DOI:
10.1039/D0QM00597E
(Research Article)
Mater. Chem. Front., 2021,
5, 482-491
Cobalt induced growth of hollow MOF spheres for high performance supercapacitors†
Received
15th August 2020
, Accepted 21st October 2020
First published on 21st October 2020
Abstract
The development of highly-efficient metal organic framework (MOF) based supercapacitors has attracted much attention. In this work, hollow structure Ni/Co-MOFs have been facilely synthesized by using Co ions as structure-directing agents. Various structures of MOFs, such as hollow, yolk–shell and solid spheres, can be easily obtained through adjusting the molar ratio of Ni/Co. The introduction of an appropriate amount of Co ions can induce the formation of hollow spheres, where the hollow structure can offer rich redox reaction sites and provide fast transport pathways. Moreover, adsorption simulations and density of states calculations illustrate that the adsorbability of OH− and the electrochemical activity on the mixed-metallic MOFs are improved after introducing the Co ions, which can promote the reversible redox reaction activity of the electrode materials. The as-obtained hollow Ni/Co-MOF with the optimized amount of Co ions yields a high capacitance of 1498 F g−1 at 1 A g−1, an excellent rate capability of 67% capacitance retention at 30 A g−1 and a good cycling stability of 71% after 3000 cycles at 5 A g−1. This work may provide useful guidance for tuning the structure and electronic properties of MOFs to enhance the electrochemical performance for supercapacitors.
1. Introduction
Environment issues and the shortage of fossil energy have called for the exploitation of sustainable and clean energy sources.1 Therefore, the development of highly-efficient energy storage and conversion devices has attracted ever-increasing research interest.2–4 Supercapacitors, taking advantage of the properties of high power density, fast charge–discharge time and long cycle life, have been considered as one of the most promising candidates for use in electronic storage devices.5 The types of supercapacitors can be generally classified as electric double layer capacitance and pseudocapacitance depending on the energy storage mechanism. Compared with electric double layer materials, which store charge by the adsorption and desorption of ions, pseudocapacitive materials give a higher specific capacitance, owing to fast redox reactions between the electrode and electrolyte.6–8
Pseudocapacitive electrode materials for supercapacitors are mainly focused on transition-metal oxides/sulfides/phosphides, such as Co3O4,9 MoS2,10 and Co2P.11 In order to further improve the electrochemical performance, engineering the morphology of transition metal compounds is a vitally effective strategy. Hollow nanostructures with interior cavities and high surface-to-volume ratio can reduce the mass/charge-transport lengths and offer more accessible storage sites and sufficient contact area between the electrode and electrolyte. These features make them promising candidates for supercapacitors to improve the electrochemical performance.12,13 For example, the Lou group14 fabricated CoS hollow cubes through a self-template method, where the well-defined hollow CoS structure delivers a high capacitance of 980 F g−1 at current densities of 1 A g−1. The hollow CeO2–CuO spheres prepared by Ji et al. gave the best electrochemical performance among three types of as-made CeO2–CuO structures, indicating that hollow materials may be more suitable for supercapacitors than solid and yolk–shell materials.15 However, the inferior porosity of most transition-metal compounds constrains their advantages, resulting in limited capacitance.6 Therefore, the development of new materials for electrodes is a key strategy to improve the electrochemical performance of supercapacitors.
Metal organic frameworks (MOFs) are a new kind of porous materials consisting of metal ions and organic ligands.16 MOFs have been widely used in many aspects such as gas separation, catalysis, and energy storage/conversion due to their adjustable pore size, remarkable specific surface area, and abundant active sites.17–20 Recently, MOF-based materials have been investigated as electrode materials for supercapacitors. The Du group21 successfully synthesized a Ni-based MOF by the solvothermal method, exhibiting a good specific capacitance of 1057 F g−1 at a current density of 1 A g−1. The lamellae-like Ni/Co MOFs prepared by He et al.22 also exhibited a superior specific capacity and good rate performance with 80.1% retention at 10 A g−1. Therefore, the use of hollow MOFs as supercapacitor electrodes may take advantage of both the structure and composition of MOFs to enhance the electrochemical performance. However, there are also many challenges in preparing hollow structure MOFs. Until now, etching is a typical method for creating voids of MOFs. Hu et al. reported a strategy to prepare hollow ZIF-8 by using phenolic acid as an etching agent.23 Nevertheless, the development of etching of MOFs is still hindered by several problems: (i) the introduced voids are not well controlled and may lead to structural collapse of the MOFs; and (ii) the multi-step process of the top-down approach and the inevitable use of acidic reagents may cause a waste of time and environmental problems.24
Herein, we developed a novel strategy to fabricate hollow MOFs by using Co ions as inducers. The Co ions can induce the formation of hollow MOFs by regulating the chemical transformation processes. The partial substitution with Co ions in Ni-MOFs may result in an increase in the density of states around the Fermi level and the adsorption energy of OH−, and the synergistic effect of the Co and Ni components can boost the specific capacitance. Therefore, Ni/Co-MOFs with the advantages of hollow structure and fast multi-electron transfer faradaic processes show a high specific capacitance of 1498 F g−1 at 1 A g−1 and an excellent rate capability of 67% capacitance retention at 30 A g−1.
2. Experimental
2.1. Fabrication of the hollow sphere metal–organic framework (Ni/Co-MOF)
In a typical procedure, trimesic acid (0.75 mmol), polyvinylpyrrolidone (PVP 2 g), Co(NO3)2·6H2O (0.25 mmol) and Ni(NO3)2·6H2O (1.25 mmol) were dissolved in 30 mL of a mixture solution with a volume ratio of ethanol
:
DMF
:
water = 1
:
1
:
1 under stirring at room temperature. After stirring for 30 min, the mixture was then transferred into a 50 mL Teflon-lined autoclave and kept at 150 °C for 10 h. After the reaction, the products were centrifuged and washed with ethanol several times. After drying in a vacuum at 50 °C over 12 h, hollow Ni/Co-MOF (Ni/Co-MOF-1) spheres were obtained. For comparison, the mole ratio of Co2+
:
Ni2+ was set to 1
:
3 and 1
:
2, respectively, and the corresponding materials were denoted as Ni/Co-MOF-2 and Ni/Co-MOF-3, respectively. The pure Ni-MOF was synthesized through a similar process without the addition of Co(NO3)2·6H2O.
2.2. Material characterization
The morphologies and microstructures of the samples were analyzed using a field-emission scanning electron microscope (FESEM, Nova NanoSEM 450) equipped with an energy-dispersive spectrometry (EDS) accessory and a transmission electron microscope (TEM, Tecnai F20 system). Crystallographic data of the samples were obtained by X-ray diffraction (XRD, Shimadzu X-ray diffractometer). The different functional groups in the materials were determined by FT-IR. The valence states of the samples were measured by high resolution X-ray photoelectron spectroscopy (XPS, ESCALAB250Xi). Nitrogen sorption isotherms were measured at 77 K on a physical sorption instrument (Autosorb-iQ-C).
2.3. Electrochemical measurements
The electrochemical measurements of the samples were performed using a CHI 760E electrochemistry workstation using a typical three-electrode cell with a 6 M KOH electrolyte solution. For the three-electrode test, Pt foil was used as the counter electrode and a Hg/HgO electrode was used as the reference electrode. Films prepared by mixing the as-made MOFs, poly(tetrafluoroethylene) and carbon black with a mass ratio of 7
:
1
:
2 were used as working electrodes. The mass loading of the electrodes is around 1.5 mg for all the samples.
An asymmetric supercapacitor was assembled by using Ni/Co-MOF-1 as the positive electrode and active carbon as the negative electrode. The electrochemical performance was studied using a CHI 760E electrochemistry workstation in 6 M KOH electrolyte. To acquire optimized supercapacitors, the charges should be equilibrated by balancing the weight of the positive and negative active material,
in which
m+/
m−,
C+/
C−, and
V+/
V− denote the active material, specific capacity and potential window of the positive electrode and negative electrode, respectively.
2.4. Simulation details
The adsorption behaviors of OH− on the MOFs were studied using the Adsorption Locator program using the Materials Studio package with the universal force field. The simulation was performed with 100
000 loading steps and 3 heating cycles with 15
000 steps per cycle. The group based summation method was used with a cutoff distance of 12.5 Å and buffer width of 0.5 Å for the electrostatic terms. In the van der Waals terms, the atom based summation method and cubic spline truncation method were employed with a cutoff distance of 12.5 Å, spline width of 1 Å, and buffer width of 0.5 Å.
All theoretical calculations of the density of states (DOS) and partial density of states (PDOS) values for Ni-MOF and Ni/Co-MOF-1 were performed based on density functional theory (DFT) using the Cambridge Sequential Total Energy Package (CASTEP) program in the Materials Studio package. The generalized gradient approximation with the Perdew–Burke–Ernzerhof (PBE) functional was utilized to describe the electronic exchange and correlation effects. The self-consistent field tolerance was 2 × 10−6 eV with an on-the-fly generated ultrasoft pseudo-potential for core electrons.
3. Results and discussion
3.1. Synthesis and characterization of the Ni/Co-MOFs
The Ni-MOF and Ni/Co-MOFs are fabricated by a facile one-step hydrothermal method, and the schematic illustration of the fabrication process is shown in Fig. 1. The coordination of Ni2+ and trimesic acid (BTC) results in the Ni-MOF with a yolk–shell structure covered by some needles on the surface. Nevertheless, when Co2+ is introduced, hollow, yolk–shell, or solid spheres are formed with the change of the Co2+ content, suggesting that Co2+ plays an important role in influencing the structure of the MOFs.
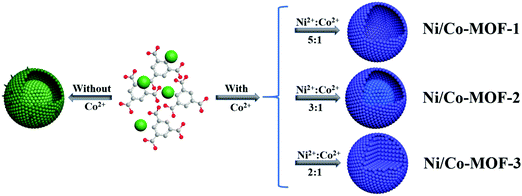 |
| Fig. 1 Schematic illustration of the fabrication routes of the Ni-MOF and Ni/Co-MOFs. | |
The morphologies of the Ni-MOFs and Ni/Co-MOFs were first observed by field-emission scanning electron microscopy (FESEM). Fig. 2a and b and Fig. S1a (ESI†) show that the Ni-MOF has a spherical structure with needles on the surface. The diameter of the spherical Ni-MOFs is around 3 μm. After introducing Co2+, the morphology change of Ni/Co-MOF-1 is visible, where the needles on the sphere surface disappeared, and the hollow structure of Ni/Co-MOF-1 can be found from the broken spheres and the thickness of the shell is about 300 nm. The corresponding energy-dispersive spectroscopy (EDS) elemental mapping images of Ni/Co-MOF-1 (Fig. S2, ESI†) reveal the uniform distribution of C, O, Ni and Co elements throughout the sample, indicating that the Co ions are successfully introduced into the MOFs. The internal void space of the hollow spheres can provide a sufficient contact area between the active sites and the electrolyte and reduce the diffusion length for ions and electrons to improve the electrochemical performance. When increasing the mole ratio of Co2+
:
Ni2+ to 1
:
3, surprisingly, the morphology of Ni/Co-MOF-2 is changed to a yolk–shell structure, where the core is fluffy (Fig. 2c and d and Fig. S1b, ESI†). When the mole ratio of Co2+ to Ni2+ is increased to 1
:
2 (Fig. 2g and h and Fig. S1d, ESI†), some of the spherical MOFs are aggregated into blocks, indicating that the amount of Co2+ is important for the regulation of the structure of the MOFs, which needs to be carefully controlled.
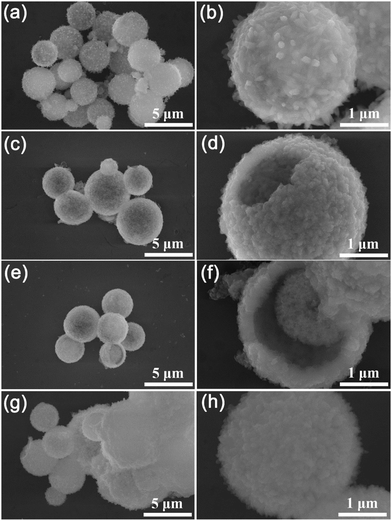 |
| Fig. 2 SEM images of the prepared MOFs under different magnifications: (a) and (b) Ni-MOF; (c) and (d) Ni/Co-MOF-1; (e) and (f) Ni/Co-MOF-2; and (g) and (h) Ni/Co-MOF-3. | |
In order to further investigate the internal structure of the Ni-MOFs and Ni/Co-MOFs, transmission electron microscopy (TEM) was used. As shown in Fig. 3a, the Ni-MOF has a yolk–shell structure with needles on the surface. In agreement with the FESEM findings, hollow structure Ni/Co-MOF-1 spheres were observed in Fig. 3b and Ni/Co-MOF-2 exhibits yolk–shell structured spheres (Fig. 3c). For Ni/Co-MOF-3, it can be found that the structure of the MOFs is further transformed into solid spheres (Fig. S3, ESI†). The above results indicate that the morphologies of the Ni/Co-MOFs can be easily fabricated by adjusting the mole ratio of Co2+ to Ni2+.
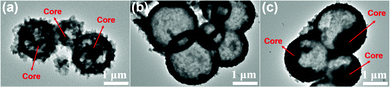 |
| Fig. 3 TEM images of the prepared MOFs: (a) Ni-MOF; (b) Ni/Co-MOF-1; and (c) Ni/Co-MOF-2. | |
The X-ray diffraction (XRD) patterns of all the MOFs are displayed in Fig. 4a. The diffraction patterns of the MOFs are similar to the MOFs synthesized with Ni ions and BTC linkers from other reports.25 Compared with the Ni-MOF, all the Ni/Co-MOFs give a higher degree of crystallization, suggesting that Co2+ may promote the crystallization process. The high degree of crystallization may enable a more stable frame structure for a rapid redox reaction to enhance the electrochemical performance.26 FT-IR is performed to investigate the components of the Ni-MOF and Ni/Co-MOFs; the results are shown in Fig. 4b. The peaks at around 724 cm−1, 763 cm−1, 1364 cm−1 and 1437 cm−1 for the MOFs are assigned to the C–H deformation vibration of aromatics. The additional peaks at around 1568 cm−1 and 1622 cm−1 are attributed to the stretching vibration of the coordinated carboxyl groups, referring to BTC linkers.15 The FT-IR peaks of the Ni-MOF and Ni/Co-MOFs are nearly the same, confirming that the as-made MOFs have similar chemical functional groups.
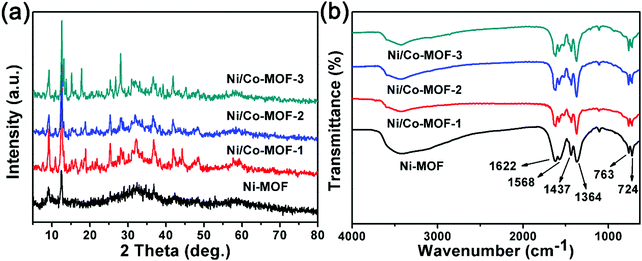 |
| Fig. 4 (a) XRD patterns and (b) FT-IR spectra of the as-made Ni-MOF and Ni/Co-MOFs. | |
X-Ray photoelectron spectroscopy (XPS) was further used to investigate the information of the components of the Ni-MOF and Ni/Co-MOFs. From Fig. S4a (ESI†), it can be found that the C, O and Ni elements are the main components of the Ni-MOF. Moreover, the additional Co element is clearly seen for all the Ni/Co-MOFs. For detailed information, the C 1s spectra of all the MOFs in Fig. S4b (ESI†) can be divided into two individual peaks, corresponding to the C
C bond (∼284.6 eV) and the carboxylate carbon (∼288.1 eV) of the BTC linkers.27 The high-resolution Co 2p and Ni 2p XPS spectra of Ni/Co-MOF-1 in Fig. 5 are well fitted with two spin–orbit doublets and two shakeup satellites. The peaks at 856.1 eV and 873.7 eV can be assigned to Ni 2p1/2 and Ni 2p3/2 and the spin-energy separation is due to the spin–orbit characteristic of Ni2+.28 For the Co 2p XPS spectra, the Co 2p3/2 and Co 2p1/2 peaks are located at a binding energy of 797.3 eV and 781.3 eV accompanied by another two shake-up satellite peaks, respectively, indicating the presence of Co2+ (Fig. 5b).29 Furthermore, Fig. S4c and d (ESI†) show that the Co 2p and Ni 2p XPS spectra of the Ni-MOF, Ni/Co-MOF-2 and Ni/Co-MOF-3 are similar to those of Ni/Co-MOF-1, indicating both Ni and Co elements in the form of a divalent state. The Ni2+ and Co2+ species as redox centers will play an important role in achieving high pseudocapacitance.30 In addition, the Ni/Co molar ratio of Ni/Co-MOF-1, Ni/Co-MOF-2 and Ni/Co-MOF-3 measured by XPS is 5.04, 2.96 and 2.17, respectively, which correspond with the theoretical ratios. The Brunauer–Emmett–Teller surface areas of the Ni-MOF and Ni/Co-MOFs were analyzed by the nitrogen sorption technique, and the results are shown in Fig. S5 (ESI†). The surface area of Ni/Co-MOF-1 is about 68 m2 g−1, which is higher than that of the Ni-MOF (42 m2 g−1), Ni/Co-MOF-2 (56 m2 g−1) and Ni/Co-MOF-3 (40 m2 g−1); this may be helpful for Ni/Co-MOF-1 to achieve good capacitive performance.
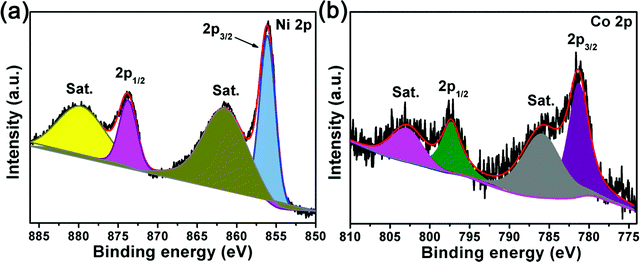 |
| Fig. 5 High-resolution XPS Ni 2p spectra (a) and Co 2p spectra (b) of Ni/Co-MOF-1. | |
3.2. Electrochemical performance of the Ni-MOF and Ni/Co-MOFs
The electrochemical performance of the Ni-MOF and Ni/Co-MOF electrodes for supercapacitors was first analyzed using a three-electrode cell in 6 M KOH aqueous electrolyte. Fig. 6a shows the cyclic voltammograms (CVs) of the Ni-MOF and Ni/Co-MOFs tested at a scan rate of 5 mV s−1; it can be observed that all CV curves exhibit pairs of broad redox couple peaks, indicating a typical pseudocapacitance behavior. Fig. S6 (ESI†) shows the CV curves of the Ni-MOF and Ni/Co-MOF-1 measured at scan rates from 5 mV s−1 to 50 mV s−1; a large deformation of the CV curve can be found for the Ni-MOF while that of Ni/Co-MOF-1 maintains the shape containing the redox peaks, suggesting an improved rate capability of Ni/Co-MOF-1. The galvanostatic charge/discharge (GC) curves of the MOFs are nonlinear with plateaus at a current density of 1 A g−1 (Fig. 6b), indicating the significant contribution of the redox reaction, which agrees well with the results of the CV curves. Ni/Co-MOF-1 exhibits the largest CV areas and longest discharge time, suggesting its highest specific capacitance. The specific capacitances of all the MOF electrodes calculated using the results of the GC curves are shown in Fig. 6c. The Ni-MOF possesses a specific capacitance of 1009 F g−1 at a current density of 1 A g−1. When the current density is increased up to 30 A g−1, the capacitance retention is only 14% (146 F g−1). The capacitance decay may be attributed to the low conductivity and the insufficient redox reactions between the surface active sites of the MOF and electrolyte at the high current density. Nevertheless, after introducing Co2+, all the Ni/Co-MOFs show increased specific capacitances, which can reach 1498 F g−1 (Ni/Co-MOF-1), 1244 F g−1 (Ni/Co-MOF-2) and 1065 F g−1 (Ni/Co-MOF-3) at 1 A g−1, respectively. The electrochemical performance of Ni/Co-MOF-1 is comparable to the recently reported MOF-based electrodes (Table S1, ESI†).20,30–37 The Ni/Co-MOFs also show better rate capability of 67% (Ni/Co-MOF-1), 65% (Ni/Co-MOF-2) and 47% (Ni/Co-MOF-3) at a high current density of 30 A g−1 than the capacitance retention of the Ni-MOF. The incorporation of Co2+ may improve the electronic conductivity of the active materials, leading to better reaction kinetics. The effects of the introduction of Co2+ into the Ni-MOF on the charge transport and ion diffusion properties were investigated by electrochemical impedance spectroscopy (EIS) measured in the frequency range from 10−2 to 105 Hz. The Nyquist plots of the various Ni-MOF and Ni/Co-MOFs are presented in Fig. 6d. The equivalent electrical circuit model in Fig. S7 (ESI†) was used for EIS data fitting. For the equivalent circuit, Rs is the ohmic resistance of the system, Cd corresponds to the double layer capacitance, Rct corresponds to the charge transfer resistance and QA corresponds to the adsorption of OH− onto the electrode surfaces. The values of the equivalent circuit elements are summarized in Table S2 (ESI†). The semicircle of the Nyquist plot in the high-frequency range corresponds to the charge transfer resistance, which is associated with the surface properties of the electrode.38 The diameters of the semicircles of the Ni/Co-MOFs are smaller than that of the Ni-MOF, indicative of the faster charge transport, which may be beneficial to electrochemical reactions. It is worth noting that the charge transfer resistance (Rct) of Ni/Co-MOF-3 containing solid spheres is still smaller than that of the yolk–shell Ni-MOF, indicating that the Ni/Co-MOFs with Co2+ can offer easy diffusion of the electrolyte and faster charge transportation. Specifically, the hollow Ni/Co-MOF-1 exhibits the lowest Rct and ohmic resistance of the system (Rs), which is beneficial for fast ion/charge transport to improve the performance of the as prepared materials.
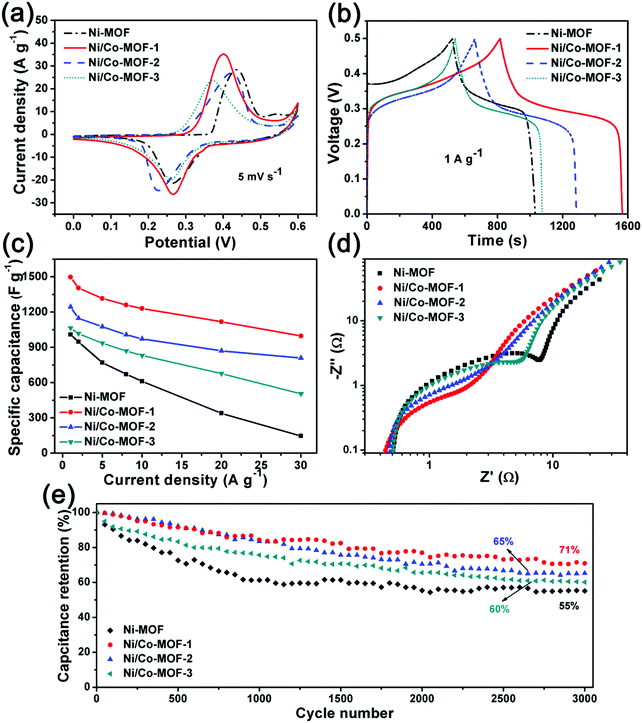 |
| Fig. 6 Electrochemical performance of the as-made Ni-MOF and Ni/Co-MOFs for supercapacitors: (a) CV curves at scan rates of 5 mV s−1; (b) galvanostatic charge–discharge curves at a current density of 1 A g−1; (c) specific capacitances at different current densities; (d) Nyquist plots; and (e) cycling performance at a current density of 5 A g−1. | |
The cycling stability of all the MOFs is shown in Fig. 6e. The Ni-MOF delivers about 55% capacitance retention after 3000 charge–discharge cycles at a current density of 5 A g−1. However, all the Ni/Co-MOF electrodes show improved cycling stability. The hollow Ni/Co-MOF-1 can maintain 71% of its original capacitance after 3000 cycles, which is higher than those of Ni/Co-MOF-2 (65%) and Ni/Co-MOF-3 (60%), indicative of good cycling performance.
Some previous studies have reported that the incorporation of second central metal ions into organic frameworks can significantly improve the electrochemical performance,30,31,39 but this improvement mechanism is still under discussion. To further understand the advantages of Ni/Co-MOFs, we carried out theoretical calculations to investigate the effect of introduction of Co2+ on the electronic structure.
The density of states (DOS) of the Ni-MOF and Ni/Co-MOF-1 is displayed in Fig. 7a and b. The continuous distribution of the DOS of the Ni-MOF and Ni/Co-MOF-1 near the Fermi level indicates that the Ni-MOF and Ni/Co-MOF-1 are in metallic states with high electrical conductivity, which facilitates fast electron transfer in the electrochemical processes. Moreover, it can be found that the electronic properties of Ni/Co-MOF-1 changed significantly in comparison with the Ni-MOF; more electron states around the Fermi level are favorable for achieving higher electronic conductivity, ensuring enhanced electronic conductivity and electrochemical reactivity of Ni/Co-MOF-1 for boosting the redox reactions. The continuous distribution of the partial density of states (PDOS) shown in Fig. S8 (ESI†) further confirms that the Ni-MOF and Ni/Co-MOF-1 are in metallic states. The higher density of states based on the s and p atomic orbitals demonstrates the higher electronic conductivity and electrochemical activity of Ni/Co-MOF-1. The adsorption simulations in Fig. 7c and d show that the incorporation of Co2+ facilitates the adsorption of OH− ions on Ni/Co-MOF-1 (−5.02 eV) compared to that of Ni-MOF (−3.75 eV), which may be favorable for more active sites to participate in the redox reaction. Both the adsorption simulation and density of states calculations reveal that incorporation of Co2+ plays a crucial role in the electrochemical reactivity and electrical conductivity enhancement for the electrochemical reactivity of Ni-MOF based materials.
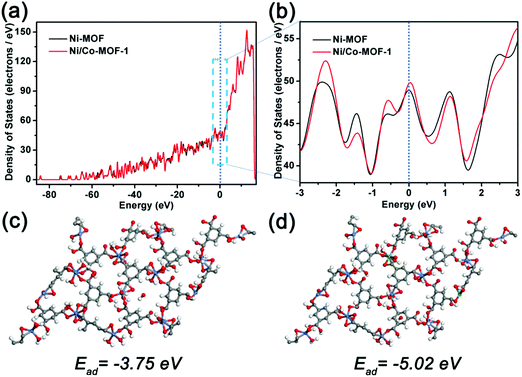 |
| Fig. 7 (a) and (b) DOS of the Ni-MOF and Ni/Co-MOF-1 and optimized binding energies of OH− on the Ni-MOF (c) and Ni/Co-MOF-1 (d). | |
Therefore, the best electrochemical performance of Ni/Co-MOF-1 may be due to the synergistic effect of the unique hollow structure and the mixed metal composition. The hollow structure spheres can reduce the charge transport length and provide rich redox reaction sites. The optimized amount of added Co2+ can exhibit low charge transfer resistance, increased adsorption energy of OH− ions and high electrochemical activity, which may enhance the reversible redox reactivity and improve the reaction kinetics in the electrode materials.
To investigate the potential practical application as electrode materials, the synthesized Ni/Co-MOF-1 was studied in two-electrode cell tests in 6 M KOH electrolyte with Ni/Co-MOF-1 as the positive electrode and commercial active carbon (AC) as the negative electrode. The voltage range of 0–1.6 V has been chosen as the used electrochemical window because the Ni/Co-MOF-1 electrode can be measured in the voltage range of 0–0.6 V while the AC electrode is evaluated in the voltage range of −1.0 to 0 V at a scan rate of 10 mV s−1 (Fig. S9, ESI†). Fig. 8a shows the CV curves of Ni/Co-MOF-1//AC at scan rates from 5 to 100 mV s−1 and Fig. 8b displays the GC profiles of Ni/Co-MOF-1//AC at different current densities from 1 to 10 A g−1. The quasi-rectangular geometry of the CV curves and the nonlinear curves of the GC profiles suggest that the capacitance of Ni/Co-MOF-1//AC results from both pseudocapacitance and electric double-layer capacitance. But Ni/Co-MOF-1//AC also exhibits a low coulombic efficiency, which may be because the high binding energy of OH− to Ni/Co-MOF-1 is harmful for the desorption of ions. The specific capacitances calculated based on the GC curves at different current densities are presented in Fig. 8c. Ni/Co-MOF-1//AC exhibits a high specific capacitance of ca. 157 F g−1 at a current density of 1 A g−1, and 65% initial capacitance can be retained at a high current density of 10 A g−1. The Ragone plot in Fig. 8d shows that Ni/Co-MOF-1//AC has a high energy density of 56 W h kg−1 at a power density of 0.8 kW kg−1; when the power density is increased up to 8.0 kW kg−1, the energy density can still maintain 36 W h kg−1. The electrochemical performance of Ni/Co-MOF-1//AC is comparable to the recently reported asymmetric supercapacitors (Table S3, ESI†).7,14,20,40–44 The cycling performance of the Ni/Co-MOF-1//AC asymmetric supercapacitor was also investigated at a current density of 3 A g−1. Fig. 6e shows that Ni/Co-MOF-1//AC can retain ca. 61% of the initial response after 4000 cycles, indicating good cycling stability. In order to further improve the cycling stability, we decrease the voltage window to 0–1.5 V, and it can be found that Ni/Co-MOF-1//AC can maintain ca. 62% of the initial capacity after 6000 cycles, indicating that the cycling performance is improved significantly (Fig. S10, ESI†).
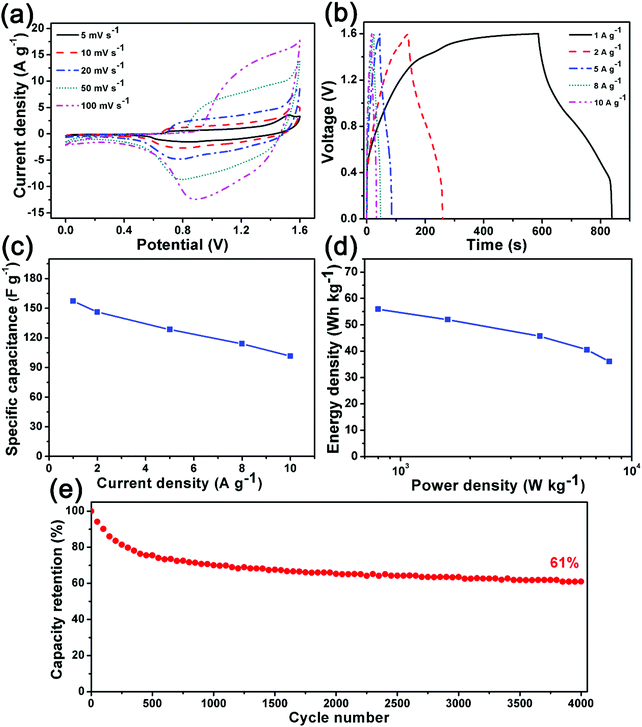 |
| Fig. 8 Electrochemical performance of the as-fabricated Ni/Co-MOF-1//AC asymmetric supercapacitor: (a) CV curves at scan rates from 5 to 100 mV s−1; (b) galvanostatic charge/discharge curves at various current densities of 1–10 A g−1; (c) the corresponding specific capacitance at different current densities; (d) the Ragone plots of the as-fabricated Ni/Co-MOF-1//AC; and (e) cycling performance at a current density of 3 A g−1. | |
4. Conclusions
In summary, hollow Ni/Co-MOF spheres have been prepared by a facile one-step hydrothermal method with adjusting the Ni2+/Co2+ ratio. The amount of Co2+ can influence the structure of the MOFs significantly, where the appropriate amount of Co ions can induce the formation of hollow structure MOF spheres. Furthermore, the introduction of Co2+ can reduce the charge transfer resistance, increase the adsorption energy of OH− ions and improve the electrochemical activity. Benefiting from the synergistic effect of the unique hollow structure and the electronic properties, the prepared Ni/Co-MOF-1 electrode can achieve excellent performance of 1498 F g−1 at a current density of 1 A g−1 and 67% capacitance retention at 30 A g−1. Moreover, the Ni/Co-MOF-1//AC asymmetric supercapacitor yields a high energy density of 56 W h kg−1 at a power density of 0.8 kW kg−1. This work may provide a facile strategy to tune the structure and electronic properties of MOFs for high-performance supercapacitors.
Conflicts of interest
The authors declare no competing financial interest.
Acknowledgements
This work is supported by the China Postdoctoral Science Foundation (No. 2018M631168), the Fundamental Research Funds for the Central Universities (DUT20RC(4)020, DUT20LK44), National Natural Science Foundation of China (21902021), the Joint Research Fund Liaoning-Shenyang National Laboratory for Materials Science (20180510020), the Natural Science Foundation of Liaoning Province (2019-ZD-0020) and Supercomputing Center of Dalian University of Technology.
References
- A. M. Liu, M. F. Gao, X. F. Ren, F. N. Meng, Y. N. Yang, L. G. Gao, Q. Y. Yang and T. L. Ma, Current progress in electrocatalytic carbon dioxide reduction to fuels on heterogeneous catalysts, J. Mater. Chem. A, 2020, 8, 3541–3562 RSC
.
- D. W. Feng, T. Lei, M. R. Lukatskaya, J. Park, Z. H. Huang, M. Lee, L. Shaw, S. C. Chen, A. A. Yakovenko, A. Kulkarni, J. P. Xiao, K. Fredrickson, J. B. Tok, X. D. Zou, Y. Cui and Z. A. Bao, Robust and conductive two-dimensional metal-organic frameworks with exceptionally high volumetric and areal capacitance, Nat. Energy, 2018, 3, 30–36 CrossRef CAS
.
- L. Tang, X. G. Meng, D. H. Deng and X. H. Bao, Confinement catalysis with 2D materials for energy conversion, Adv. Mater., 2019, 31, 1901996 CrossRef CAS
.
- H. W. Huang, C. Yu, C. T. Zhao, X. T. Han, J. Yang, Z. B. Liu, S. F. Li, M. D. Zhang and J. S. Qiu, Iron-tuned super nickel phosphide microstructures with high activity for electrochemical overall water splitting, Nat. Energy, 2017, 34, 472–480 CAS
.
- X. Y. Shi, S. H. Zheng, Z.-S. Wu and X. H. Bao, Recent advances of graphene-based materials for high-performance and new-concept supercapacitors, J. Energy Chem., 2018, 27, 25–42 CrossRef
.
- Y. J. Zhao, J. Z. Liu, M. Horn, N. Motta, M. J. Hu and Y. Li, Recent advancements in metal organic framework based electrodes for supercapacitors, Sci. China Mater., 2018, 61, 159–184 CrossRef CAS
.
- X. Zhang, Q. Y. Fan, S. Y. Liu, N. Qu, H. Yang, M. Wang and J. Yang, A facile fabrication of 1D/2D nanohybrids composed of NiCo-hydroxide nanowires and reduced graphene oxide for high-performance asymmetric supercapacitors, Inorg. Chem. Front., 2020, 7, 204–211 RSC
.
- X. Zhang, Q. Y. Fan, N. Qu, H. Yang, M. Wang, A. M. Liu and J. Yang, Ultrathin 2D nitrogen-doped carbon nanosheets for high performance supercapacitors: insight into the effects of graphene oxides, Nanoscale, 2019, 11, 8588–8596 RSC
.
- Y.-Z. Zhang, Y. Wang, Y.-L. Xie, T. Cheng, W.-Y. Lai, H. Pang and W. Huang, Porous hollow Co3O4 with rhombic dodecahedral structures for high-performance supercapacitors, Nanoscale, 2014, 6, 14354–14359 RSC
.
- S. C. Zhang, R. R. Hu, P. Dai, X. X. Yu, Z. L. Ding, M. Z. Wu, G. Li, Y. Q. Ma and C. J. Tu, Synthesis of rambutan-like MoS2/mesoporous carbon spheres nanocomposites with excellent performance for supercapacitors, Appl. Surf. Sci., 2017, 396, 994–999 CrossRef CAS
.
- X. J. Chen, M. Cheng, D. Chen and R. M. Wang, Shape-controlled synthesis of Co2P nanostructures and their application in supercapacitors, ACS Appl. Mater. Interfaces, 2016, 8, 3892–3900 CrossRef CAS
.
- T. Liu, L. Y. Zhang, B. Cheng and J. G. Yu, Hollow carbon spheres and their hybrid nanomaterials in electrochemical energy storage, Adv. Energy Mater., 2019, 9, 1803900 CrossRef
.
- L. Zhou, Z. C. Zhuang, H. H. Zhao, M. T. Lin, D. Y. Zhao and L. Q. Mai, Intricate hollow structures: controlled synthesis and applications in energy storage and conversion, Adv. Mater., 2017, 29, 1602914 CrossRef
.
- H. Hu, B. Y. Guan and X. W. Lou, Construction of complex CoS hollow structures with enhanced electrochemical properties for hybrid supercapacitors, Chem, 2016, 1, 102–113 CAS
.
- Y. J. Ji, Z. Y. Jin, J. Li, Y. Zhang, H. Z. Liu, L. S. Shi, Z. Y. Zhong and F. B. Su, Rambutan-like hierarchically heterostructured CeO2–CuO hollow microspheres: facile hydrothermal synthesis and applications, Nano Res., 2017, 10, 381–396 CrossRef CAS
.
- D. Sheberla, J. C. Bachman, J. S. Elias, C.-J. Sun, Y. Shao-Horn and M. Dinca, Conductive MOF electrodes for stable supercapacitors with high areal capacitance, Nat. Mater., 2017, 16, 220–224 CrossRef CAS
.
- A. Indra, T. Song and U. Paik, Metal organic framework derived materials: progress and prospects for the energy conversion and storage, Adv. Mater., 2018, 30, 1705146 CrossRef
.
- R.-B. Lin, L. B. Li, H.-L. Zhou, H. Wu, C. H. He, S. Li, R. Krishna, J. P. Li, W. Zhou and B. L. Chen, Molecular sieving of ethylene from ethane using a rigid metal-organic framework, Nat. Mater., 2018, 17, 1128–1133 CrossRef CAS
.
- D. S. Raja, H.-W. Lin and S.-Y. Lu, Synergistically well-mixed MOFs grown on nickel foam as highly efficient durable bifunctional electrocatalysts for overall water splitting at high current densities, Nano Energy, 2019, 57, 1–13 CrossRef
.
- X. Zhang, N. Qu, S. X. Yang, Q. Y. Fan, D. Lei, A. M. Liu and X. Chen, Shape-controlled synthesis of Ni-based metal–organic frameworks with albizia flower-like spheres@nanosheets structure for high performance supercapacitors, J. Colloid Interface Sci., 2020, 575, 347–355 CrossRef CAS
.
- P. C. Du, Y. N. Dong, C. Liu, W. L. Wei, D. Liu and P. Liu, Fabrication of hierarchical porous nickel based metal–organic framework (Ni-MOF) constructed with nanosheets as novel pseudo-capacitive material for asymmetric supercapacitor, J. Colloid Interface Sci., 2018, 518, 57–68 CrossRef CAS
.
- X. L. Zhang, J. M. Wang, X. Ji, Y. W. Sui, F. X. Wei, J. Q. Qi, Q. K. Meng, Y. J. Ren and Y. Z. He, Nickel/cobalt bimetallic metal–organic frameworks ultrathin nanosheets with enhanced performance for supercapacitors, J. Alloys Compd., 2020, 825, 154069 CrossRef CAS
.
- M. Hu, Y. Ju, K. Liang, T. Suma, J. W. Cui and F. Caruso, Void engineering in metal–organic frameworks via synergistic etching and surface functionalization, Adv. Funct. Mater., 2016, 26, 5827–5834 CrossRef CAS
.
- C. Avci, A. Yazdi, M. Tarres, E. Bernoud, N. G. Bastus, V. Puntes, I. Imaz, X. Ribas and D. Maspoch, Sequential deconstruction-reconstruction of metal–organic frameworks: an alternative strategy for synthesizing (multi)-layered ZIF composites, ACS Appl. Mater. Interfaces, 2018, 10, 23952–23960 CrossRef CAS
.
- F. Zou, Y.-M. Chen, K. W. Liu, Z. T. Yu, W. F. Liang, S. M. Bhaway, M. Gao and Y. Zhu, Metal Organic Frameworks Derived Hierarchical Hollow NiO/Ni/Graphene Composites for Lithium and Sodium Storage, ACS Nano, 2016, 10, 377–386 CrossRef CAS
.
- P. Wan, H. Xie, N. Zhang, S. Zhu, C. D. Wang, Z. Yu, W. S. Chu, L. Song and S. Q. Wei, Stepwise hollow prussian blue nanoframes/carbon nanotubes composite film as ultrahigh rate sodium ion cathode, Adv. Funct. Mater., 2020, 30, 2002624 CrossRef CAS
.
- X. Zhang, N. Qu, Q. Y. Fan and H. Yang, Surface functionalization of graphene oxide with DBU as electrode materials for supercapacitors, Mater. Res. Express, 2019, 6, 085606 CrossRef CAS
.
- Y. Y. Zhao, N. Jiang, X. Zhang, J. Guo, Y. Q. Li, L. G. Gao, H. X. Wang and T. L. Ma, Low-cost, large-scale, one-pot synthesis of C/Ni3(NO3)2(OH)4 composites for high performance supercapacitor, Mater. Chem. Phys., 2018, 217, 291–299 CrossRef CAS
.
- D. Zhang, H. Wang, G. Chen, H. Wan, N. Zhang, X. H. Liu and R. Z. Ma, Post-synthesis isomorphous substitution of layered Co–Mn hydroxide nanocones with graphene oxide as high-performance supercapacitor electrodes, Nanoscale, 2019, 11, 6165–6173 RSC
.
- S. W. Gao, Y. W. Sui, F. X. Wei, J. Q. Qi, Q. K. Meng, Y. J. Ren and Y. Z. He, Dandelion-like nickel/cobalt metal–organic framework based electrode materials for high performance supercapacitors, J. Colloid Interface Sci., 2018, 531, 83–90 CrossRef CAS
.
- H. Gholipour-Ranjbar, M. Soleimani and H. R. Naderi, Application of Ni/Co-based metal–organic frameworks (MOFs) as an advanced electrode material for supercapacitors, New J. Chem., 2016, 40, 9187–9193 RSC
.
- S. F. Zhao, L. Z. Zeng, G. Cheng, L. Yu and H. Zeng, Ni/Co-based metal–organic frameworks as electrode material for high performance supercapacitors, Chinese, Chem. Lett., 2019, 30, 605–609 CAS
.
- S. Sanati, R. Abazari, A. Morsali, A. M. Kirillov, P. C. Junk and J. Wang, An asymmetric supercapacitor based on a non-calcined 3D pillared cobalt(II) metal–organic framework with long cyclic stability, Inorg. Chem., 2019, 58, 16100–16111 CrossRef CAS
.
- W. L. Xuan, R. Ramachandran, C. H. Zhao and F. Wang, Influence of synthesis temperature on cobalt metal–organic framework (Co-MOF) formation and its electrochemical performance towards supercapacitor electrodes, J. Solid State Electrochem., 2018, 22, 3873–3881 CrossRef CAS
.
- R. Rajak, M. Saraf and S. M. Mobin, Mixed-ligand architected unique topological heterometallic sodium/cobalt-based metal–organic framework for high-performance supercapacitors, Inorg. Chem., 2020, 59, 1642–1652 CrossRef CAS
.
- M. G. Radhika, B. Gopalakrishna, K. Chaitra, L. K. G. Bhatta, K. Venkatesh, M. K. S. Kamath and N. Kathyayini, Electrochemical studies on Ni, Co & Ni/Co-MOFs for high-performance hybrid supercapacitors, Mater. Res. Express, 2020, 7, 054003 CrossRef CAS
.
- D. Tian, X. F. Lu, Y. Zhu, M. X. Li and C. Wang, Fabrication of two-dimensional metal–organic frameworks on electrospun nanofibers and their derived metal doped carbon nanofibers for an advanced asymmetric supercapacitor with a high energy density, J. Power Sources, 2019, 413, 50–58 CrossRef CAS
.
- J. Yang, C. Yu, X. M. Fan and J. S. Qiu, 3D Architecture Materials Made of NiCoAl-LDH Nanoplates Coupled with NiCo-Carbonate Hydroxide Nanowires Grown on Flexible Graphite Paper for Asymmetric Supercapacitors, Adv. Energy Mater., 2014, 4, 1400761 CrossRef
.
- Y. Jiao, J. Pei, D. H. Chen, C. S. Yan, Y. Y. Hu, Q. Zhang and G. Chen, Mixed-metallic MOF based electrode materials for high performance hybrid supercapacitors, J. Mater. Chem. A, 2017, 5, 1094–1102 RSC
.
- J. X. Dong, S. J. Li and Y. Ding, Anchoring nickel-cobalt sulfide nanoparticles on carbon aerogel derived from waste watermelon rind for high-performance asymmetric supercapacitors, J. Alloys Compd., 2020, 845, 155701 CrossRef CAS
.
- S. J. Hou, X. T. Xu, M. Wang, Y. Q. Xu, T. Lu, Y. F. Yao and L. K. Pan, Carbon-incorporated Janus-type Ni2P/Ni hollow spheres for high performance hybrid supercapacitors, J. Mater. Chem. A, 2017, 5, 19054–19061 RSC
.
- Y. Zhao, M. Zhao, X. Ding, Z. R. Liu, H. Tian, H. H. Shen, X. T. Zu, S. A. Li and L. Qiao, One-step colloid fabrication of nickel phosphides nanoplate/nickel foam hybrid electrode for high-performance asymmetric supercapacitors, Chem. Eng. J., 2019, 373, 1132–1143 CrossRef CAS
.
- R. B. Rakhi, N. A. Alhebshi, D. H. Anjum and H. N. Alshareef, Nanostructured cobalt sulfide-on-fiber with tunable morphology as electrodes for asymmetric hybrid
supercapacitors, J. Mater. Chem. A, 2014, 2, 16190–16198 RSC
.
- M. Feng, J. M. Gu, G. C. Zhang, M. Xu, Y. N. Yu, X. Liu, Z. Wang, B. P. Yin, Y. W. Liu and S. M. Liu, Homogeneous nickel bicarbonate nanocrystals as electrode materials for high-performance asymmetric supercapacitors, J. Solid State Chem., 2020, 282, 121084 CrossRef CAS
.
Footnote |
† Electronic supplementary information (ESI) available. See DOI: 10.1039/d0qm00597e |
|
This journal is © the Partner Organisations 2021 |