DOI:
10.1039/D0QM00723D
(Research Article)
Mater. Chem. Front., 2021,
5, 472-481
Biomorphic triangulations: constructing an additional formation pathway to achieve hierarchical self-evolution in biomorphs†
Received
17th September 2020
, Accepted 19th October 2020
First published on 20th October 2020
Abstract
Biomimetic morphologies constructed in pure inorganic systems, called biomorphs, provide a promising approach to produce advanced biomimetic materials via bottom-up self-organization. Although existing biomorphs show the potential to imitate complex and/or hierarchical micro-architectures, they can only be achieved by changing the conditions manually during the reaction, not an intelligent morphological self-evolution. In this contribution, we propose a strategy to introduce more than one type of initial variable for the construction of different formation/transition pathways to realize a hierarchical architecture via self-evolution. A hierarchical architecture with unique triangular branches was obtained successfully by initial control of both the temperature and Mg2+ doping, highlighting two pathways for the textured growth of polycrystals and single crystals in different hierarchies led by CO2 and MgCO3, respectively. Our findings lay the foundation for the future construction of materials with new complex hierarchical architectures via a self-evolution approach realized by rational pathway design.
Introduction
Biomorphs are the biomimetic morphologies, with non-crystallographic structural features, typically constructed in pure inorganic systems by the self-organization of silicon-coated carbonate nanocrystals.1–3 This self-organization process can be simply triggered by precipitating carbonate in alkaline silicon-containing solution or silica gels,4 and the resulting microstructure exhibits impressive similarity with biominerals, which breaks the morphological barrier between the abiotic and the biotic.5,6 The incredible but easy-to-implement bionic ability demonstrated in this system provides a promising strategy for the construction of advanced biomimetic materials.7–9 Thus, great efforts have been made to generate polymorphic (refer in particular to the morphological diversity in this article) biomorphs by modulating various physical or chemical variables such as temperature,10 flow field,11 pH,12 species concentration,13,14 doping or not15,16 as control schemes during the preparation process. And the resultant biomorphs did show distinct structural features, namely flat sheets, worms, corals, helicoids, conical vases and stems etc. (Fig. 1A, reproduced by us). However, if we consider the diversity and complexity of morphologies in nature, the present achievements are still far from enough: (1) current biomorphs are generally characterized by smoothly curved shape,17,18 lacking non-sinuous but also life-like shapes. Therefore, the species of achieved polymorphisms are actually not rich enough for the potential application foreground of this system. (2) Biomorphs with hierarchical morphological characteristics have been reported in BaCO3/SiO2 and CaCO3/SiO2 systems, which could only be realized by switching growth conditions manually during the formation process.19,20 Nevertheless, the emulation of a complex morphological evolution without manual interventions, which serves as a widely used intelligent biomaterial synthesis strategy for nature,21–23 is still unachievable in this system. In living organisms, numerous building blocks self-organize into intricate dynamic structures caused by the cooperation of multiple factors in the physiological environment, macroscopically manifesting as a spontaneous hierarchical morphological evolution process (known as self-evolution), then underlie the generation of diverse biological functions.24–26
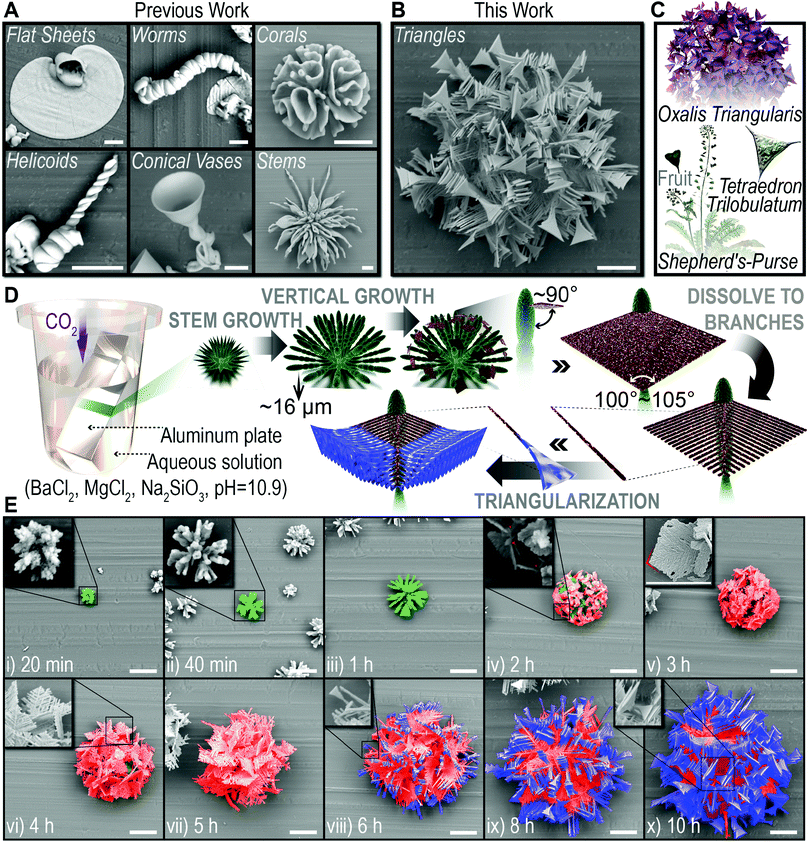 |
| Fig. 1 Biomorphs and the self-evolution process of “triangles”. (A and B) SEM images of previously reported biomorphs reproduced by our lab (A) and “triangles” (B). (C) Triangles in nature, including the leaves of Oxalis triangularis, and the fruits of shepherd's-purse and Tetraedron trilobulatum (side length is about 25 μm). (D) Schematic representation of the self-evolution process of “triangles”. (E) False-colored SEM images of the self-evolution process of “triangles” with increasing reaction time (from 20 min to 10 h). The false colors are consistent with the texture colors in the scheme (D). Inset: SEM images for partial enlargements (i, ii, iv, vi, vii and x) and high-resolution SEM images of a diamond-shaped slice (v) with typical morphological characteristics marked in red. Scale bars, 20 μm (A, B and E). | |
Following the idea of bio-inspiration, we believe that new biomorphs could be constructed via a quite different formation/transition pathway achieved by incorporating more than one type of regulatory mechanism into this system. Accordingly, we have made a long-term effort, and in this contribution, we report the first case of non-sinuous biomorphs, “triangles”, which is reached when we introduce a chemical variable (by doping magnesium ions) and a physical variable (by controlling temperature) simultaneously before depositing BaCO3 in Na2SiO3 solution. The biomorph is a spherical cluster, with unexpected triangle branches as morphological features, hence the name “triangles” (Fig. 1B). Triangle is a basic shape and widely exists in bio-systems over various scales. Presented examples here, including the leaves of Oxalis triangularis, and the fruits of shepherd's-purse and Tetraedron trilobulatum (Fig. 1C), are all from nature and have morphological similarity to the “triangles” we obtained. Even more exciting is the fact that the formation of the “triangles” has been observed to undergo four distinct but continuous periods, which are stem growth, vertical growth, dissolving into branches and triangularization (Fig. 1D and E), providing the first example of intelligent hierarchical self-evolution. Based on the exploration of its mechanism, we are further able to tune the formation pathway of the “triangles” by altering the initial conditions, and obtain a series of derivative hierarchical biomorphs, including two complex hierarchical architectures that the “triangles” grow on stems and corals (i.e. biomorph-on-biomorph). Our findings not only demonstrate a rational approach for the discovery of polymorphic biomorphs with as-yet-unknown morphological features, but also underline the pivotal role of the formation pathway to the final morphology of biomorphs. Moreover, the intelligent self-evolution behavior opens the possibility of combining structural diversities and integrating two or more distinct biomorphs into one architecture without manual intervention, for the design of materials that possess complex and contradictory morphological features and even properties.
Results and discussion
Observation of the hierarchical self-evolution of “triangles”
The “triangles” were prepared by gas diffusion on an aluminum plate as a growth substrate, at a Mg2+ doping ratio of 0.2 (molar ratio, Mg2+/Ba2+) and a fixed temperature of 30 °C.4 The formation process is extracted into a schematic representation (Fig. 1D) based on the scanning electron microscope (SEM) observation (Fig. 1E and Fig. S1, ESI†). Typically, the carbonate single crystal seed forms at the very beginning,18 then splits at the tips, for the growth of subsequent biomorphs (Fig. 1E, 20 min).8 Afterwards, a consecutive self-evolution process was identified into four periods as follows.
(1) Stem growth. The thorny tips further grew into radially arranged stems with curved surfaces (Fig. 1E, 20 min−1 h),20 which would be the innermost texture of the entire “triangle” architecture.
(2) Vertical growth. The stem ceased to grow at a certain length (∼16 μm); instead, a diamond-shaped slice grew vertically from the top of it, with a thickness of 0.5–1 μm and an apex angle of 100–105° (Fig. 1E, 1–2 h). Then, the slices gradually covered the interior stems by extension in two-dimensions.
(3) Dissolving to branches. Surprisingly, the thickness of the slices was observed to oscillate in two-dimensions, manifesting dendritic stripes of different depths (Fig. 1E, 3 h, inset). Then the thin regions dissolved, while the thick regions continued to grow, causing the outer edge of the slices to continue expanding. As the thin regions dissolved completely, the slices eventually turned into a perfect dendritic crystal with an angle of about 60° between the trunk and the branches (Fig. 1E, 3–5 h).
(4) Triangularization. The fore-most ends of the branches gradually broadened in a direction vertical to the dendritic slices (Fig. 1E, 6 h, inset). Thus, the shape of the branches ended up as triangles (Fig. 1E, 6–10 h). Since then, the four-period self-evolution process had ended and the final hierarchical “triangles” had been formed.
Mechanism of the hierarchical self-evolution of “triangles”
Stem growth has an already-known mechanism, recognized as solution-directed growth.20 As to the other three growth periods, more detailed characterizations are needed for the exploration of their mechanisms.
(1) Vertical growth. First of all, we characterized the solution composition (1 h reaction) before vertical growth using transmission electron microscope (TEM), selected area electron diffraction (SAED) and energy dispersive X-ray (EDX) elemental mapping (Fig. 2A–G). Rod and spherical-shaped nanoparticles were found in the TEM image (Fig. 2C), showing polycrystalline (corresponding to witherite) and amorphous diffraction patterns, respectively (Fig. 2A and B). With further EDX elemental mapping (Fig. 2D–G), we confirmed that the two main solid phases were the rod-shaped BaCO3 nanocrystals and spherical amorphism rich in silicon and magnesium. Then, we studied the orientation of the stems and slices on the aluminum plate at the time of vertical growth (1.5 h reaction). The TEM image (Fig. 2H) and the corresponding SAED patterns (Fig. 2I–K) of a vertical growth fragment showed obvious diffraction rings of polycrystals for the stem and discrete spots of single crystals for the slice, and a superposition of both the diffraction rings and discrete spots for the junction part. Subsequently, the TEM image of a larger slice (2 h reaction) was obtained (Fig. 2L) and the corresponding SAED patterns showed clear diffraction spots, consistent with the [001] zone axis of the witherite lattice. Besides, rod-like BaCO3 nanocrystals, which are considered as typical building units for related biomorphs,2,27 were also observed in the SEM image (1.5 h reaction, Fig. 2N–P).
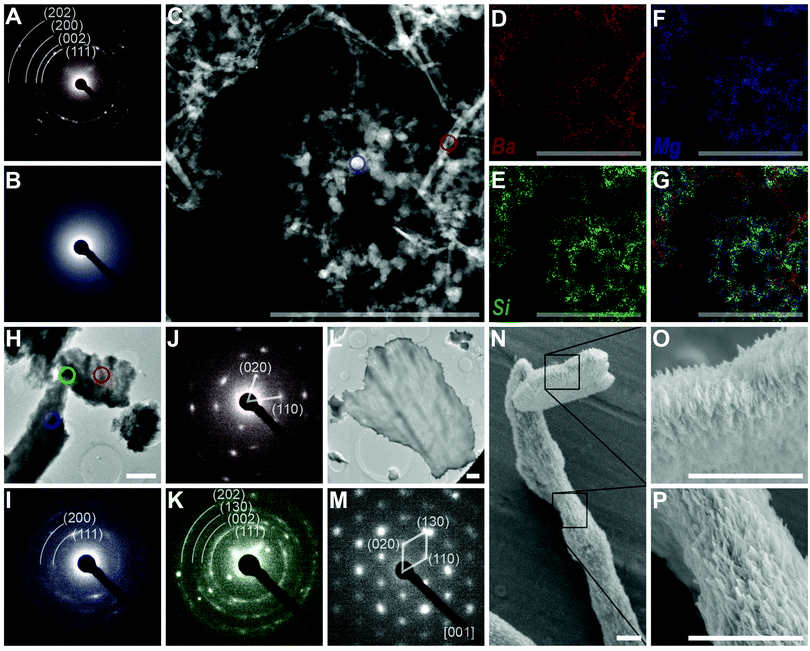 |
| Fig. 2 Vertical growth. (A to G) Respective SAED patterns of the rod (A) and the sphere (B) in red and blue circles in the TEM image (C) of the nanoparticles present in solution (1 h reaction), and corresponding EDX elemental mapping data (D)–(G). (H)–(K) TEM images of a vertical growth fragment of the stem and the slice (1.5 h reaction, H), and the corresponding SAED patterns (I)–(K, stem in blue circle, junction in green circle and slice in red circle, respectively). (L and M) TEM images (L) and corresponding SAED pattern (M) of a larger slice (2 h reaction). (N)–(P) SEM image of a slice vertically grown on a stem (1.5 h reaction, N), and the corresponding SEM images for partial enlargements of the slice (O) and the stem (P). Scale bars, 1 μm (C to H, L and N to P). | |
(2) Dissolving to branches. Based on the SEM observation of the two-dimensional slices (Fig. 1E, 3 h, inset), unpredictable dendritic stripes formed before dissolving. We further confirmed this special structural feature using atomic force microscopy (AFM, Fig. 3A–E). The inner texture of the biomorph was covered with scattered slices, resulting in extremely uneven height (2.5 h reaction, Fig. 3A), not conducive to analyzing the fine structure of each slice. Fortunately, the graphs of field phase (Fig. 3B) and vibrational energy (Fig. 3C) did show evidently the surface texture, dendritic stripes of different depth. Then, TEM and EDX elemental mapping were further performed to determine the respective composition of both the thin and thick regions (3.5 h reaction, Fig. 3F–J and Fig. S2, ESI†), showing obvious oscillation of the Ba/Si ratio along the trunk direction of forming branches. Both thin and thick regions had been confirmed to be witherite according to the SAED patterns (Fig. 3K–N), despite different dissolution or growth behaviors. And the SAED patterns of different areas of a formed branch (4 h reaction), from front edge to trunk, showed exactly the same diffraction spots indexed to the (110) and (020) crystal planes (Fig. 3O–S).
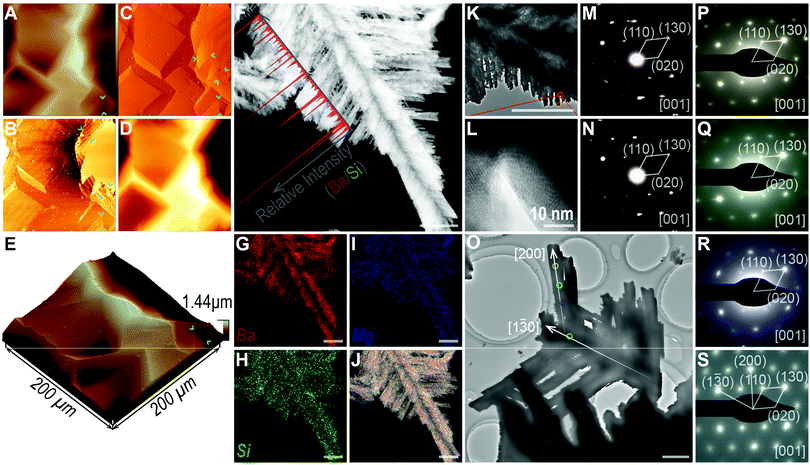 |
| Fig. 3 Dissolving to branches. (A)–(E) AFM analysis for an area of the biomorph (2.5 h reaction), including images of height difference (A), field phase (B), vibrational energy (C), Z-offset (D) and three-dimensional reconstructed height profile (E). Green arrows highlight the stripes of different depth. (F)–(J) TEM images of forming branches which contained the relative intensity profile of Ba/Si along the trunk direction (3.5 h reaction, F), and corresponding EDX elemental mapping data (G)–(J). (K)–(N) TEM images of a dissolving slice (3.5 h reaction, K) and a partially enlarged image of the dissolving edge (L), with the corresponding SAED patterns (M and N, the dissolving edge in red cycle and the forming branch in orange circle, respectively). (O)–(S) TEM images of the formed branches (4 h reaction, O) and SAED patterns of different areas of a branch (P to S, areas in yellow, green, blue, and indigo circles from the front edge to trunk, respectively). The crystal orientations indicated by arrows in the TEM image (O) are determined by the corresponding SAED patterns (P to S). Scale bars, 1 μm (F to L, O). | |
(3) Triangularization. Through ultrasound, we obtained isolated triangular branches with spear-like tail and wide flat front edge (10 h reaction, Fig. 4A–F). SAED patterns confirmed that both the tail and front edge were perfect single crystal, showing agreement with the [010] zone axis of witherite. Besides, the high-resolution TEM image (Fig. 4G) revealed the clear crystal lattice, giving a spacing of the lattice fringes in two directions of 0.326 nm and 0.266 nm, corresponding to the (002) and (200) planes of the witherite. EDX elemental mapping data of the border of a triangular branch suggested that the silica covered the carbonate structures as a shell, consistent with the previous studies.2,28
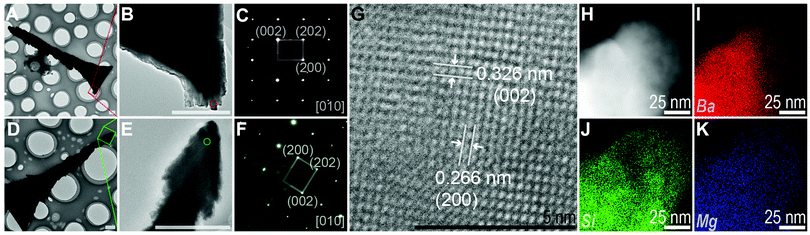 |
| Fig. 4 Triangularization. (A)–(F) TEM images of two isolated triangular branches (10 h reaction, A and D) and respective images for partial enlargements (B) and (E) with corresponding SAED patterns (C) and (F). (G) High-resolution TEM image of a triangular branch, with clear crystal lattices indexed to the (002) and (200) crystal planes of witherite. (H)–(K) EDX elemental mapping data of the border of a triangular branch. Scale bars, 1 μm (A, B, D and E). | |
Thus, the formation of “triangles” underwent the growth (co-orientation,27 particularly in this article) of polycrystals during stem growth, the growth of single crystals during vertical growth, dissolution into branches and triangularization. So, the occurrence of vertical growth is the dividing point of polycrystal and single crystal growth in the “triangles”.
Since CO2 gradually diffused into solution, four reactions would happen, as follows.
| Ba2+ + CO2 + H2O → BaCO3 + 2H+, Ksp,25 °C = 2.6 × 10−9 | (1) |
| SiO32− + 2H+ → SiO2 + H2O | (2) |
| Mg2+ + CO2 + H2O → MgCO3 + 2H+, Ksp,25 °C = 6.8 × 10−6 | (3) |
| Ba2+ + MgCO3 → Mg2+ + BaCO3 | (4) |
where
Ksp is the solubility product obtained from the previous report.
29
Because of the high initial concentration of Ba2+ and the low solubility product of BaCO3, stable three-dimensional nucleation happened at the beginning (reaction (1)), leading to polycrystalline stems of BaCO3.27 Meanwhile, SiO2 and MgCO3 would precipitate as well (reaction (2) and (3)), in the form of amorphous SiO2–MgCO3 nanoclusters according to the results of the SAED patterns and EDX elemental mapping (1 h reaction, Fig. 2B–G). We considered that these SiO2–MgCO3 complexes performed similar structural functions to the silica, gradually attached on the surface of the biomorphs and eventually formed a membrane at a macro level.2,28 And at the micro level, sparingly soluble MgCO3 in these complexes would serve as the carbonate source for the formation of insoluble BaCO3 (reaction (4)), embodied as an original pathway to construct biomorphs, giving the opportunity for two-dimensional growth of BaCO3 single crystals by moderate carbonate release.30,31 As the reaction progressed, SiO2–MgCO3 complexes were accumulating on the surface of the biomorphs, steering the two-dimensional growth of witherite single crystal slices along the preferred crystal directions [1
0] (formed the trunk in this direction) and [200] (formed the branches in this direction, Fig. 3O); hence vertical growth.
In the next period, the abnormal behavior, dissolved to branches, was attributed to Ostwald ripening. Ostwald ripening is a thermodynamically spontaneous process in which particles or crystals with high surface energy (usually characterized by large surface to volume ratio) dissolve and redeposit on ones with low surface energy.32–34 In our system, the thin regions possess larger surface to volume ratio and higher surface energy, and accordingly dissolved. Meanwhile, the thick regions could grow, resulting in the branches.
During triangularization, a typical diffusion-limited growth condition was formed. The Ba2+ near the front edge was depleted and the bulk solution could not replenish the consumed Ba2+ in time because of a low diffusion rate in a static solution, under which crystals tended to grow towards high concentration areas and formed outward expansion structures.10,35,36 Herein, the front edge of the branches could only broaden to draw the remaining Ba2+ in the bulk solution, and triangulated eventually.
Derivative morphologies
CO2 and MgCO3 are two different carbonate sources for witherite (reaction (1) and (4)), playing an important role in the morphological evolution of “triangles”. CO2 as a trigger to start the reaction, has an increasing then gradually smooth concentration in the solution along with its continuous diffusion and consumption, and meanwhile constructs polycrystalline witherite via a three-dimensional nucleation pathway. MgCO3, in the form of SiO2–MgCO3 complexes, attaches on the surface of biomorphs and supports the two-dimensional growth of single crystal witherite by gentle carbonate supply, representing an original pathway we proposed in this system. On the basis of the above understanding, we intend to alter the formation pathway to get derivative morphologies. The equilibrium concentration of CO2 and the content of MgCO3 can be adjusted separately by the temperature and the initial concentration of Mg2+ (Fig. 5A–G).
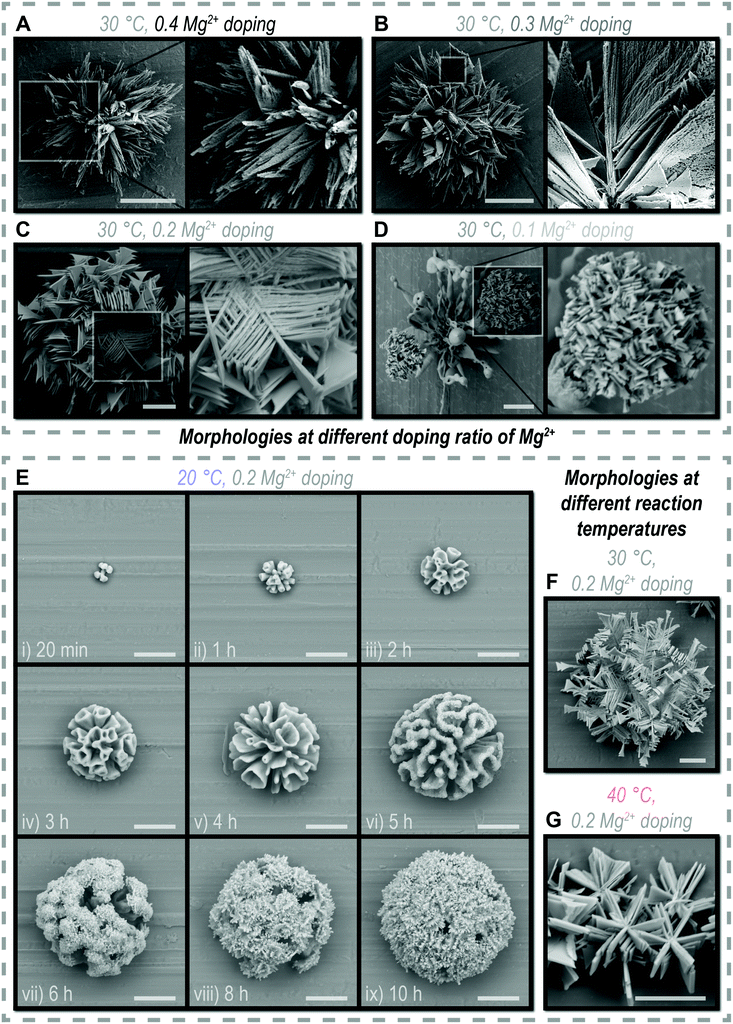 |
| Fig. 5 Derivative morphologies. (A)–(D) SEM images and enlarged SEM images of morphologies at different doping ratio of Mg2+. (E)–(G) SEM images of morphologies at different reaction temperatures, including the formation process of triangles-on-corals (E). Scale bars, 20 μm (A, B and E). | |
When increasing the initial concentration of Mg2+ (doping ratio, from 0.2 to 0.3), the SiO2–MgCO3 complexes may contain more MgCO3 composition to provide extra chances for two-dimension growth, resulting in denser triangular branches (Fig. 5B). However, a further increase in the Mg2+ concentration (doping ratio 0.4) did not result in a more dense branched biomorph but a spiny cluster (Fig. 5A), probably the excessive Mg2+ damaged the chemical surrounding for biomorph growth. When decreasing the initial concentration of Mg2+ (doping ratio, from 0.2 to 0.1), longer time was taken to accumulate enough MgCO3, so that the two-dimensional growth was delayed. Meanwhile, less MgCO3 composition was formed, making the triangular branches sparser. Thus, a hierarchical architecture where tiny “triangles” grew on vimineous stems was observed (Fig. 5D).
When decreasing the reaction temperature (from 30 to 20 °C), the increase of the equilibrium concentration of CO2 is beneficial to the three-dimension nucleation pathway.10,37 The formation process of a hierarchical biomorph-on-biomorph that great corals hold “triangles” was observed (Fig. 5E), in which the duration of three-dimension nucleation (for corals formation) was clearly extended (from about 1 h to 4 h, Fig. 1E and 5E). As the temperature increases (from 30 to 40 °C), the CO2-concentration-dependent three-dimensional nucleation pathway was severely inhibited and inferior to two-dimensional growth, leading to immense triangular branches (Fig. 5G).
Conclusions and prospects
Newly constructed biomorph “triangles” are presented here as an example to reveal a complex four-period hierarchical self-evolution process. Different from the previous reports, spontaneous dissolution happened based on thermodynamic surface energy minimization for the overall optimization of the architecture, showing a remarkable coincidence with the natural law “survival of the fittest”. Besides, a common sight that a polycrystalline texture grows on the single crystal carbonate core is inadequate to show the whole as later single crystal growth on the polycrystals can be achieved by an additional formation pathway led by MgCO3-to-BaCO3 precipitation transformation.18 Through other intentionally adjusted pathways, a series of derivative biomorphs were successfully evolved out by the simple control of the initial conditions, reminding us of the behavior that living organisms regulate different pathways to produce customized biological organization under an instinctive condition change and stimulus-response,38 thus suggesting more commonalities than we ever thought between biomorphs and organisms.
In this contribution, we controlled over Mg2+ and temperature to produce polymorphic biomorphs. Fortunately, we succeeded and got some amazing results. However, it should be noted that multiple factors will participate in the morphological evolution process and affect the final morphologies of the biomorphs, causing the nondeterminacy for the choice of factors and the resulting morphologies. The choosing of Mg2+ is not blind but on account of the fact that Mg2+ is used by all living organisms in biomineralization and cell differentiation, much less to serve the pivotal role in respiration and photosynthesis.39,40 Although the formation of biomorphs, biomineralization and the differentiation of organisms are different processes that happen in pure inorganic systems, inorganic systems affected by organics, and organic systems affected by organics/inorganics respectively, strange connections may exist and provide creative ideas to researchers.
Some issues remain unsolved for the current progress: (1) factors with unidentified mechanisms will cause unpredictable results. (2) Not all textures are controllable in a hierarchical architecture (the polycrystal texture is uncontrollable in our and related studies).3,27 Our experiments indicate that the combined effect of multi-factors is able to create a huge difference in polymorphism. However, it is difficult to seek the whole picture in one case study. So, we encourage more efforts to investigate the cooperative effect of other factors on the morphological evolution, not only to enrich the polymorphism but also to clarify the mechanism, especially the unique functions of the pathway we highlighted in this work. Meanwhile, advanced characterization technologies are expected to in situ observe the formation process of biomorphs. After all, we haven’t known much at the fundamental level so far. We hope that based on an ingenious pathway design, biomorphs could self-evolve into a more intelligent and natural behavior, bringing us endless possibilities for morphologies and functions.
Experimental section
Preparation of “triangles”
The “triangles” were prepared by the gas diffusion method. Firstly, 0.330 mmol Na2SiO3·5H2O was dissolved well into 40 mL distilled deionized water (pretreatment with N2 bubbling for more than 2 hours to drive off pre-dissolved CO2) in a 100 mL beaker. Then, 0.760 mmol BaCl2·2H2O and 0.152 mmol MgCl2·6H2O (molar ratio, Mg2+/Ba2+ = 0.2) were dissolved into the Na2SiO3 solution in sequence. And the pH value of the solution was adjusted to 10.90 by dropping 0.1 M HCl (about 0.9 mL). After that, an aluminum plate (4 cm × 2 cm) was inserted into the solution as a growth substrate and the beaker was loosely covered by a Petri dish to allow CO2 to slowly diffuse into the solution. Then, the beaker was transferred into an air-dry oven with a set temperature of 30 °C to keep the temperature constant. After 10 hours, the aluminum plate was taken out and quickly immersed into distilled deionized water for 1 min to dilute the solution composition, and then it was immersed into acetone for 1 min to replace the surface water. Finally, the aluminum plate was dried in the air for 12 h and “triangles” would be found on the aluminum plate. Samples during the self-evolution process were taken out at the corresponding reaction time and went through exactly the same post-processing steps for further characterization.
Preparation of the derivative morphologies
The preparation procedure of the derivative morphologies is similar to the preparation procedure of “triangles”. For morphologies at different doping ratio of Mg2+, the molar ratio of Mg2+/Ba2+ was changed into 0.1 (0.083 mmol MgCl2·6H2O with 0.829 mmol BaCl2·2H2O), 0.3 (0.210 mmol MgCl2·6H2O with 0.702 mmol BaCl2·2H2O) and 0.4 (0.261 mmol MgCl2·6H2O with 0.651 mmol BaCl2·2H2O). For morphologies at different reaction temperatures, the temperatures of air-dry oven were set into 20 °C and 40 °C. With the selfsame gas diffusion method, and pre- and post-processing steps, the corresponding derivative morphologies would be found on the aluminum plate. Samples during the self-evolution process were taken out at the corresponding reaction time and went through exactly the same post-processing steps for further characterization.
Preparation of the previous biomorphs
The reported biomorphs were prepared according to the literature with some modifications.20 Firstly, 0.330 mmol Na2SiO3·5H2O was dissolved well into 40 mL distilled deionized water (pretreatment with N2 bubbling for more than 2 hours to drive off pre-dissolved CO2) in a 100 mL beaker. Then, 0.760 mmol BaCl2·2H2O was dissolved into the Na2SiO3 solution. And the pH value of the solution was adjusted by dropping 0.1 M HCl. For flat sheets, worms and helicoids, the pH value was adjusted to 11.20. And for corals, conical vases and stems, the pH value was adjusted to 11.80. After that, an aluminum plate (4 cm × 2 cm) was inserted into the solution as the growth substrate and the beaker was loosely covered by a Petri dish to allow CO2 to slowly diffuse into the solution. Then, the beaker was placed at room temperature. After 12 hours, the aluminum plate was taken out and quickly immersed into distilled deionized water for 1 min to dilute the solution composition, and then immersed into acetone for 1 min to replace the surface water. Finally, the aluminum plate was dried in the air for 12 h and the corresponding biomorphs could be found on the aluminum plate (Fig. 1A).
Scanning electron microscope (SEM) characterization
The morphological information of the biomorphs was characterized using SEM. Both a Hitachi X650 (low magnification observation, working voltage of 15 kV) and Zeiss Merlin compact (high magnification observation, working voltage between 3–5 kV) were used in this work. To prepare the SEM samples, the aluminum plate with samples was covered with gold by a magnetron sputterer (Shinkku VD, MSP-1S, gold disk targets). Then, the aluminum plate was adhered by conducting resin onto the sample stage of the SEM for observation.
Transmission electron microscope (TEM) characterization
The composition and crystallography information of the biomorphs were characterized using TEM. An FEI Talos F200X G2 (combined with FEI Super X energy dispersive spectrometer for EDX elemental mapping), JEOL JEM-2800 (combined with JEOL JED-2300T X energy dispersive spectrometer for EDX elemental mapping) and JEOL JEM-2100F were all used in this work. To prepare the TEM samples, the aluminum plates with samples were immersed in 3 mL ethanol and ultrasonicated for 10 minutes. The resulting suspension was added, dropwise, on the copper grids (EMCN, 200 mesh) several times and then dried in the air. For the characterization of the solution composition, 20 μL reaction solution at the corresponding reaction time was diluted with 3 mL ethanol and ultrasonicated for 10 minutes; then, the same operation procedure as the above was followed to prepare the sample.
Atomic force microscope (AFM) characterization
The surface information of the biomorphs was characterized under ambient conditions using a multimode scanning probe microscope (MicroNano D5A, Shanghai Cciinstrument Co. Ltd) in AFM tapping mode with a micro-cantilever (Mikromasch, CSC37/AlBS, 20–40 kHz, 0.3–0.8 N m−1). To prepare the AFM samples, the aluminum plate with samples was immersed in 3 mL of ethanol and ultrasonicated for 10 minutes. Then, about 0.15 mL of the resulting suspension was dropped onto the newly cleaved mica (SPI, Φ = 9.9 mm) and stored in a vacuum desiccator.
Schematic representation and false-colored SEM images
Schematic representations of the self-evolution process shown in Fig. 1D were originally designed using a Maxon Cinema 4D R19. The false-colored SEM images shown in Fig. 1E were post processed using Photoshop CC 2018. All the schemes and figures presented in this work were originally designed and created by the authors.
Author contributions
Y. C. conceptualized the project, directed the study, performed the AFM characterization and drew the graphs. S. W. prepared the samples, and performed most of the experiments and characterization. Y. C. and S. W. determined the experimental methods, analyzed and discussed the results, and wrote the manuscript together. Y. C. and S. W. contributed equally to this work. J. G. and J. W. acquired funding for the whole study. All authors commented on the manuscript.
Conflicts of interest
There are no conflicts to declare.
Acknowledgements
We thank Tingting Gong for assistance during the SEM and TEM investigations. We acknowledge the National Science Foundation of Tianjin (19JCZDJC37500), Innovative Group Project (21621004) and National Science Foundation of China (21938009).
Notes and references
- J. M. García-Ruiz, Carbonate precipitation into alkaline silica-rich environments, Geology, 1998, 26, 843–846 CrossRef.
- J. M. García-Ruiz, S. T. Hyde, A. M. Carnerup, A. G. Christy, M. J. Van Kranendonk and N. J. Welham, Self-assembled silica-carbonate structures and detection of ancient microfossils, Science, 2003, 302, 1194–1197 CrossRef.
- E. Nakouzi and O. Steinbock, Self-organization in precipitation reactions far from the equilibrium, Sci. Adv., 2016, 2, e1601144 CrossRef.
-
J. J. De Yoreo, Research Methods in Biomineralization Science, Elsevier, 2013 Search PubMed.
- J. M. García-Ruiz, A. Canerup, A. G. Christy, N. J. Welham and S. T. Hyde, Morphology: an ambiguous indicator of biogenicity, Astrobiology, 2002, 2, 353–369 CrossRef.
- J. M. García-Ruiz, E. Nakouzi, E. Kotopoulou, L. Tamborrino and O. Steinbock, Biomimetic mineral self-organization from silica-rich spring waters, Sci. Adv., 2017, 3, e1602285 CrossRef.
- K. S. Pérez and A. Moreno, Influence Of Pyruvic Acid And UV Radiation On The Morphology Of Silica-Carbonate Crystalline Biomorph, Crystals, 2019, 9, 67 CrossRef.
- C. N. Kaplan, W. L. Noorduin, L. Li, R. Sadza, L. Folkertsma, J. Aizenberg and L. Mahadevan, Controlled growth and form of precipitating microsculptures, Science, 2017, 355, 1395–1399 CrossRef CAS.
- L. Helmbrecht, M. Tan, R. Röhrich, M. H. Bistervels, B. O. Kessels, A. F. Koenderink, B. Kahr and W. L. Noorduin, Directed emission from self-assembled microhelices, Adv. Funct. Mater., 2018, 8, 1908218 Search PubMed.
- J. Opel, M. Kellermeier, A. Sickinger, J. Morales, H. Cölfen and J. M. García-Ruiz, Structural transition of inorganic silica-carbonate composites towards curved lifelike morphologies, Minerals, 2018, 8, 75 CrossRef.
- M. Kellermeier, E. Melero-García, W. Kunz and J. M. García-Ruiz, Local autocatalytic co-precipitation phenomena in self-assembled silica-carbonate materials, J. Colloid Interface Sci., 2012, 380, 1–7 CrossRef CAS.
- J. Eiblmeier, M. Kellermeier, D. Rengstl, J. M. García-Ruiz and W. Kunz, Effect of bulk pH and supersaturation on the growth behavior of silica biomorphs in alkaline solutions, CrystEngComm, 2013, 15, 43–53 RSC.
- E. Bittarello, F. R. Massaro and D. Aquilano, The epitaxial role of silica groups in promoting the formation of silica/carbonate biomorphs: a first hypothesis, J. Cryst. Growth, 2010, 312, 402–412 CrossRef CAS.
- J. Eiblmeier, U. Schürmann, L. Kienle, D. Gebauer, W. Kunz and M. Kellermeier, New insights into the early stages of silica-controlled barium carbonate crystallization, Nanoscale, 2014, 6, 14939–14949 RSC.
- J. Eiblmeier, S. Dankesreiter, A. Pfitzner, G. Schmalz, W. Kunz and M. Kellermeier, Crystallization of mixed alkaline-earth carbonates in silica solutions at high pH, Cryst. Growth Des., 2014, 14, 6177–6188 CrossRef CAS.
- E. Nakouzi, R. Rendina, G. Palui and O. Steinbock, Effect of inorganic additives on the growth of silica-carbonate biomorphs, J. Cryst. Growth, 2016, 452, 166–171 CrossRef CAS.
- A. E. Voinescu, M. Kellermeier, B. Bartel, A. M. Carnerup, A. K. Larsson, D. Touraud, W. Kunz, L. Kienle, A. Pfitzner and S. T. Hyde, Inorganic self-organized silica aragonite biomorphic composites, Cryst. Growth Des., 2008, 8, 1515–1521 CrossRef CAS.
- J. M. García-Ruiz, E. Melero-García and S. T. Hyde, Morphogenesis of self-assembled nanocrystalline materials of barium carbonate and silica, Science, 2009, 323, 362–365 CrossRef.
- G. Zhang, C. Verdugo-Escamilla, D. Choquesillo-Lazarte and J. M. García-Ruiz, Thermal assisted self-organization of calcium carbonate, Nat. Commun., 2018, 9, 5221 CrossRef CAS.
- W. L. Noorduin, A. Grinthal, L. Mahadevan and J. Aizenberg, Rationally designed complex, hierarchical microarchitectures, Science, 2013, 340, 832–837 CrossRef CAS.
- L. R. Monteiro and A. S. Abe, Functional and historical determinants of shape in the scapula of Xenarthran mammals: evolution of a complex morphological structure, J. Morphol., 1999, 241, 251–263 CrossRef CAS.
- W. Jiang, Z.-b. Qu, P. Kumar, D. Vecchio, Y. Wang, Y. Ma, J. H. Bahng, K. Bernardino, W. R. Gomes, F. M. Colombari, A. Lozada-Blanco, M. Veksler, E. Marino, A. Simon, C. Murray, S. R. Muniz, A. F. de Moura and N. A. Kotov, Emergence of complexity in hierarchically organized chiral particles, Science, 2020, 368, 642–648 CrossRef CAS.
- Y. Zhou, R. L. Marson, G. van Anders, J. Zhu, G. Ma, P. Ercius, K. Sun, B. Yeom, S. C. Glotzer and N. A. Kotov, Biomimetic hierarchical assembly of helical supraparticles from chiral nanoparticles, ACS Nano, 2016, 10, 3248–3256 CrossRef CAS.
- D. Gilmour, M. Rembold and M. Leptin, From morphogen to morphogenesis and back, Nature, 2017, 541, 311–320 CrossRef CAS.
- A. Grosberg, P. L. Kuo, C. L. Guo, N. A. Geisse, M. A. Bray, W. J. Adams, S. P. Sheehy and K. K. Parker, Self-organization of muscle cell structure and function, PLoS Comput. Biol., 2011, 7, e1001088 CrossRef CAS.
- M. R. Sawaya, S. Y. Guo, S. Tabor, C. C. Richardson and T. Ellenberger, Crystal structure of the helicase domain from the replicative helicase-primase of bacteriophage T7, Cell, 1999, 99, 167–177 CrossRef CAS.
- M. Kellermeier, H. Cölfen and J. M. García-Ruiz, Silica biomorphs: complex biomimetic hybrid materials from “sand and chalk”, Eur. J. Inorg. Chem., 2012, 5123–5144 CrossRef CAS.
- J. Eiblmeier, M. Kellermeier, M. Deng, L. Kienle, J. M. García-Ruiz and W. Kunz, Bottom-up self-assembly of amorphous core-shell-shell nanoparticles and biomimetic crystal forms in inorganic sili-ca-carbonate systems, Chem. Mater., 2013, 25, 1842–1851 CrossRef CAS.
-
D. L. Perry, Handbook of Inorganic Compounds, CRC press, 2016 Search PubMed.
- Z. Zhou and Y. Deng, Solution synthesis of magnesium hydroxide sulfate hydrate nanobelts using sparingly soluble carbonate salts as super-saturation control agents, J. Colloid Interface Sci., 2007, 316, 183–188 CrossRef CAS.
- X. Huang, G. Li, G. Sun, X. Dou, L. Li and L. Zheng, Initial growth of single-crystalline nanowires: from 3D nucleation to 2D growth, Nanoscale Res. Lett., 2010, 5, 1057–1062 CrossRef CAS.
-
L. Ratke and P. W. Voorhees, Growth and Coarsening: Ostwald Ripening in Material Processing, Springer Science & Business Media, 2013 Search PubMed.
- N. J. J. Johnson, A. Korinek, C. Dong and F. C. J. M. van Veggel, Self-focusing by Ostwald ripening: a strategy for layer-by-layer epitaxial growth on upconverting nanocrystals, J. Am. Chem. Soc., 2012, 134, 11068–11071 CrossRef CAS.
- M. Yang, T. Zhang, P. Schulz, Z. Li, G. Li, D. H. Kim, N. Guo, J. J. Berry, K. Zhu and Y. Zhao, Facile fabrication of large-grain CH3NH3PbI3-xBrx films for high-efficiency solar cells via CH3NH3Br-selective Ostwald ripening, Nat. Commun., 2016, 7, 12305 CrossRef CAS.
- L. Cera and C. A. Schalley, Under diffusion control: from structuring matter to directional motion, Adv. Mater., 2018, 30, 1707029 CrossRef.
- T. C. Halsey, Diffusion-limited aggregation: a model for pattern formation, Phys. Today, 2000, 53, 36–41 CrossRef CAS.
- R. F. Weiss, Carbon dioxide in water and seawater: the solubility of a non-ideal gas, Mar. Chem., 1974, 2, 203–215 CrossRef CAS.
- F. H. Arnold, Design by directed evolution, Acc. Chem. Res., 1998, 31, 125–131 CrossRef CAS.
- Y. Politi, D. R. Batchelor, P. Zaslansky, B. F. Chmelka, J. C. Weaver, I. Sagi, S. Weiner and L. Addadi, Role of magnesium ion in the stabilization of biogenic amorphous calcium carbonate: a structure-function investigation, Chem. Mater., 2010, 22, 161–166 CrossRef CAS.
- A. C. Montezano, D. Zimmerman, H. Yusuf, D. Burger, A. Z. Chignalia, V. Wadhera, F. N. van Leeuwen and R. M. Touyz, Vascular smooth muscle cell differentiation to an osteogenic phenotype involves TRPM7 modulation by magnesium, Hypertension, 2010, 56, 453–462 CrossRef CAS.
Footnotes |
† Electronic supplementary information (ESI) available: Materials, original SEM images, and relative intensity profile of elements (PDF). See DOI: 10.1039/d0qm00723d |
‡ These authors contributed equally to this work. |
|
This journal is © the Partner Organisations 2021 |