% and 0 ≤ y ≤ 0.1 mol%) were characterized via XRD. As shown in Fig. 1(a), only the perovskite phase is observed and no impurity phases can be detected for all the samples, indicating that Ce and Sb diffused into the lattice to form a solid solution. Furthermore, crystallographic indexing and the splitting peak near 45° can be observed in Fig. 1(b), which implies the coexisting structures of orthorhombic with rhombohedral phases in the ceramic samples. Due to the similar ionic radii of Sb5+ (0.600 Å) and Ti4+ (0.605 Å), the in-plane lattice parameters (3.999 Å and 3.998 Å) of the ceramic sample show no significant changes after Sb doping. Considering that Ce exhibit +3 and +4 valences, the occupation mechanism for samples that are doped with Ce is complicated, Although CeO2 is used as a dopant, Ce3+ ions might be generated during the sintering process.33 Upon the introduction of 0.10 mol% Ce into the BCZT ceramic, the in-plane lattice parameter increased from 3.999 Å to 4.002 Å, implying that most of the Ce occupies B-site in the samples. The ionic radius of Ce4+ (0.870 Å) is bigger than that of Ti4+ (0.605 Å), whereas the ionic radius of Ce3+ (1.340 Å) is smaller than that of Ba2+ (1.610 Å). The B-site occupation is considered as the dominant form for Ce4+ in the Ce-doped BCZT ceramics.
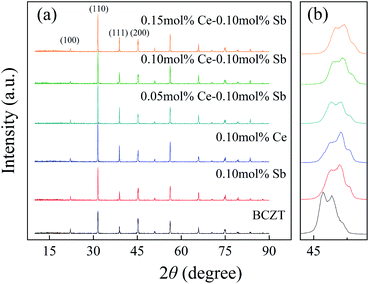 |
| Fig. 1 (a) XRD patterns of BCZT ceramics prepared by co-doping with Ce and Sb and (b) enlarge patterns near 45°. All the measurements were conducted at room temperature. | |
The surface micrographs of ceramic samples are shown in Fig. 2. All the samples demonstrate an irregularly elliptical grain. With the increase in the number of dopants, the bonding between the grains becomes tighter, indicating the reduction in the number of pores in the sample. In order to study the effect of dopants on the microstructure of the ceramics, the statistic distribution of the grain size for more than 900 grains in every sample was investigated. The grain size distribution maps are shown in Fig. 3. It was found that dopants can significantly enlarge the grain size of the samples. It can be seen in Fig. 3(a)–(e) that when 0.05 mol% Ce and 0.10 mol% Sb were co-doped into the BCZT sample the average grain size grew from 11.10 μm to 13.51 μm with a standard deviation of 5.93.
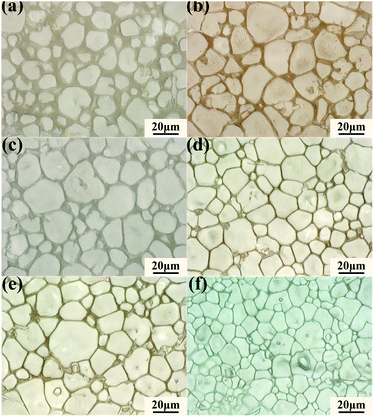 |
| Fig. 2 Surface morphologies of BCZTCxSy ceramics: (a) BCZT; (b) x = 0.10; (c) y = 0.10; (d) x = 0.05, y = 0.10; (e) x = 0.10, y = 0.10; (f) x = 0.15, y = 0.10. | |
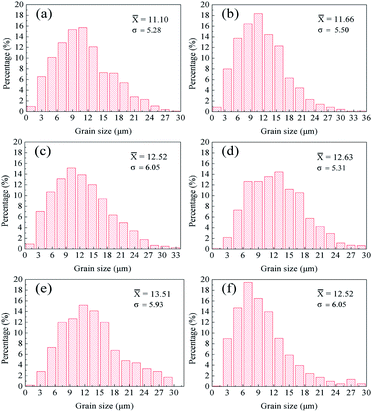 |
| Fig. 3 Grain size distribution in BCZTCxSy ceramics: (a) BCZT; (b) x = 0.10; (c) y = 0.10; (d) x = 0.05, y = 0.10; (e) x = 0.10, y = 0.10; (f) x = 0.15, y = 0.10. | |
During sintering, titanium was easily enriched at grain boundaries, and the impurity phases (such as BaTi2O5, BaTi3O7) were formed.34 The impurity phases converted back to BaTiO3 during the cooling process, and then generated numerous oxygen vacancies and barium vacancies. Compared to the inside grains, the oxygen vacancies would rather accumulate at grain boundaries. The good thermal stability of oxygen vacancies at grain boundaries was considered to hinder the growth of grains.35 When Ce and Sb were used as dopants for the donor doping of ceramics, the barium vacancies were generated inside the grain. The barium vacancies inside the grains facilitate the diffusion of ions. Moreover, the oxygen vacancy concentration on grain boundaries would be restricted. This can be attributed to the increase in barium ions at grain boundaries, preventing the generation of the impurity phase. Nevertheless, excessive Ce doping would restrain the growth of grain. Because CeO2 will precipitate at the grain boundaries, thus causing grain refinement.
To determine if the oxygen vacancy concentration in BCZT was restricted by Ce doping, XPS for the pure BCZT and 0.10 mol% Ce-doped BCZT were measured. The XPS spectra of O 1s binding states are shown in Fig. 4. The curve can be fitted with two peaks of the binding energy centered at 529.3 eV and 531.1 eV. The peak at 529.1 eV corresponds to the lattice oxygen and the peak at 531.1 eV is assigned to oxygen vacancies.36 When 0.10 mol% Ce was doped into BCZT, the relative density ratio of vacancy oxygen (OVacancy/OLattice) was decreased from 1.54 to 1.04. This result undoubtedly indicates that Ce doping in BCZT would restrict the oxygen vacancy concentration.
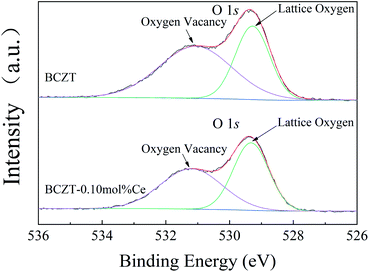 |
| Fig. 4 XPS spectra of O 1s binding states for BCZT and BCZT–0.10 mol% Ce. | |
Density is closely related to the grain size and pores for ceramics. Fig. 5 shows the relative density and volume density for ceramic samples with the co-doping of Ce and Sb. When 0.05 mol% Ce and 0.10 mol% Sb were co-doped into the ceramic, the optimal relative density could reach 93.52%. The increasing relative density may have been caused by the grain growth, which reduced the pores and increased the unit cell volume of Ce and Sb co-doped ceramic samples.
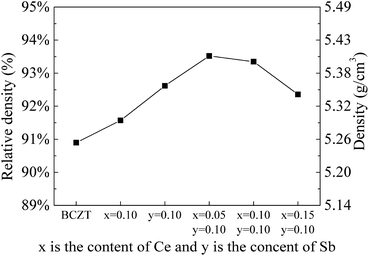 |
| Fig. 5 Relative density and density of BCZTCxSy ceramics. | |
In order to investigate the influence of dopants on the dielectric performance, the frequency-dependent dielectric permittivity (εr) for BCZTCxSy ceramics was measured at room temperature. As depicted in Fig. 6, all samples exhibiting high εr. Also, εr decreased with the increase in frequency. For BCZT ceramics, the εr value is mainly contributed by the bulk and domain wall motion: εr = εr.vol + εr.wall.37
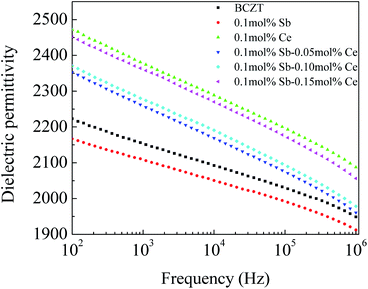 |
| Fig. 6 Frequency-dependent dielectric permittivity of BCZTCxSy ceramics at room temperature. | |
According to the dielectric polarization theory, the micro-polarization mechanism of bulk ceramics involves three types: electron displacement polarization, ion displacement polarization and turning-direction polarization. Due to the different polarization mechanisms, εr was varied at different frequencies. At lower frequencies, all three polarization mechanisms contribute to εr, resulting in a large εr value. As the frequency went up to 106 Hz, the turning of the electric dipole moment could not keep up with the increase in the electric field frequency. Thus, εr was decreased.
Moreover, the motion of the domain wall under an alternating electric field cannot be ignored. To reduce the system energy in polycrystals, multiple electrical domains would be formed inside the grain. The domain wall vibrates at its equilibrium position under an alternating electric field, which increases capacitance. As the electric field frequency was gradually increased, the domain wall would fail to move synchronously. As a result, the εr value was decreased.
Compared to the undoped ceramic, εr for sample with the 0.10 mol% Sb-doped sample slightly decreased. It may have been caused by the reduction of interfacial polarization and domain wall motions. As shown in Fig. 2(b) and 3(b), the introduction of 0.10 mol% Sb facilitates the grain growth. The tight bonding between grains then reduced the interface concentration and restricted domain wall motion. When Ce was doped into the samples, the lattice distortion and generated cation vacancies increased the spontaneous polarization and facilitated domain inversion. As a result, εr was increased.
The frequency-dependent dielectric losses (tan
δ) for BCZTCxSy ceramics at room temperature are measured. As shown in Fig. 7, the dielectric loss of all ceramic samples first decreased slightly with the increase in frequency then increased rapidly. The main reason for the dielectric loss at a low frequency is the resistance caused by the electric dipole turning and the friction of the domain wall motion.38 The turning-direction polarizations become weaker as the frequency gradually increases. As a result, the dielectric loss is slightly reduced. However, at higher frequencies, the domain wall frictional movement would increase the dielectric loss.
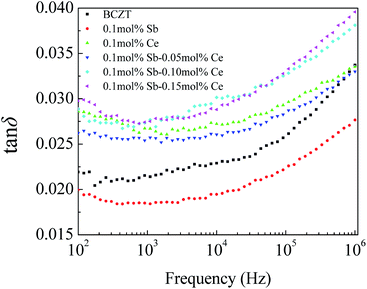 |
| Fig. 7 Frequency-dependent dielectric loss of BCZTCxSy ceramics at room temperature. | |
Compared to that for undoped ceramic sample, the dielectric loss for 0.10 mol% Sb-doped sample is lower. This contrast could be explained by increased grain size, increased domain size and restriction of the friction between the domain walls. Moreover, the decreased intergranular porosity leads to a decrease in the conductivity loss. When Ce co-doped into the samples, dielectric loss was increased. This behavior can be attributed to the lattice distortion, which promotes the domain wall motion.
To investigate the phase structure variation of ceramic samples with temperature, we measured temperature-dependent dielectric permittivity at the frequency of 1 kHz for BCZTCxSy. As shown in Fig. 8, the Curie temperature (Tc) of the sample rises to 110.2 °C and 109.1 °C when 0.10 mol% Ce and Sb were doped, respectively, into the BCZT ceramic.
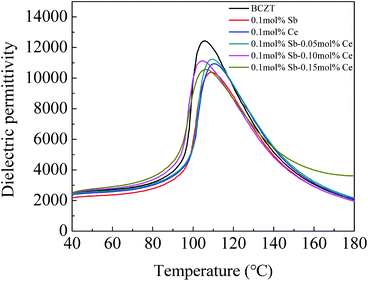 |
| Fig. 8 Temperature dependence of dielectric permittivity for BCZTCxSy ceramics at 1 kHz. | |
The increase in Tc indicates the improved stability of the ceramic when used at room temperature. According to Fig. 2 and 3, dopants can effectively facilitate the growth of grains, which benefits the release of internal stress for grains, thus increasing Tc.39 Nevertheless, dopants will increase the concentration of cation vacancies, which reduces the stability of oxygen octahedron.21 As a result, Tc decreased slightly with the increase in Ce doping. Compared with Tc for the undoped BCZT ceramics, Tc for the Ce and Sb co-doped ceramic was elevated. Moreover, the peak for dielectric Tc was broadened. In order to quantitatively characterize the degree of the diffuse behavior, the modified Curie–Weiss law was employed in this study,40
|
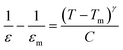 | (1) |
where
εm is the maximum permittivity,
C is the modified Curie–Weiss constant, and
γ value ranges from 1 (normal ferroelectric) to 2 (typical relaxer ferroelectric). According to temperature-dependent dielectric permittivity at 10 kHz, the parameter
γ can be obtained by the slope of ln(1/
ε − 1/
εm)
versus ln(
T −
Tm), as shown in
Fig. 9. It can be observed that
γ of the pure BCZT sample is 1.667 and increases to 1.733 with 0.10 mol% Sb and 0.15 mol% Ce co-doping. The increase in the value of
γ indicates that the ceramics exhibit more relaxed ferroelectric characteristics. The doping of Ce and Sb leads to a cation disorder in the perovskite unit cell, which elevates the stability of polar nanoregions (PNRs). In addition, macrodomains could be divided into micro-domains due to the increase in the PNR concentration. Thus, the ceramics show a more relaxer-like behavior.
41
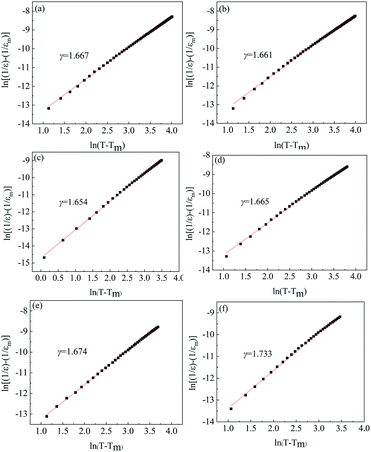 |
| Fig. 9 Linear fitted ln[(1/ε) − (1/εm)] as a function of ln(T − Tm) for BCZTCxSy ceramics at 10 kHz: (a) BCZT; (b) x = 0.10; (c) y = 0.10; (d) x = 0.05, y = 0.10; (e) x = 0.10, y = 0.10; (f) x = 0.15, y = 0.10. | |
The hysteresis loops, ferroelectric parameter remnant polarization (Pr) and coercive field (Ec) of BCZTCxSy ceramics measured at room temperature are shown in Fig. 10(a) and (b), respectively. It can be observed that all the P–E loops are saturated, and Pr can be obviously improved by dopants. When 0.10 mol% Sb and 0.05 mol% Ce were co-doped into ceramic, Pr was increased from 6.67 to 10.09 μC cm−2 and Ec was decreased to a minimum 2.87 kV cm−1. All the results indicate the enhanced ferroelectricity of BCZT.
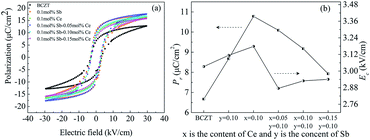 |
| Fig. 10 Room temperature ferroelectricity of BCZTCxSy ceramics: (a) electric hysteresis loops (b) Pr and Ec as a function of Ce and Sb contents. | |
The enhanced Pr of Ce and Sb co-doped ceramics may have been caused by the increase in the grain size. For ferroelectrics, the proportion of grains contributing to polarization reverses can be expressed as42
|
f = f0[1 − e−Gad3/kT]
| (2) |
where
d is the grain size, and
Ga is a constant used to describe the grain anisotropy energy density. Therefore,
f is only relevant to the grain size
d. According to
Fig. 2 and
3, dopants can promote the grain growth and then enhance the ferroelectricity. Moreover, the coarsening of grain elevates the domain width, and reduces the grain boundaries, which is beneficial to the continuous inversion of polarization. However, with the increase in the concentration of dopants, the PNRs generated inside the grain were increased. The appearance of PNRs makes the domain structure more complicated, resulting in the reduction of
Pr and
Ec for samples.
In order to explore the effect of dopants on the piezoelectric performance, the unipolar S–E loops at 30 kV cm−1 and the converse piezoelectric coefficient (
) were studied, and the data are shown in Fig. 11(a) and (b), respectively. It is can be seen that the unipolar positive strain is enhanced when ceramic is co-doped with Ce and Sb. The ceramic co-doping with 0.05 mol% Ce and 0.10 mol% Sb shows the maximum value of 0.125%, indicating a significant improvement in the piezoelectric properties of BCZT ceramics. In addition,
can be calculated by the unipolar S–E loops, which are shown in Fig. 11(b). All the result indicates that the dopants improve the
of ceramics, and the 0.05 mol% Ce and 0.10 mol% Sb co-doped ceramic show the optimum value of 417 pm V−1.
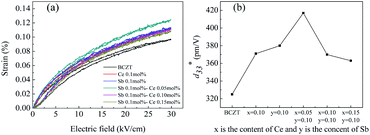 |
| Fig. 11 (a) Unipolar S–E loops and (b) of Ce and Sb co-doped ceramics. | |
The room temperature piezoelectric coefficient (d33) of BCZTCxSy ceramics poled under a DC field of 3.5 kV cm−1 is shown in Fig. 12. It can be found that dopants show obvious enhancement for the d33 of ceramics. The ceramics with 0.05 mol% Ce and 0.10 mol% Sb co-doped exhibit an optimal piezoelectric coefficient d33 of 372 pC N−1. The improved piezoelectric performance may have resulted from two reasons. The first reason is that more 90° domains were formed in the grains due to the internal stress of ceramics released with the increase in the grain size.43 Moreover, grain coarsening increases the domain width and size. The other reason is the restriction of the oxygen vacancy concentration, which can reduce the pinning of domains and promote the domain wall motion. In addition, the appearance of PNRs can reduce Ec, which then makes the samples easier for polarization under the electric field. However, when an excessive dopant was applied, the grain size would be reduced and more PNRs would be formed in grains. As a result, the growth of domains was restricted, which reduced the piezoelectric performance of ceramics.
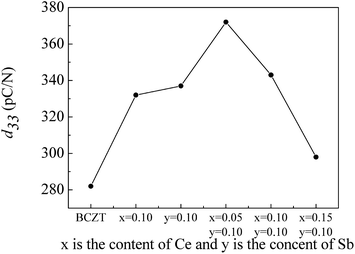 |
| Fig. 12 Piezoelectric coefficient of Ce and Sb co-doped ceramics. | |
Conclusions
In this study, we utilized Ce to synergize with Sb to enhance the electrical properties of BCZT ceramics. The co-doped sample with 0.05 mol% Ce and 0.10 mol% Sb shows the optimal properties (Pmax = 17.65 μC cm−2, Pr = 10.43 μC cm−2, Ec = 2.88 kV cm−1,
= 417 pm V−1, d33 = 372 pC N−1, Tc = 109.4 °C). Two reasons were considered for the improvement of ferroelectric and piezoelectric performances. One is the grain coarsening that increases the domain width and size. The other is the restriction of the oxygen vacancy concentration by the donor doped. Therefore, the pinning of domains is reduced and the domain wall motion is promoted simultaneously. In addition, PNRs would be formed with the co-doping of Ce and Sb. The PNRs reduce the coercive field and then facilitate the polarization of the sample. With the addition of dopants, the diffuseness γ was increased from 1.663 to 1.733, indicating a more relaxer ferroelectric characteristic. The increase in Tc indicates the improved stability of the ceramic samples when used at room temperature. All improvements in BCZT ceramics would show their promising applications in electronic devices.
Conflicts of interest
There are no conflicts to declare.
Acknowledgements
This work was financially supported by the University Nursing Program for Young Scholars with Creative Talents in the Heilongjiang Province under Grant No. UNPYSCT-2017095, the Natural Science Foundation of the Heilongjiang Province under Grants No. YQ2019A004 and LH2019F022, the National Natural Science Foundation of China under Grants No. 11444004 and 61372013, and the National Science Foundation for Post-Doctoral Scientists of the Heilongjiang Province under Grant No. LBH-Z19070.
Notes and references
- B. S. Aronov, E. K. Aikins and D. A. Brown, Increasing piezoelectric effect in radially polarized soft piezoelectric cylinders by pressure treating and its practical applications, J. Acoust. Soc. Am., 2020, 147(6), 4145–4152 CrossRef CAS.
- C. Othmani, H. Zhang and C. Lu, Effects of initial stresses on guided wave propagation in multilayered PZT-4/PZT-5A composites: a polynomial expansion approach, Appl. Math. Model., 2020, 78, 148–168 CrossRef.
- M. Y. Li, N. Y. Chan and D. Y. Wang, Improved thermal stability of the piezoelectric properties of (Li,Ag)-co-modified (K,Na)NbO3-based ceramics prepared by spark plasma sintering, J. Am. Ceram. Soc., 2017, 100(7), 1–7 Search PubMed.
- G. S. Xu, H. S. Luo, H. Q. Xu and Z. W. Yin, Third ferroelectric phase in PMNT single crystals near the morphotropic phase boundary composition, Phys. Rev. B: Condens. Matter Mater. Phys, 2001, 64(2), 20102 CrossRef.
- L. Qing, Y. C. Zhang, G. Jing, Z. Zhou, H. Wang, K. Wang, X. W. Zhang, L. T. Li and J. F. Li, High-performance lead-free piezoelectrics with local structural heterogeneity, Energy Environ. Sci., 2018, 11(12), 3531–3539 RSC.
- J. G. Wu, Perovskite lead-free piezoelectric ceramics, J. Appl. Phys., 2020, 127(19), 190901 CrossRef CAS.
- S. J. Zhang, R. Xia and T. R. Shrout, Modified (K0.5Na0.5)NbO3 based lead-free piezoelectrics with broad temperature usage range, Appl. Phys. Lett., 2007, 91(13), 132913 CrossRef.
- V. Fruth, L. Mitoseriu, D. Berger, A. lanculescu, C. Matei, S. Preda and M. Zaharescu, Preparation and characterization of BiFeO3 ceramic, Prog. Solid State Chem., 2007, 35, 193–202 CrossRef CAS.
- H. H. Gu, K. Zhu, X. P. Pang, B. Shao, J. H. Qiu and H. L. Ji, Synthesis of (K,Na)(Nb,Ta)O3 lead-free piezoelectric ceramic powders by high temperature mixing method under hydrothermal conditions, Ceram. Int., 2012, 38(3), 1807–1813 CrossRef CAS.
- M. Feizpour, H. B. Bafrooei H, R. Hayati and T. Ebadzadeh, Microwave-assisted synthesis and sintering of potassium sodium niobate lead-free piezoelectric ceramics, Ceram. Int., 2014, 40(1), 871–877 CrossRef CAS.
- H. W. Lu, J. Q. Lin and H. W. Zheng, Superior ferroelectric properties and fatigue resistance in Tb modified (BaCa)(ZrTi)O3 film grown on SrTiO3 prepared by pulsed laser deposition, Appl. Surf. Sci., 2020, 527, 146892 CrossRef CAS.
- S. Suzuki, S. Yamaguchi, A. Doi, S. Abe, M. Matsuda, T. Nakamura, A. Ando and H. Sano, Effect of alloying Ni inner electrodes on the leakage current degradation of BaTiO3-based multilayer ceramic capacitors, Appl. Phys. Lett., 2020, 116(13), 132903 CrossRef CAS.
- N. H. B. Janil, M. H. B. H. Jumali, Z. Z. B. Zainuddin, I. Izzuddin and S. Pojprapai, Penentuan Parameter Optimum bagi Rawatan Pengutuban Elektrik ke atas Seramik-Piezo (BaTiO3) menggunakan Sistem Buatan Sendiri, Sains Malays., 2019, 48(2), 425–433 CrossRef CAS.
- H. Takahashi, Y. Numamoto, J. Tani, k. Matsuta, J. H. Qiu and S. Tsurekawa, Lead-Free Barium Titanate Ceramics with Large Piezoelectric Constant Fabricated by Microwave Sintering, Jpn. J. Appl. Phys., 2006, 45(1), 30–32 CrossRef CAS.
- H. S. Mallik, I. Fujii, G. P. Khanal, S. Kim, S. Ueno, T. Suzuki and S. Wada, Fabrication of 〈111〉-oriented BaTiO3 ceramics by high magnetic field electrophoretic deposition using hexagonal–tetragonal co-existing BaTiO3 powder, J. Ceram. Soc. Jpn., 2020, 128(8), 469–474 CrossRef CAS.
- F. Q. Guo, B. H. Zhang, Z. X. Fan, X. Peng, Q. Yang, Y. X. Dong and R. R. Chen, Grain size effects on piezoelectric properties of BaTiO3 ceramics prepared by spark plasma sintering, J. Mater. Sci.: Mater. Electron., 2016, 27(6), 5967–5971 CrossRef CAS.
- M. S. Alkathy and K. C. J. Raju, Structural, dielectric, electromechanical, piezoelectric, elastic and ferroelectric properties of lanthanum and sodium co-substituted barium titanate ceramics, J. Alloys Compd., 2018, 45(8), 10518–10524 Search PubMed.
- T. M. Kamel and G. D. With, Poling of hard ferroelectric PZT ceramics, J. Eur. Ceram. Soc., 2008, 28(9), 1827–1838 CrossRef CAS.
- Y. M. Li, W. Chen, Q. Xu, J. Zhou, Y. Wang and H. Sun, Piezoelectric and dielectric properties of CeO2-doped Bi0.5Na0.44K0.06TiO3 lead-free ceramics, Ceram. Int., 2007, 33(1), 95–99 CrossRef CAS.
- B. C. Keswani, S. I. Patil, A. R. James, Y. D. Kolekar and C. V. Ramana, Correlation between Structural, Ferroelectric, Piezoelectric and Dielectric Properties of Ba0.7Ca0.3TiO3–xBaTi0.8Zr0.2O3 (x = 0.45, 0.55) Ceramics, Ceram. Int., 2018, 44, 20921–20928 CrossRef CAS.
- M. T. Fu, C. X. Li, B. Yang, D. Q. Liu, L. Bian, Y. N. Hong, C. H. Zhang, C. T. Chen and J. Q. Lin, Structure and piezoelectric properties of MnO2 doped Ba0.985Ca0.005Ti0.98Sn0.02O3 lead-free ceramics, J. Mater. Sci.: Mater. Electron., 2019, 30, 18950–18958 CrossRef CAS.
- Y. Liu, Z. Xu, L. J. Liu and F. Li, Morphotropic phase boundary-like properties in a ferroelectric–paraelectric nanocomposite, J. Appl. Phys., 2019, 126(12), 124102:1–124102:8 Search PubMed.
- M. Budimir, D. Damjanovic and N. Setter, Piezoelectric Response and Free Energy Instability in the Perovskite Crystals BaTiO3, PbTiO3 and Pb(Zr,Ti)O3, Phys. Rev. B: Condens. Matter Mater. Phys, 2006, 73, 174106 CrossRef.
- W. F. Liu and X. B. Ren, Large Piezoelectric Effect in Pb-Free Ceramics, Phys. Rev. Lett., 2009, 103(25), 257602 CrossRef.
- H. Wu, Y. A. Huang, F. Xu, Y. Q. Duan and Z. P. Yin, Energy Harvesters for Wearable and Stretchable Electronics: From Flexibility to Stretchability, Adv. Mater., 2016, 28(45), 9881–9919 CrossRef CAS.
- K. K. Poon, M. C. Wurm, D. M. Evans, M. A. Einarsrud, R. Lutz and J. Glaum, Biocompatibility of (Ba,Ca)(Zr,Ti)O3 piezoelectric ceramics for bone replacement materials, J. Biomed. Mater. Res., Part B, 2019, 108(4), 1–9 Search PubMed.
- L. J. Wang, W. F. Bai, X. Y. Zhao, Y. Q. Ding, S. T. Wu, P. Zheng, P. Li and J. W. Zhai, Influences of rare earth site engineering on piezoelectric and electromechanical response of (Ba0.85Ca0.15)(Zr0.1Ti0.9)O3 lead-free ceramics, J. Mater. Sci.: Mater. Electron., 2020, 31(9), 6560–6573 CrossRef CAS.
- V. Bijalwan, P. Tofel and V. Holcman, Grain size dependence of the microstructures and functional properties of (Ba0.85 Ca0.15−xCex)(Zr0.1Ti0.9)O3 lead-free piezoelectric ceramics, J. Asian Ceram. Soc., 2018, 6(4), 384–393 CrossRef.
- R. Hayati, M. A. Bahrevar, Y. Ganjkhanlou, V. Rojas and J. Koruza, Electromechanical properties of Ce-doped (Ba0.85Ca0.15)(Zr0.1Ti0.9)O3 lead-free piezoceramics, J. Adv. Ceram., 2019, 8, 186–195 CrossRef CAS.
- Q. Xu, D. P. Huang, M. Chen, W. Chen, H. X. Liu and B. H. Kim, Effect of bismuth excess on ferroelectric and piezoelectric properties of a (Na0.5Bi0.5)TiO3–BaTiO3 composition near the morphotropic phase boundary, J. Alloys Compd., 2009, 471(1–2), 310–316 CrossRef CAS.
- Y. F. Chang, Z. P. Yang, L. R. Xiong, Z. H. Liu and Z. L. Wang, Phase Structure, Microstructure, and Electrical Properties of Sb-Modified (K,Na,Li)(Nb,Ta)O3 Piezoelectric Ceramics, J. Am. Ceram. Soc., 2010, 91(7), 2211–2216 CrossRef.
- D. Lin, K. W. Kwok, K. H. Lam and H. L. W. Chan, Structure, piezoelectric and ferroelectric properties of Li- and Sb-modified K0.5Na0.5NbO3 lead-free ceramics, J. Phys. D: Appl. Phys., 2004, 40(11), 3500–3505 CrossRef.
- D. Y. Lu, D. D. Han, X. Y. Sun, X. L. Zhuang and Y. F. Zhang, Raman Evidence for Ba-Site Ce3+ in BaTiO3, Jpn. J. Appl. Phys., 2013, 52(1), 111501 CrossRef.
- M. Wegmann, L. Watson and A. Hendry, XPS Analysis of Submicrometer Barium Titanate Powder, J. Am. Ceram. Soc., 2004, 87(3), 371–377 CrossRef CAS.
- J. Nowotny and M. Rekas, Defect chemistry of BaTiO3, Solid State Ionics, 1991, 49, 135–154 CrossRef CAS.
- Z. L. Miao, L. Chen, F. Zhou and Q. Wang, Modulation of Resistive Switching Characteristics for Individual BaTiO3 Microfiber by Surface Oxygen Vacancies, J. Phys. D: Appl. Phys., 2017, 51(2), 025107 CrossRef.
- G. Arlt and H. Peusens, The dielectric constant of coarse grained BaTiO3 ceramics, Ferroelectrics, 1983, 48(1), 213–224 CrossRef CAS.
- J. O. Gentner, P. Gerthsen, N. A. Schmidt and R. E. Send, Dielectric losses in ferroelectric ceramics produced by domain-wall motion, J. Appl. Phys., 1978, 49(8), 4485–4489 CrossRef CAS.
- H. J. Hwang, T. Nagai, T. Ohji, M. Sando, M. Toriyama and K. Niihara, Curie Temperature Anomaly in Lead Zirconate Titanate/Silver Composites, J. Am. Ceram. Soc., 2010, 81(3), 709–712 CrossRef.
- M. Wolters and A. J. Burggraaf, Relaxational polarization and diffuse phase transitions of LA-substituted Pb(Zr,Ti)O3-ceramics, Mater. Res. Bull., 1975, 10(5), 417–423 CrossRef CAS.
- L. J. Liu, Z. Yang, M. X. Wu, L. Fang and C. Z. Hu, Dielectric Properties of (NaBi(1−x)Kx)0.5Ti(1−x)NbxO3 Ceramics Fabricated by Mechanical Alloying, J. Alloys Compd., 2010, 507(1), 196–200 CrossRef CAS.
- J. G. Hao, W. F. Bai, W. Li and J. W. Zhai, Correlation Between the Microstructure and Electrical Properties in High-Performance (Ba0.85Ca0.15)(Zr0.1Ti0.9)O3 Lead-Free Piezoelectric Ceramics, J. Am. Ceram. Soc., 2012, 95(6), 1998–2006 CrossRef CAS.
- P. Zheng, J. L. Zhang, Y. Q. Tan and C. L. Wang, Grain-size effects on dielectric and piezoelectric properties of poled BaTiO3 ceramics, Acta Mater., 2012, 60(13–14), 5022–5030 CrossRef CAS.
|
This journal is © The Royal Society of Chemistry 2021 |