DOI:
10.1039/D0RA09105G
(Paper)
RSC Adv., 2021,
11, 288-295
Synthetic and thermal studies of four insensitive energetic materials based on oxidation of the melamine structure†
Received
25th October 2020
, Accepted 28th November 2020
First published on 23rd December 2020
Abstract
Oxidation of nitrogen-rich aromatic heterocycles has a significant impact on the development of energetic materials. 2,4,6-Triamino-1,3,5-triazine-1,3-dioxide (MDO) is a promising insensitive energetic backbone obtained from melamine under strong oxidation conditions with impressive thermal behaviors and detonation performances. In this paper, MDO was prepared with improved yields of 85% and its thermal behavior, non-isothermal decomposition kinetics and gas products were investigated in detail. The corresponding decomposition mechanism was also deduced by applying the TG-DSC-FTIR-MS technique for the first time. The decomposition temperature of MDO reaches 300 °C and the apparent activation energy of MDO (E) calculated by the Kissinger and Ozawa method proved to be 303.63 and 279.95 kJ mol−1, indicating great thermal stability. Three new monoanionic energetic salts with impressively improved properties were achieved based on the basicity of MDO with yields of >80%. Their thermal decomposition temperatures proved to be higher than 230 °C and their densities are in the range of 1.75–1.89 g cm−3. The calculations and experiments show that their detonation velocities (vD: 8711–9085 m s−1) are comparable to or exceed those of RDX (D: 8795 m s−1) while the sensitivities to impact (IS: 23–27 J) and friction (FS: >240 J) are much lower.
Introduction
The introduction of N-oxide moieties can greatly improve the physical properties and detonation performances of energetic materials.1–10 During the past few decades, N-oxide rich aromatic heterocycles have attracted intensive attention and numerous novel N-oxide rich energetic structures, such as 1,2,3,4-tetrazino[5,6-e]-1,2,3,4-tetrazine- 1,3,6,8-tetraoxide (TTTO),11,12 1-hydroxy-1H-[1,2,3]triazolo[4,5-e] [1,2,3,4]tetrazine 5,7-dioxide (HTTDO),13,14 and [1,2,5] oxadiazolo [3,4-e] [1,2,3,4] tetrazine 4,6-di-N-oxide15 were successfully achieved. From a structural point of view, strong hydrogen bonds will be formed by incorporating nitro or N-oxide groups with adjacent amino groups and typical structures include 2,6-diamino-3,5-dinitropyrazine-1-oxide (LLM-105),16,17 triamino-5-nitropyrimidine-1,3-dioxide (ICM-102),18 and 3,8-dinitropyrazolo[5,1-c][1,2,4] triazine-4,7-diamine.19 However, the preparations of these energetic structures still suffer multistep synthesis and a simple synthetic method is highly needed.20
Unlike nitro groups, N-oxide moieties are embedded in the molecular backbones and therefore, lead to more compact packings. Melamine is a trimer of cyanamide with a 1,3,5-triazine skeleton and has been extensively used for chemical engineering. Selective oxidation of melamine (Scheme 1) will be a straightforward strategy for the synthesis of hydrogen bond rich structure. For instance, 2,4,6-triamino-1,3,5-triazine-1,3-dioxide (MDO)21,22 is a promising insensitive heat-resistant energetic compounds in weaponry with high decomposition temperature (Td: >300 °C) and low impact sensitivity (IS: >20 J). In this paper, we synthesized the MDO by H2O2/CF3COOH oxidation with improved yield, moreover, by tuning with energetic anion (ClO4−, N(NO2)2− and NO3−), three new insensitive energetic monoanionic salts, including perchlorate salt of MDO (MDOP), dinitramide salt (MDONA) and mono nitrate salt (MDOMN) with significantly improved properties were prepared based on the basicity of MDO via ion exchange reaction. A comprehensively study of their thermal behaviors, non-isothermal decomposition kinetics and the thermal decomposition mechanisms were also carried out for the first time by using TG-DSC-FTIR-MS technique. In addition, the densities and sensitivities (to impact, friction and electrostatic-spark) were investigated by experimental approaches and the detonation performances were calculated.
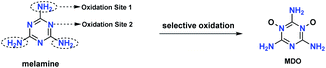 |
| Scheme 1 Selective oxidation of melamine. | |
Experiment
General caution! Although we have experienced no explosion accident in synthesis and characterization of these materials, proper protective measures should be adopted.
Materials and instruments
Ammonium perchlorate, ammonium dinitramide and ammonium nitrate were supplied by Xi'an Modern Chemistry Research Institute. Melamine, trifluoroacetic acid and hydrogen peroxide aqueous used in the study were purchased and used directly without further purification. The dehydrated MDO sample was used for the DSC-TG-MS-FTIR, density and sensitivity tests. 1H NMR and 13C NMR spectra were obtained on a Bruker 500 MHz spectrometer in deuterated dimethyl sulfoxide at room temperature, using trimethyl silane (TMS) as internal standard. The infrared spectra were recorded on a Nicolet NEXUS870 infrared spectrometer in the range of 4000 cm−1 to 400 cm−1 in KBr matrix. Elemental analysis (C, H and N) were carried out on a VARI-E1-3 elementary analysis instrument. The DSC and TG studies were undertaken on a TGA/DSC Mettler Toledo calorimeter at a heating rate of 10 °C min−1, under dry oxygen-free nitrogen atmosphere with a flowing rate of 50 mL min−1. The impact and friction sensitivities were determined using the BAM method. Electrostatic-spark sensitivities (E50) were measured on HT-201B-3 electrostatic-spark sensitivity tester, under electrode gap of 0.5 mm, electric capacity of 10
000 pF, E50 = 1/2CV (50)2. The synchronous thermal analysis was undertaken on a Germany Benz 449C TG-DSC synchronous thermal analyzer. The operation conditions are as follows: sample mass is about 0.35 mg, the crucible is made of aluminum, heating rates are 2.5, 5.0, 10.0 and 20.0 °C min−1, the flow rate of the dynamic argon gas atmosphere is 25 mL min−1. Infrared spectrometer was carried out on American Nicolet 5700 infrared spectrometer in the range of 4000–650 cm−1. The operation conditions are as follows: the detector is DTGS; resolution > 0.09 cm−1; purge gas flow is 50 mL min−1; protective gas flow is 25 mL min−1. The operating temperature of MS connection tube (sampling capillary) is 190 °C. Mass spectrometer was made on a Germany NETZSCH QMS403 quadrupole mass spectrometer. The operation conditions are as follows: the temperature of thermal analysis instrument and mass spectrometry and interface is 200 °C; the test mass range is between 1–300 amu; the resolution is <0.5 amu and the detection limit > 1 ppm.
Synthetic procedures
Synthesis of 2,4,6-triamino-1,3,5-triazine-1,3-dioxide (MDO). Melamine (0.5 g, 4 mmol) was suspended in 12 mL trifluoroacetic acid at 0 °C, then 5 mL 50% hydrogen peroxide aqueous was slowly added. After maintaining at room temperature for 5 h, white precipitate was filtered and dissolved in water. Then, the solution was neutralized to pH = 7 with NaHCO3, colorless precipitate was filtered out, washed with a small amount of cold water, and dried as 2,4,6-triamino-1,3,5-triazin-1,3-dioxide tetrahydrate (MDO·4H2O) (0.78 g, 85% yield). 1H NMR (500 MHz, DMSO-d6): δ ppm: 9.33 (s, 2H, NH2), 8.80 (s, 4H, NH2); 13C NMR (125 MHz, DMSO-d6): δ ppm: 150.97, 152.76; IR ν: 3416, 3346, 3185, 1678, 1467, 1428, 1250, 1196, 1140, 827, 798, 720, 680, 607 cm−1; elemental analysis calculated (%) for MDO·4H2O: C 15.65, H 6.13, N 36.51; found: C 15.37, H 6.04, N 37.21. MDO·4H2O (2 g) was putted in a round bottom flask and heated to 130 °C for 1 h to obtain MDO without H2O (1.35 g). Elemental analysis calculated (%) for MDO: C 22.79, H 3.82, N 53.15; found: C 23.01, H 3.75, N 53.35.
Synthesis of perchlorate salt of 2,4,6-triamino-1,3,5-triazine-1,3-dioxide (MDOP). Melamine (0.5 g, 4 mmol) was suspended in 12 mL trifluoroacetic acid at 0 °C, then 5 mL hydrogen peroxide aqueous (50%) was slowly added. After maintaining at room temperature for 5 h, white precipitate was filtered and dissolved in water. Ammonium perchlorate (0.41 g, 3.5 mmol) was added in batches, and the reaction was carried out at 0 °C for 1 h, white precipitate was filtered and the filter cake was washed with cold water, drying in the air to get MDOP (0.83 g, 81% yield). 1H NMR (500 MHz, DMSO-d6): δ ppm: 8.69 (s, 2H, NH2), 9.14 (s, 2H, NH2), 9.37 (s, 2H, NH2); 13C NMR (125 MHz, DMSO-d6): δ ppm: 151.92, 152.63; IR ν: 3427, 3360, 3290, 1682, 1661, 1506, 1254, 1113, 1084, 728, 628 cm−1; elemental analysis calculated (%) for MDOP: C 13.94, H 2.73, N 32.50; found: C 13.72, H 2.87, N 32.64.
Synthesis of dinitramide salt of 2,4,6-triamino-1,3,5-triazine-1,3-dioxide (MDONA). Melamine (0.5 g, 4 mmol) was suspended in 12 mL trifluoroacetic acid at 0 °C, then 5 mL hydrogen peroxide aqueous (50%) was slowly added. After maintaining at room temperature for 5 h, white precipitate was filtered and dissolved in water. Ammonium dinitramide (0.44 g, 3.5 mmol) was added in batches, and the reaction was carried out at 0 °C for 1 h, white precipitate was filtered and the filter cake was washed with cold water, drying in the air to get MDONA (0.87 g, 83% yield). 1H NMR (500 MHz, DMSO-d6): δ ppm: 8.69 (s, 2H, NH2), 9.14 (s, 2H, NH2), 9.37 (s, 2H, NH2); 13C NMR (125 MHz, DMSO-d6): δ ppm: 151.92, 152.63; IR ν: 3416, 3278, 3134, 1674, 1648, 1510, 1437, 1327, 1244, 1171, 1010, 894 cm−1; elemental analysis calculated (%) for MDONA: C 13.59, H 2.66, N 47.54; found: C 13.41, H 2.82, N 47.60.
Synthesis of mono nitrate salt of 2,4,6-triamino-1,3,5-triazine-1,3-dioxide (MDOMN). Melamine (0.5 g, 4 mmol) was suspended in 12 mL trifluoroacetic acid at 0 °C, then 5 mL hydrogen peroxide aqueous was slowly added. After maintaining at room temperature for 5 h, white precipitate was filtered and dissolved in water. Ammonium nitrate (0.28 g, 3.5 mmol) was added in batches, and the reaction was carried out at 0 °C for 1 h, white precipitate was filtered and the filter cake was washed with cold water, drying in the air to get MDOMN·H2O (0.73 g, 83% yield). 1H NMR (500 MHz, DMSO-d6): δ ppm: 8.72 (s, 2H, NH2), 9.14 (s, 2H, NH2), 9.39 (s, 2H, NH2); 13C NMR (125 MHz, DMSO-d6): δ ppm: 151.92, 152.62; IR ν: 3597, 3433, 3165, 1668, 1644, 1499, 1384, 1316, 1246, 1185, 892, 726, 665 cm−1; elemental analysis calculated (%) for MDOMN·H2O: C 15.07, H 3.79, N 41.00; found: C 15.19, H 3.75, N 41.93.
Results and discussion
Controllable synthesis
In the melamine molecule, both the amino group and the N atom on the triazine backbone are reaction sites that could be oxidized, so which site is more reactive? We conducted comparative analyses of the reactivities of melamine and 1,3,5-triazine molecules through the surface electrostatic potential (ESP). It was found that the introduction of the three amino groups to the triazine backbone structure (Fig. 1a) completely changes the charge distribution of the 1,3,5-triazine (Fig. 1b). In the melamine molecule, the electron cloud distribution is concentrated on the triazine backbone and the N atom due to the p–π conjugation effect between the amino groups and the triazine ring, so that the triazine ring and the nitrogen atom are both negatively charged. The surface minimum point of melamine is located on the N atoms of triazine backbone with value of −30.27 kcal mol−1. Moreover, due to the electron-withdrawing effect of the N atoms on the H atoms in the amino groups, the H atoms exhibit positive charge with the surface maximum point value of 32.98 kcal mol−1. Therefore, in the melamine molecular structure, the N atoms on the triazine ring are easier to be oxidized than the amino groups.
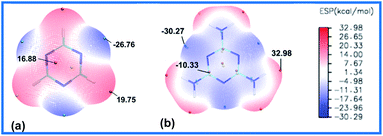 |
| Fig. 1 (a) Electrostatic potential of 1,3,5-triazine; (b) electrostatic potential of melamine. | |
Initially, we commenced our experiments using H2O2/HCOOH and H2O2/CH3COOH as oxidant and melamine as starting material (Scheme 3). Unfortunately, we didn't obtain MDO at the temperatures of 20 °C, 40 °C or 60 °C, and mixture products were generated, possibly caused by the weak oxidation capacity of the chosen oxidants that leads to poor selectivity. Subsequently, we employed 50% H2O2/CF3COOH and 98% H2O2/CF3COOH as the oxidants respectively, and two nitrogen atoms of melamine were oxidized successfully, generating MDO with improved yield of 85%. While the yield of MDO was only around 50% when 30% H2O2/CF3COOH was used as the oxidant due to the weakening of the oxidation capacity.22 Both elemental analysis and single-crystal X-ray diffraction indicated that the MDO existed as tetrahydrate, and H2O molecules could be completely removed by heating MDO·4H2O sample at 130 °C. It is noteworthy that using the cheap and readily available melamine as starting material and this one-step synthetic strategy with simple post-treatment, the large-scale production of MDO could potentially be achieved.
MDO is a nitrogen-rich energetic compound. It is readily to be protonated to generate MDO ionic salts, taking advantage of the negative polarity of oxygen atoms or nitrogen atoms. Siwei Song et al.22 reported two dianionic salts of MDO (2,4,6-triamino-1,3,5-triazine-1,3-dioxide-HNO3 (TTDON) and 2,4,6-triamino-1,3,5-triazine-1,3-dioxide-HClO4 (TTDOP)) prepared via three-step reactions with the yields of 40% and 54% under strong acid solutions. However, the impact sensitivities of these two compounds are 14 J and 13 J, respectively, which is dangerous for further synthesis and storage of them (Scheme 2).
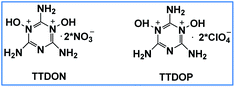 |
| Scheme 2 The structures of TTDON and TTDOP.22 | |
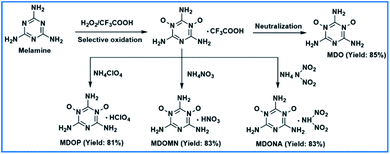 |
| Scheme 3 The synthesis of MDO, MDOP, MDONA and MDOMN. | |
Here, using intermediate MDO·CF3COOH as starting material, three new MDO energetic monoanionic salts were prepared by ion exchange reaction, with yields ranging from 81% to 87%. Compared with TTDON and TTDOP, the strategy applied in our paper exhibits shorter reaction time, higher yields and safer operations.
Thermal analysis of MDO
Thermal analysis is important for the production, storage, transportation, and application of energetic materials. The thermal behavior, non-isothermal decomposition kinetics and gas products generated from thermal decomposition of MDO were investigated in detail by using a set of specific experimental devices (i.e., TG-DSC-FTIR-MS technique), under the heating rate of 2.5, 5, 10 and 20 °C min−1. Based on the results, the thermal decomposition mechanism of MDO was deduced. Fig. 2 shows the DSC-TG trace of MDO under a heating rate of 10 °C min−1. MDO commenced to decompose around 273.4 °C without melting process. With the increasing of temperature, the decomposition of MDO became more intense, reaching the maximum at around 318.9 °C. It is noteworthy that the decomposition temperature of MDO is comparable to that of the reference thermostable HNS explosive (Td: 315 °C), indicating MDO possess a good thermal stability.
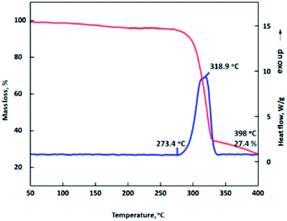 |
| Fig. 2 DSC-TG trace of MDO under a heating rate of 10 °C min−1. | |
In order to get further information about the thermal decomposition process of MDO, the reaction kinetic parameters during the heating process of MDO were studied by Kissinger's and Ozawa–Doyle's method.23–25 The DSC traces at different heating rates (2.5, 5, 10 and 20 °C min−1) were shown in Fig. 3. The apparent activation energy E for the thermal decomposition of MDO calculated by Kissinger and Ozawa method is 303.63 kJ mol−1 and 279.95 kJ mol−1, respectively (Table 1). Notably, the linear correlation coefficients (r) calculated by Kissinger's method (0.9903) and Ozawa's method (0.9896) are very close and greater than 0.98. So the calculated results are credible and provide a good reference for the thermal safety of MDO.
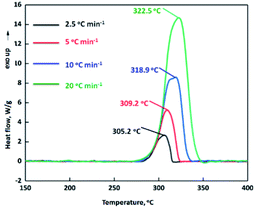 |
| Fig. 3 DSC traces of MDO at different heating rates. | |
Table 1 Kinetic parameters and enthalpies of thermal decomposition of MDO
β K min−1 |
Tp K |
Ea/(kJ mol−1) |
rb |
ln(Akc s−1) |
Kissinger |
Ozawa |
Kissinger |
Ozawa |
Kissinger |
Apparent activation energy. Liner correlation coefficient. Pre-exponential factor. |
2.5 |
542.32 |
303.63 |
279.95 |
0.9903 |
0.9896 |
91.44 |
5 |
546.33 |
10 |
556.09 |
20 |
559.65 |
FTIR and MS are used for in situ tracking analysis, providing a comprehensive profile of the gas products. The three-dimensional IR absorption spectrum of MDO is shown in Fig. 4a, illustrating the changes of absorbance intensity of gases released during the decomposition of MDO with time (temperature) under a heating rate of 10 °C min−1. The gas products started to appear about 270 °C, and the concentration reached maximum at around 319 °C. The changing trend of absorbance intensity is in good consistency with DSC-TG curve (Fig. 2). From the infrared spectrum of the gas generated at 319 °C (Fig. 4b), the gas products are identified as: H2O (3748, 1576 cm−1), CO2 (2357, 2311, 2288, 672 cm−1), N2O (2248 cm−1), HCN (716 cm cm−1), NH3 (3335, 969, 933 cm−1).
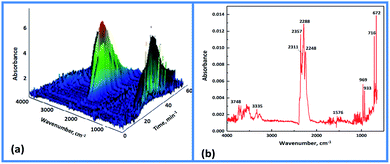 |
| Fig. 4 (a) 3D IR absorption spectrum of MDO at a heating rate of 10 °C min−1; (b) IR spectrum of MDO at 319 °C. | |
The mass spectrum of the gas products generated during thermal decomposition of MDO (Fig. 5) indicates the relationship between MS ion current intensity and temperature. The intensity of MS ion current originated from each gas fragment reaches highest at around 319 °C, which is in good consistency with DSC characterization (Fig. 2) and three-dimensional IR analysis (Fig. 4a). These results also suggest that MDO decomposed directly and intensely around this temperature. From the mass of the ion fragments, the gas products generated from thermal decomposition of MDO are assigned to: NH3 (m/z = 15, 16, 17), NO (m/z = 30), NO2 (m/z = 46), N2O or CO2 (m/z = 44), H2O (m/z = 18), CO or N2 (m/z = 28), HN
C
NH (m/z = 42), HCN (m/z = 26, 27). Since N2 is infrared inactive and CO was not detected by IR, the ion current at m/z = 28 should be assigned to N2. Taking both IR and MS characterization into consideration, the gas products generated from thermal decomposition of MDO are primarily H2O, CO2, N2O, HCN, NH3, NO, NO2, and N2.
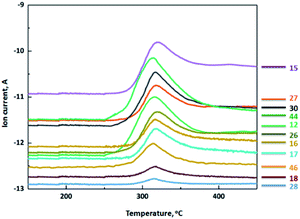 |
| Fig. 5 The mass spectra of the gas products generated from thermal decomposition of MDO. | |
On the basis of the above-described DSC-TG-MS-IR analytical results and relative knowledge in the literature, we deduced a plausible thermal decomposition mechanism of MDO, as schematically illustrated in Scheme 4. Because of the strong polarity of N–O bond in MDO, the oxygen atom exhibits negative charge. This condition is very favorable for the transfer of proton from the adjacent amino group to oxygen atom. Hence, the oxygen atom is protonated to afford b.26 With the increasing of temperature, b loses one molecule of water and decomposes to c. Subsequently, the cleavage of C–N bond of c leads to the release of nitrogen oxides (e.g., N2O, NO, NO2). Meanwhile, owning to the instability of N
C
N bond, this bond of c cleaves and generates intermediate d. We inferred that the intermediate d may further decompose via two different pathways. The intermediate d might undergo coupling reaction to generate cross-linking compounds, or decompose into smaller molecules such as N2 and NH3.27,28
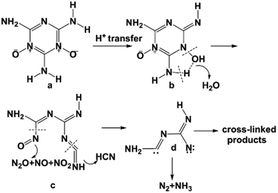 |
| Scheme 4 Plausible thermal decomposition mechanism of MDO. | |
Thermal behaviours of MDOP, MDONA and MDOMN
Differential scanning calorimetric (DSC) and thermal gravimetric analyzer (TG) measurements were used to determine the thermo-dynamic stability of MDO energetic ionic salts from 40 °C to 400 °C. All these three energetic salts are decomposed directly without melting process. The decomposition temperatures (Td) of MDONA, MDOMN and MDOP are 231.5 °C, 242.3 °C and 286.5 °C, respectively (Fig. 6).
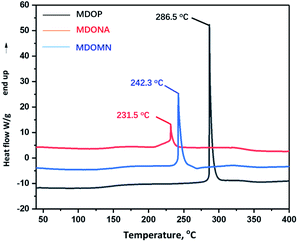 |
| Fig. 6 DSC curves of MDONA, MDOMN, and MDOP. | |
The decomposition temperatures of MDOMN and MDONA are obviously higher than that of RDX (Td: 210 °C). It is noteworthy that MDOP exhibits good thermal stability that the decomposition temperature is 286.5 °C, which is significantly higher than RDX and even higher than HMX (Td: 279 °C). In addition, MDONA, MDOMN and MDOP shows better thermal stability than the dianionic salts TTDON (Td: 180 °C) and TTDOP (Td: 176 °C).22
The DSC traces of MDONA, MDOMN and MDOP obtained at different heating rates of 2.5, 5, 10 and 20 °C min−1 are shown in Fig. 7. Based on the Kissinger's and Ozawa–Doyle's methods, the non-isothermal kinetic parameters during the heating processes of MDONA, MDOMN and MDOP were investigated with the linear correlation coefficients (r) of greater than 0.98. From Table 2, although the decomposition point of MDOP is the highest among these three compounds, the apparent activation energy E for it is the lowest of 116.16 kJ mol−1 by Kissinger method and 165.78 kJ mol−1 by Ozawa method.
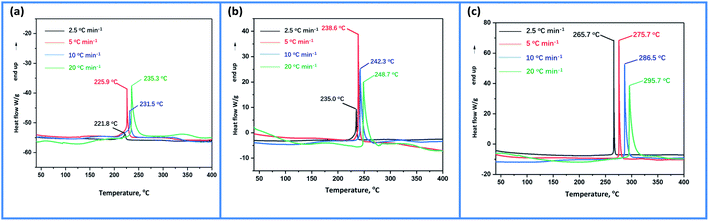 |
| Fig. 7 DSC traces of MDONA (a), MDOMN (b) and MDOP (c) at different heating rates. | |
Table 2 Kinetic parameters and enthalpies of thermal decomposition of MDONA, MDOMN and MDOP
Comp. |
β (K min−1) |
Tp (K) |
Ea/(kJ mol−1) |
rb |
ln(Akc s−1) |
Kissinger |
Ozawa |
Kissinger |
Ozawa |
Kissinger |
Apparent activation energy. Liner correlation coefficient. Pre-exponential factor. |
MDONA |
2.5 |
494.98 |
306.69 |
298.11 |
0.99367 |
0.99441 |
73.56 |
5 |
499.05 |
10 |
504.65 |
20 |
508.45 |
MDOMN |
2.5 |
508.15 |
329.46 |
321.45 |
0.98065 |
0.98162 |
77.10 |
5 |
512.13 |
10 |
515.48 |
20 |
521.82 |
MDOP |
2.5 |
538.90 |
166.16 |
165.78 |
0.99818 |
0.99834 |
35.31 |
5 |
548.82 |
10 |
559.65 |
20 |
568.82 |
Physiochemical and energetic properties of MDO, MDOP, MDONA and MDOMN
The experimental density of MDO was 1.71 g cm−3 as measured by gas pycnometer, which is comparable to the widely used insensitive reference thermostable explosive HNS (D: 1.70 g cm−3). Based on the heat of formation calculated by Gaussian 09 program29 and tested density, the detonation performances were calculated by EXPLO5 (v 6.01).30 The results showed MDO possesses a detonation velocity of 6988 m s−1 and a detonation pressure of 21.4 GPa, which are approximately equivalent to those of HNS. Furthermore, the tested impact sensitivity (IS) and friction sensitivity (FS) of MDO are greater than 40 J and 360 N, respectively. The electrostatic-spark sensitivity of MDO is 53 mJ, which is very close to that of HNS (Table 3).
Table 3 Comparison of the physicochemical properties and detonation performances
Comp. |
Tda (°C) |
D b (g cm−3) |
ΔfHc (kJ mol−1) |
Ωd (%) |
vDe (m s−1) |
Pf (GPa) |
ISg (J) |
FSh (N) |
E50i (mJ) |
Decomposition temperature (exothermic peak). Experimental density measured by gas pycnometer (25 °C). Heats of formation calculated by Gaussian 09. Oxygen balance (based on CO2) for CaHbOcNd, 16(c − (2a + 0.5b))/Mw, Mw = molecular weight. Calculated detonation velocity (EXPLO5 v 6.01). Calculated detonation pressure (EXPLO5 v 6.01). Impact sensitivity evaluated by a standard BAM fall-Hammer. Friction sensitivity evaluated by BAM technique. Measured electrostatic-spark sensitivity, E50 = 1/2CV (50)2. |
MDO |
319 |
1.71 |
−1.71 |
−70.9 |
6988 |
21.4 |
>40 |
>360 |
53 |
MDOP |
287 |
1.89 |
104.4 |
−21.7 |
8711 |
34.4 |
23 |
240 |
97 |
MDONA |
232 |
1.82 |
259.4 |
−21.1 |
9085 |
35.4 |
27 |
>360 |
153 |
MDOMN |
242 |
1.75 |
59.5 |
−32.6 |
8857 |
32.3 |
27 |
>360 |
142 |
TTDON22 |
180 |
1.79 |
−31.5 |
−11.3 |
8900 |
34.2 |
14 |
— |
— |
TTDOP22 |
176 |
1.99 |
714.2 |
4.5 |
9284 |
41.0 |
13 |
— |
— |
HNS |
315 |
1.70 |
78.2 |
−69.4 |
7000 (ref. 31) |
21.8 (ref. 31) |
5 |
240 |
62 |
RDX |
210 (ref. 18) |
1.80 |
86.3 (ref. 18) |
−21.6 |
8795 |
34.9 |
7.5 (ref. 18) |
120 (ref. 18) |
70 |
HMX |
279 (ref. 18) |
1.90 |
116.1 (ref. 18) |
−21.7 |
9144 |
39.2 |
7.51 (ref. 8) |
120 (ref. 18) |
37 |
In comparison with MDO, the ionic salts of MDO show higher densities and much improved detonation performances: the experimental densities of the energetic salts are between 1.75–1.89 g cm−3, the detonation velocities are greater than 8700 m s−1, which are similar to those of RDX. More importantly, these energetic salts also possess low sensitivities to impact (IS: >20 J), friction (FS: 240–360 N) and electrostatic-spark (E50: >90 mJ). The sensitivity properties are apparently superior to those of RDX (IS: 7.4 J, FS: 120 J, E50: 70 mJ) and HMX (IS: 7.4 J, FS: 120 J, E50: 37 mJ). It's worth to point out that the reason of the thermal and mechanical stability of MDO are much better than that of MDOMN, MDOP and MDONA is closely related to their molecular structure. From the crystal structure of MDO in Fig. S1,† MDO possesses a complete planar structure and all the atoms are in the same plane. Numerous strong intermolecular hydrogen bonds formed between the adjacent layers, which further afford MDO a face-to-face π–π stacking structure and stabilized its solid structure. With the help of the π–π stacking structure, the large conjugated system and large number of hydrogen bonds, MDO is insensitive to mechanical stimulation and has good thermal stability. After the introduction of anions (ClO4−, N(NO2)2− and NO3−), the complete planar configuration was destroyed, so the thermal decomposition temperatures and mechanical stabilities of MDOP, MDOMN and MDONA decreased. Moreover, the oxygen content of the compounds increases significantly after the introduction of oxygen rich anions, and the increased O⋯O interactions will increase the mechanical sensitivity of the compounds. Compared with dianionic salts TTDON (IS: 14 J) and TTDOP (IS: 14 J),22 the three energetic salts are less sensitivity to impact. These results clearly indicate that the energetic salts of MDO are a class of promising new energetic ionic salts with high energy and low sensitivity.
Conclusions
In conclusion, we have demonstrated efficient synthetic and thermal studies on MDO based on oxidations of melamine structures. Thermal behavior of MDO in detail and possible thermal decomposition mechanism based on the results of TG-DSC-FTIR-MS technique were investigated for the first time. The gas products of MDO during the thermal decomposition are primarily H2O, CO2, N2O, HCN, NH3, NO, NO2, and N2. The apparent activation energy of MDO (E) calculated by Kissinger and Ozawa method were 303.63 and 279.95 kJ mol−1, indicating the good thermal stability of MDO. Originated from the conjugated structure and the abundant hydrogen bonds between the amino group and N-oxide moiety, MDO exhibits similar performances to commonly used explosive HNS, representing a promising insensitive heat-resistant energetic compound. By tuning with energetic anions, three novel energetic ionic salts of MDO with improved performances, namely MDOP, MDONA, and MDOMN, were further designed and synthesized. MDOP, MDONA, and MDOMN possess thermal decomposition temperatures higher than 230 °C and decomposed directly without melting process. The three energetic salts hold satisfactory detonation performances (vD: 8711–9085 m s−1, P: 32.3–35.4 GPa) which are comparable to that of RDX, but with lower sensitivities to impact, friction and electrostatic-spark (IS: 23–27 J, FS: >240 J, E50: 97–153 mJ), showing potential application prospect in the field of propellants.
Conflicts of interest
There are no conflicts to declare.
Acknowledgements
We are grateful to the financial support from National Natural Science Foundation of China (No. 21805223).
Notes and references
- C. J. Snyder, L. A. Wells, D. E. Chavez, G. H. Imler and D. A. Parrish, Chem. Commun., 2019, 55, 2461 RSC.
- C. L. He, H. X. Gao, G. H. Imler, D. A. Parrish and J. M. Shreeve, J. Mater. Chem. A, 2018, 6, 9391 RSC.
- D. Fischer, T. M. KlapÖtke, D. G. Piercey and J. Stierstorfer, Chem.–Eur. J., 2013, 19, 4602 CrossRef CAS.
- Q. Wu, W. H. Zhu and H. M. Xiao, RSC Adv., 2014, 4, 53000 RSC.
- A. A. Voronin, V. P. Zelenov, A. M. Churakov, Y. A. Strelenko and V. A. Tartakovsky, Russ. Chem. Bull., 2014, 63, 475 CrossRef CAS.
- V. P. Zelenov, A. A. Voronin, A. M. Churakov, Y. A. Strelenko and V. A. Tartakovsky, Russ. Chem. Bull., 2014, 63, 123 CrossRef CAS.
- A. M. Churakov, O. Y. Smirnov, S. L. Loffe, Y. A. Strelenko and V. A. Tartakovsy, Eur. J. Org. Chem., 2002, 14, 2342 CrossRef.
- Y. Shang, B. Jin, R. F. Peng, Z. C. Guo, Q. Q. Liu, J. Zhao and Q. C. Zhang, RSC Adv., 2016, 6, 48590 RSC.
- H. Wei, H. Gao and J. M. Shreeve, Chem.–Eur. J., 2015, 21, 2726 CrossRef CAS.
- L. Hu, P. Yin, G. H. Imler, D. A. Parrish, H. X. Gao and J. M. Shreeve, Chem. Commun., 2019, 55, 8979 RSC.
- M. S. Klenov, A. A. Guskov, O. V. Anikin, A. M. Churakov, Y. A. Strelenko, I. V. Fedyanin, K. A. Lyssenko and V. A. Tartakovsky, Angew. Chem., Int. Ed., 2016, 55, 11472 CrossRef CAS.
- P. Politzer, P. Lane and J. S. Murray, Cent. Eur. J. Energ. Mater., 2013, 10, 37 Search PubMed; K. O. Christe, D. A. Dixon, M. Vasiliu, R. I. Wagner, R. Haiges, J. A. Boatz and H. L. Ammon, Propellants, Explos., Pyrotech., 2015, 40, 463 CrossRef CAS.
- A. A. Voronin, V. P. Zelenov, A. M. Churakov, Y. A. Strelenko, I. V. Fedyanin and V. A. Tartakovsky, Tetrahedron, 2014, 70, 3018 CrossRef CAS.
- Y. F. Luo, F. Q. Bi, L. J. Zhai, X. Z. Li, J. L. Zhang and B. Z. Wang, Chin. J. Energ. Mater., 2018, 26, 919 Search PubMed.
- A. M. Churakov, S. L. Loffe and V. A. Tartakovsky, Mendeleev Commun., 1995, 5, 227 CrossRef.
- C. M. Tarver, P. A. Urtiew and T. D. Tran, J. Energ. Mater., 2005, 23, 183 CrossRef CAS.
- P. F. Pagoria, A. R. Mitchell and K. Bala, US Pat., US20090299067 A1, 2009 Search PubMed.
- Y. Wang, Y. J. Liu, S. W. Song, Z. J. Yang, X. J. Qi, K. C. Wang, Y. Liu, Q. H. Zhang and Y. Tian, Nat. Commun., 2018, 9, 2444 CrossRef.
- Y. X. Tang, C. L. He, G. H. Imler, D. A. Parrish and J. M. Shreeve, ACS Appl. Energy Mater., 2019, 2, 2263 CrossRef CAS.
- M. B. Talawar, R. Sivabalan, T. Mukundan, H. Muthurajan, A. K. Sikder, B. R. Gandhe and A. Subhananda Rao, J. Hazard. Mater., 2009, 161, 589 CrossRef CAS.
- J. R. Zhang, F. Q. Bi and B. Z. Wang, 50th International annual conference of the Fraunhofer ICT, 2019, June 25–28 Search PubMed.
- S. W. Song, Y. Wang, W. He, K. C. Wang and Q. H. Zhang, Chem. Eng. J., 2020, 35, 125114 CrossRef.
- H. E. Kissinger, Anal. Chem., 1957, 29, 1702 CrossRef CAS.
- T. A. Ozawa, Bull. Chem. Soc. Jpn., 1965, 38, 1881 CrossRef CAS.
- J. Zhou, L. Ding, F. Q. Zhao, B. Z. Wang and J. L. Zhang, Chin. Chem. Lett., 2019, 31, 554 CrossRef.
- C. C. Ye, Q. An, T. Cheng, S. Zybinm, S. Naserifar, X. H. Ju and W. A. Goddard III, J. Mater. Chem. A, 2015, 3, 12044 RSC.
- G. Singh, I. P. Kapoor and S. K. Tiwari, J. Hazard. Mater., 2001, 81, 67 CrossRef CAS.
- I. P. Kapoor, P. Srivastava and G. Singh, J. Hazard. Mater., 2008, 150, 687 CrossRef CAS.
- M. J. Frisch, G. W. Trucks, H. B. Schlegel, G. E. Scuseria, M. A. Robb, J. R. Cheeseman, J. A. Montgomery Jr, T. Vreven, K. N. Kudin, J. C. Burant, J. M. Millam, S. S. Iyengar, J. Tomasi, V. Barone, B. Mennucci, M. Cossi, G. Scalmani, N. Rega, G. A. Petersson, H. Nakatsuji, M. Hada, M. Ehara, K. Toyota, R. Fukuda, J. Hasegawa, M. Ishida, T. Nakajima, Y. Honda, O. Kitao, H. Nakai, M. Klene, X. Li, J. E. Knox, H. P. Hratchian, J. B. Cross, V. Bakken, C. Adamo, J. Jaramillo, R. Gomperts, R. E. Stratmann, O. Yazyev, A. J. Austin, R. Cammi, C. Pomelli, J. W. Ochterski, P. Y. Ayala, K. Morokuma, G. A. Voth, P. Sal-vador, J. J. Dannenberg, V. G. Zakrzewski, S. Dapprich, A. D. Daniels, M. C. Strain, O. Farkas, D. K. Malick, A. D. Rabuck, K. Raghavachari, J. B. Foresman, J. V. Ortiz, Q. Cui, A. G. Ba-boul, S. Clifford, J. Cioslowski, B. B. Stefanov, G. Liu, A. Liashenko, P. Piskorz, I. Komaromi, R. L. Martin, D. J. Fox, T. Keith, M. A. Al-Laham, C. Y. Peng, A. Nanayakkara, M. Challacombe, P. M. W. Gill, B. Johnson, W. Chen, M. W. Wong, C. Gonzalez and J. A. Pople, Gaussian 09, rev. A.02, Gaussian, Inc., Wallingford, CT, 2009 Search PubMed.
- M. Suceska, EXPLO5, Version 6.04, 2017 Search PubMed.
- B. Singh and R. K. Malhotra, Def. Sci. J., 1983, 33, 165 CrossRef CAS.
Footnote |
† Electronic supplementary information (ESI) available. CCDC 1947761. For ESI and crystallographic data in CIF or other electronic format see DOI: 10.1039/d0ra09105g |
|
This journal is © The Royal Society of Chemistry 2021 |