DOI:
10.1039/D0QM00653J
(Research Article)
Mater. Chem. Front., 2021,
5, 458-464
Silica-supported dual-dye nanoprobes for ratiometric hypoxia sensing†
Received
31st August 2020
, Accepted 15th October 2020
First published on 16th October 2020
Abstract
Oxygen (O2) levels provide an important parameter in the early-stage diagnosis of cancers and other diseases. Ratiometric optical O2 sensing has attracted much interest due to its better calibration of oxygen levels at two wavelengths. Oxygen sensing nanoprobes are generally designed by the incorporation of a reference dye and phosphorescent indicator into various matrixes. Silica nanomaterials with intriguing characteristic properties are highly preferable in hypoxia sensing. However, physical entrapment of dyes in silica suffers from leaching and moderate sensitivity. Reported herein is a covalently encapsulated dual-dye silica nanoprobe for ratiometric hypoxia detection and imaging. The nanoprobe prepared in a one-pot approach has a core–shell nanostructure where silanized indicators and reference dyes are covalently grafted within the silica core, while poly(ethylene glycol) chains form an outer shell. To our delight, through the covelent bonding of the dyes for efficient energy transfer between the reference dye and the indicator, high phosphorescence brightness, long lifetime and excellent stability of the nanoprobe were achieved. More importantly, the designed nanoprobe can respond to different oxygen levels in both solution and living cells with impressive sensitivity and stability.
Introduction
Molecular oxygen (O2), one of the essential elements of all known higher-organisms, plays a vital role in living systems. Detecting and imaging O2 levels in living cells represent an important and challenging issue in the biomedical field.1–6 The lack of O2, named as hypoxia, often provides an important parameter in the early-stage diagnosis of cancers and other diseases. It has been well established that quenching phosphorescence by O2 is a simple, noninvasive method for imaging in vivo O2 levels.7–12 Transition-metal complexes such as Pt/Pd porphyrins have been widely employed as oxygen probes. Long lifetime and high quantum yield of the phosphorescence ensure the detection of oxygen at low levels. However, the utility of small molecules for this application is hampered by their poor water solubility, nonspecific binding, self-aggregation, and unfavorable photophysical properties. To address these issues, luminescent porphyrins were incorporated into various scaffolds for the design of oxygen nanoprobes. In particular, the co-encapsulations of the reference dye and phosphorescent indicator into the matrixes enable ratiometric optical O2 sensing with better calibration of the oxygen levels at two wavelengths.9–13
Silica nanomaterials are characteristic of optical transparency, photophysical inertness, chemical durability and biocompatibility, and are highly preferable for hypoxia sensing.14–16 Immobilization of detection components in the supportive matrix is a critical step in the fabrication of silica-based optical sensors.17 Many efforts have been devoted to physical entrapment owing to its simple preparation. However, sensors designed in these ways suffer from leaching of the dyes out of the matrix and moderate sensitivity.
Motivated by our long-term interests in hypoxia sensing,12a–c we attempted to design a ratiometric dual-dye covalently-bonded hypoxia silica nanoprobe. This probe has a core–shell nanostructure, in which a silanized oxygen sensitive indicator and oxygen-insensitive reference dye are covalently grafted within the silica core, while poly(ethylene glycol) (PEG) chains from Pluronic F-127 form an outer shell. The nanoprobe possesses preferable small particle size (10 ± 2 nm), excellent water-dispersibility, low biotoxicity and membrane-penetration ability. The chemical connection of the chromphores in silica matrixes effectively prevented their leakage from silica and facilitated their energy transfer, thus endowing the core–shell nanoprobe with excellent stability and brightness. The nanoprobe showed a lifetime up to 0.52 ms and a phosphorescence quantum yield up to 1% in aqueous solution, which is 10 times over that in organic solvent. Together with the excellent oxygen permeability of the mesoporous silica structure, sensitive ratiometric hypoxia detection both in solution and in living cells was achieved with the KSV value of 243.1 and I0/I100 ratio of 153.
Results and discussion
Design, synthesis, characterization and photophysical properties of the silica nanoprobe
Schemes 1 and 2 show the one-pot preparation of the ratiometric hypoxia nanoprobe and synthetic routes to the silanized coumarin derivative (CM)15d and silanized Pd(II) porphyrin derivative (TPP). CM and TPP were selected as the fluorescence reference and phosphorescence indicator precursors, respectively, owing to their easy synthesis, the distinct spectral separation between the fluorescence from CM and phosphorescence from TPP, and the substantial overlap between the emission spectrum of CM and the absorption spectrum of TPP. The efficient energy transfer from the fluorescent reference to the phosphorescent indicator led to the design of a ratiometric hypoxia sensor with enhanced phosphorescence intensity.18 After hydrolysis/condensation of tetraethoxysilane (TEOS) with CM and TPP in the presence of a micellar dispersion of Pluronic F-127 as a template, the nanoprobe was obtained with a silica core surrounded by a PEG shell (see details in the ESI†). Transmission electron microscope (TEM) images indicated that the nanoprobe is monodispersed with an average diameter of 10 ± 2 nm. The hydrodynamic diameter of the nanoprobe, shown by dynamic light scattering measurement (DLS), is 18 ± 2 nm (Fig. 1a and Fig. S1, ESI†). No aggregation was observed by DLS or UV-vis spectral changes even after several months’ storage, indicating outstanding stability of the nanoprobe.
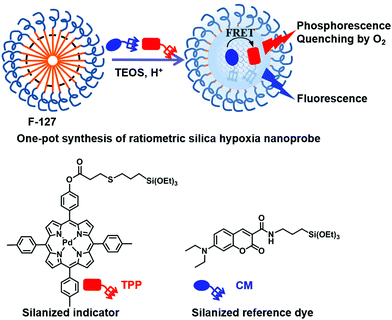 |
| Scheme 1 Schematic illustration of the one-pot preparation of ratiometric hypoxia nanoprobes based on covalent-bonded silica nanoparticles (NPs); the chemical structures of the oxygen-sensitive indicator (TPP) and oxygen-insensitive reference dyes (CM). | |
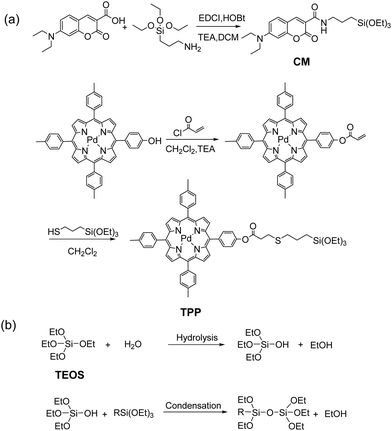 |
| Scheme 2 (a) Synthetic routes to CM and TPP; (b) hydrolysis and condensation reactions of TEOS and silanized dyes. | |
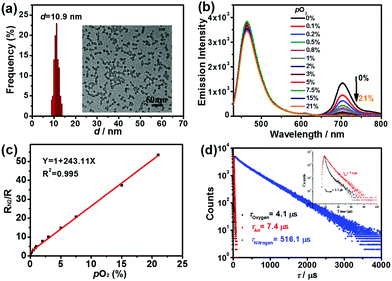 |
| Fig. 1 (a) The TEM diameter distribution and image of the silica nanoprobe. (b) Ratiometric emission spectral response of the silica nanoprobe in aqueous solution at different oxygen levels (0%, 0.1%, 0.2%, 0.5%, 0.8%, 1%, 2%, 3%, 5%, 7.5%, 15%, 21%), λex = 410 nm. (c) Linear Stern–Volmer plot of RN2/R as a function of oxygen partial pressure. (d) Phosphorescence decays at 704 nm of the nanoprobe in aqueous solution saturated with O2, N2 and air (λex = 405 nm). The concentration of the nanoprobe is 1.4 × 10−7 M.20 | |
A collection of silica nanoparticles with different loading levels of CM and TPP, referred to as CMx@SiO2 and TPPy@SiO2, were prepared. The fluorescence of the silica nanoparticles (NPs) increased with increasing loading levels of CM from 0.1% to 0.25%, then decreased and broadened at the loading level of 0.5% (Fig. S2, ESI†). A 0.25% dye to TEOS ratio was initially taken as the preferable ratio. Then, silica nanoparticles of TPP at the ratio of 0.25% (TPP0.25@SiO2) were investigated, the phosphorescence quantum yield of which was measured to be 0.4% in aqueous solution, 4 times over that of TPP in chloroform (ϕ = 0.1%) (Table 1). Obviously, the nonradiative decay processes of the indicator induced by interaction with water and excited-state conformational mobility were inhibited through the covalent connection with the silica matrix and the rigid environments of the nanoparticles. Moreover, this method could ensure covalent-bonding of the dyes and polysiloxane chains quantitatively. During purification by dialysis, no significant trace of unreacted dyes was observed in the filtrate by spectrophotometric analysis, while the absorption of the silica NPs increased quantitatively with increasing loading levels of the CM monomer (Fig. S2a, ESI†).15
Table 1 Photophysical properties of CM and TPP in different media
Sample |
λ
abs [nm] |
ε
max [M−1 cm−1] |
λ
em [nm] |
ϕ
|
τ
|
Fluorescence quantum yield, λex = 370 nm, with 9,10-diphenylanthracene (DPA, ϕ = 90% in cyclohexane) as a fluorescence standard.
Phosphorescence quantum yield, λex = 410 nm, with [Ru(bpy)3]Cl2 (ϕ = 9.4% in acetonitrile) as a standard.
Fluorescence decays at maximum emission (λex = 405 nm).
Phosphorescence decays at 704 nm under air (λex = 405 nm).
Phosphorescence decays at 704 nm under N2 (λex = 405 nm).
In aqueous solution.
|
CM in CHCl3 |
418 |
4.1 × 104 |
449 |
92.7%a |
2.9 nsc |
TPP in CHCl3 |
416, 525 |
3.3 × 105, 3.0 × 104 |
704 |
0.1%b |
4.1 μsd, 69.3 μse |
CM0.25@SiO2f |
418 |
2.4 × 104 |
468 |
25.0%a |
3.0 nsc |
TPP0.25@SiO2f |
416, 524 |
1.7 × 105, 2.0 × 104 |
704 |
0.4%b |
6.9 μsd, 370.5 μse |
CM0.25TPP0.25@SiO2f |
416, 524 |
4.6 × 106, 4.1 × 105 |
468, 704 |
1.0%b |
1.3 nsc, 7.4 μsd, 516.1 μse |
Then, we prepared a series of dual-dye loaded silica nanoparticles at different ratios and examined the emission spectra carefully to confirm the final ratios for the nanoprobe (Fig. S2c, ESI†). Loading of CM and TPP at molar percentage ratios of x and y with respect to TEOS, was referred to as CMxTPPy@SiO2. Considering the preferable fluorescence/phosphorescence intensity ratios and luminescence quantum yields, 0.25% of CM and 0.25% of TPP (CM0.25TPP0.25@SiO2) in moles with respect to TEOS was selected for the nanoprobe (see ESI† for details).
The photophysical properties of the nanoprobe were carefully studied (Table 1, see details in the ESI†) The characteristic absorption bands of the nanoprobe at 416 nm (εmax = 4.6 × 106 M−1 cm−1) are attributed to CM and the Soret band of TPP, while the absorption band at 524 nm corresponds to the Q bands of TPP (εmax = 4.1 × 105 M−1 cm−1). Upon excitation at 410 nm, the dual-dye loaded nanoprobe showed both fluorescence at 430–600 nm and phosphorescence at 630–800 nm resulting from CM and TPP, respectively (Fig. S3, ESI†). The phosphorescence quantum yield of the nanoprobe in aqueous solution was enhanced 10 times to 1% compared to that of TPP in chloroform (ϕ = 0.1%). We attributed this further enhancement of phosphorescence to the efficient energy transfer between CM (donor) and TPP (acceptor) in the confined nanospace, which is evident by the significantly reduced fluorescence intensity of the nanoprobe compared to that of CM0.25@SiO2 under otherwise identical conditions (Fig. S4, ESI†). The energy transfer efficiency (ηET) of the nanoprobe was calculated as 92% based on the fluorescenc quenching CM after the introduction of TPP in the nanoprobes, where the Förster radius R0 was estimated to be 4.1 nm between the energy donor (CM) and acceptor (TPP). The phosphorescence lifetime of the nanoprobe at 704 nm was measured to be 516.1 μs in aqueous solution, which was extremely enhanced compared to that of TPP in chloroform under nitrogen atmosphere (69.3 μs) (Fig. S5, ESI†). This long lifetime would greatly improve the sensitivity of the sensors.7d
Oxygen sensing in aqueous solution
To examine the hypoxia detection capability of the designed nanoprobe, the ratiometric emission spectral response in aqueous solution at different oxygen levels (0%, 0.1%, 0.2%, 0.5%, 0.8%, 1%, 2%, 3%, 5%, 7.5%, 15%, 21%) was investigated. Fig. 1b shows that the fluorescence at 465 nm in the spectra was almost completely independent of the oxygen partial pressure, while the phosphorescence at 704 nm was dramatically quenched with increasing oxygen partial pressure, indicating its ratiometric reponses to oxygen at 465/704 nm. Even 0.2% of oxygen partial levels could lead to over 50% decrease of the phosphorescence intensity, indicating its high sensitivity to extreme hypoxia environment.
To analyze the ratiometric oxygen sensing quantitatively, the Stern–Volmer quenching constant (KSV) was calculated based on eqn (1)19 and the data in Fig. 1b, where R is defined as the ratio of phosphorescence intensity at 704 nm to that of the fluorescence intensity at 465 nm (R = IP/IF), RN2 is the emission intensity ratio in fully deoxygenated solution, and pO2 is the oxygen partial pressure.
As shown in Fig. 1c, there is a highly linear relationship between RN2/R and pO2, whose KSV is obtained as 243.11 bar−1. On the other hand, the ratio of the luminescence intensities in oxygen-free and in oxygen-saturated solution is as high as 153 (IN2/IO2 = 153), which is rather high among the reported silica oxygen probes (Table S2, ESI†).7d In addition, the quenching efficiency (Q) was calculated as 99.3%, close to 100% (see details in the ESI†). The phosphorescence decays in oxygen, air and nitrogen were measured, respectively (Fig. 1d). All phosphorescence decay curves exhibited bi-exponential decay kinetics. The average lifetime of phosphorescence at 704 nm decreased from 516.1 μs in the nitrogen-saturated solution to 7.4 μs in the air-saturated solution, and to 4.1 μs in the oxygen-saturated solution. The phosphorescence lifetime under nitrogen atmosphere is 126 times over that of under oxygen atmosphere (τN2/τO2 = 126), indicating high sensitivity of the nanoprobe.
The detection of singlet oxygen
Owing to the triplet–triplet energy transfer (TTET) between the triplet excited state of the porphyrin in the nanoprobe and the ground state of molecular oxygen (3O2), 1O2 would be generated accompanying the oxygen sensing process. This process was confirmed by spin trap experiments in conjunction with electron paramagnetic resonance (EPR) spectroscopy. When 2,2,6,6-tetramethylpiperidine (TEMP) was employed as a scavenger for 1O2, as shown in Fig. 2a, EPR signals attributed to 1O2 were detected in the aqueous solution of the nanoprobe (100 μL, 4 μM) upon irradiation. No significant EPR signal changes were detected when the sample was irradiated in the absence of nanoprobe under identical conditions, confirming that 1O2 was generated from the nanoprobe (Fig. S6, ESI†).
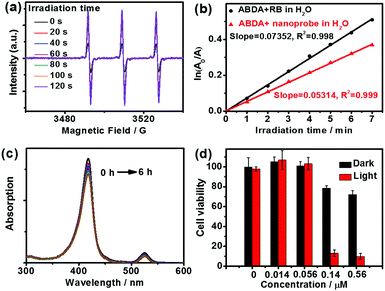 |
| Fig. 2 (a) X-Band EPR spectra of the air-saturated aqueous solution of the nanoprobe (100 μL, 4 μM) with TEMP (3 μL) upon irradiation by a Hg lamp (100 mW). (b) Plots of the natural logarithm of the optical density of ABDA (at 378 nm) vs. irradiation time in the presence of nanoprobe (red line) and RB (black line) in H2O. (c) Absorption spectra of the nanoprobe in aqueous solution (1.4 × 10−7 M), upon illumination by a medium pressure Hg lamp (300 W) with a glass filter (cutoff < 400 nm) at 6 cm distance. (d) Nanoprobe concentration dependence of the CCK-8 cell viability of HeLa cells under dark conditions and by a 532 nm laser at a power of 5.0 mW cm−2 for 20 min. Nanoprobe in the concentration range of 0–0.56 μM. | |
To further assess the capability of the nanoprobe to generate 1O2, the singlet oxygen quantum yield (φΔ) of the nanoprobe in air-saturated solution was also measured by using the chemical trapping method, where 9,10-anthracenediyl-bi(methylene)dimalonic acid (ABDA) was employed as the trapping agent, and Rose Bengal (RB, φΔ = 0.75 in H2O)21 was used as the standard. As illustrated in Fig. 2b and Fig. S7 (ESI†), the absorbance of the ABDA solution at 378 nm decreased gradually with prolonged irradiation time by a 532 nm laser in the presence of the nanoprobe and RB, in which a linear relationship between ln(A0/A) and irradiation time was observed and the φΔ was measured to be 0.55 in air-saturated aqueous solution.
Ratiometric hypoxia sensing and imaging in living cells
With the above impressive results, we tested the capacity of the nanoprobe for hypoxia sensing and imaging in living cells by confocal laser scanning microscopy (CLSM) (Fig. 3).22 The cells were incubated at 37 °C for 1 h under 21% and 1% oxygen atmosphere, respectively, after introducing the nanoprobe into HeLa cells. The observed blue and red luminescence confirmed that the nanoprobe was cell-permeable. Upon excitation by a 405 nm laser, signals in the blue channel (430–470 nm, Fig. 3b and g) and red channel (660–740 nm, Fig. 3c and h) were collected. The blue emission channel was assigned to the fluorescence from CM segments; the fluorescence intensities from the cells were almost the same under 21% and 1% O2 concentrations. In contrast, the red channel corresponding to the phosphorescent TPP segments shows a brighter image under 1% O2 conditions than under 21% O2 conditions. No obvious morphological changes of the cells were observed during the measurement. An evident change in the Iblue/Ired ratio from 3.4 under air to 1.7 under 1% O2 conditions in living cells concluded that the silica nanoprobe is capable of visualizing the hypoxia status in living cells (Fig. 3e and j).
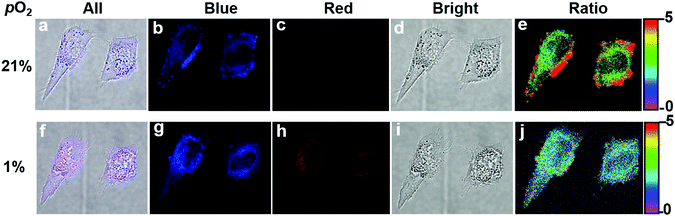 |
| Fig. 3 Confocal luminescence, bright-field images and ratiometric luminescence images (λex = 405 nm) of living HeLa cells incubated with the silica nanoprobe (1.4 × 10−9 M) at 21% (a–e) and 1% (f–j) oxygen partial pressure. In luminescence imaging, the blue (430–470 nm) and red (660–740 nm) channels were collected. In ratiometric imaging, the ratio of emission intensity at 430–470 nm to that at 660–740 nm (Iblue/Ired) was chosen as the detected signals. (Scale bar: 20 μm.) | |
Photostability and cytotoxicity are important parameters for a sensor. The UV-vis absorption spectra negligibly changed even after 6 h irradiation, indicating good stability of the nanoprobe (Fig. 2c). The dark and photocytotoxicity of the nanoprobe in HeLa cells was measured using a standard Cell Counting Kit-8 (CCK-8) assay at various concentrations (0–0.54 μM). As shown in Fig. 2d, a prominent photocytotoxicity was detected under the PDT process, while little effect on the survival of HeLa cells was observed under dark conditions at the same concentration. The investigations revealed that our prepared nanoprobe possesses low cytotoxicity, good biocompatibility and potential photodynamic cancer therapy capabilities.
Conclusions
In summary, we have developed silica-supported dual-dye nanoprobes for ratiometric hypoxia detection. The covalent-bonded phosphorescent indicator and fluorescent reference dye with silica in the nanoprobes led to enhanced phosphorescence brightness, long lifetime (0.52 ms), excellent stability and sensitivity to O2 (KSV = 243.11 bar−1, Q = 99.3%). Together with the simple preparation, good biocompatibility and ratiometric hypoxia sensing in living cells, the covalently doped silica nanoprobe demonstrated its potential for future biomedical applications.
Conflicts of interest
There are no conflicts to declare.
Acknowledgements
Financial support from the National Natural Science Foundation of China (Grants 22071258, 21871280 and 21861132004), the Ministry of Science and Technology of China (Grant 2017YFA0206903), the Strategic Priority Research Program of the Chinese Academy of Sciences (Grant XDB17000000), the Key Research Program of Frontier Sciences of the Chinese Academy of Sciences (Grant QYZDY-SSW-JSC029), and K. C. Wong Education Foundation is gratefully acknowledged. The authors thank Dr Ye Tian, Si-Jia Gao and Na Tian from TIPC for their great help with the cell experiments.
Notes and references
-
(a) G. L. Semenza, Life with oxygen, Science, 2007, 318, 62–64 CrossRef CAS;
(b) M. Höckel and P. Vaupel, Tumor Hypoxia: Definition and Current Clinical, Biologic, and Molecular Aspects, J. Natl. Cancer Inst., 2001, 93, 266–276 CrossRef.
- W. R. Wilson and M. P. Hay, Targeting Hypoxia in Cancer Therapy, Nat. Rev. Cancer, 2011, 11, 393–410 CrossRef CAS.
- J. E. Selfridge, E. Lezi, J. Lu and R. H. Swerdlow, Role of Mitochondrial Homeostasis and Dynamics in Alzheimer's Disease, Neurobiol. Dis., 2013, 51, 3–12 CrossRef CAS.
- K. Prass, A. Scharff, K. Ruscher, D. Lowl, C. Muselmann, I. Victorov, K. Kapinya, U. Dirnagl and A. Meisel, Hypoxia-Induced Stroke Tolerance in the Mouse is Mediated by Erythropoietin, Stroke, 2003, 34, 1981–1986 CrossRef CAS.
-
(a) X. Zheng, X. Wang, H. Mao, W. Wu, B. Liu and X. Jiang, Hypoxia-Specific Ultrasensitive Detection of Tumours and Cancer Cells in vivo, Nat. Commun., 2015, 6, 5834 CrossRef CAS;
(b) E. Roussakis, Z. Li, A. J. Nichols and C. L. Evans, Oxygen-Sensing Methods in Biomedicine from the Macroscale to the Microscale, Angew. Chem., Int. Ed., 2015, 54, 8340–8362 CrossRef CAS.
- J.-N. Liu, W. Bu and J. Shi, Chemical Design and Synthesis of Functionalized Probes for Imaging and Treating Tumor Hypoxia, Chem. Rev., 2017, 117, 6160–6224 CrossRef CAS.
-
(a) R. P. Briñas, T. Troxler, R. M. Hochstrasser and S. A. Vinogradov, Phosphorescent Oxygen Sensor with Dendritic Protection and Two-Photon Absorbing Antenna, J. Am. Chem. Soc., 2005, 127, 11851–11862 CrossRef;
(b) P. Lehner, C. Staudinger, S. M. Borisov and I. Klimant, Ultra-sensitive Optical Oxygen Sensors for Characterization of Nearly Anoxic Systems, Nat. Commun., 2014, 5, 4460 CrossRef CAS;
(c) K. Y. Zhang, P. Gao, G. Sun, T. Zhang, X. Li, S. Liu, Q. Zhao, K. K.-W. Lo and W. Huang, Dual-Phosphorescent Iridium(III) Complexes Extending Oxygen Sensing from Hypoxia to Hyperoxia, J. Am. Chem. Soc., 2018, 140, 7827–7834 CrossRef CAS;
(d) X.-d. Wang and O. S. Wolfbeis, Optical Methods for Sensing and Imaging Oxygen: Materials, Spectroscopies and Applications, Chem. Soc. Rev., 2014, 43, 3666–3761 RSC.
-
(a) H. Xiang, L. Zhou, Y. Feng, J. Cheng, D. Wu and X. Zhou, Tunable Fluorescent/Phosphorescent Platinum(II) Porphyrin–Fluorene Copolymers for Ratiometric Dual Emissive Oxygen Sensing, Inorg. Chem., 2012, 51, 5208–5212 CrossRef CAS;
(b) A. J. Nichols, E. Roussakis, O. J. Klein and C. L. Evans, Click-Assembled, Oxygen-Sensing Nanoconjugates for Depth-Resolved, Near-Infrared Imaging in a 3D Cancer Model, Angew. Chem., Int. Ed., 2014, 53, 3671–3674 CrossRef CAS;
(c) R. J. Meier, S. Schreml, X.-d. Wang, M. Landthaler, P. Babilas and O. S. Wolfbeis, Simultaneous Photographing of Oxygen and pH In Vivo Using Sensor Films, Angew. Chem., Int. Ed., 2011, 50, 10893–10896 CrossRef CAS.
-
(a) C. Xing, Q. Xu, H. Tang, L. Liu and S. Wang, Conjugated Polymer/Porphyrin Complexes for Efficient Energy Transfer and Improving Light-Activated Antibacterial Activity, J. Am. Chem. Soc., 2009, 131, 13117–13124 CrossRef CAS;
(b) Y. Tian, B. R. Shumway and D. R. Meldrum, A New Cross-Linkable Oxygen Sensor Covalently Bonded into Poly(2-hydroxyethyl methacrylate)-co-Polyacrylamide Thin Film for Dissolved Oxygen Sensing, Chem. Mater., 2010, 22, 2069–2078 CrossRef CAS;
(c) C. Wu, B. Bull, K. Christensen and J. McNeill, Ratiometric Single-Nanoparticle Oxygen Sensors for Biological Imaging, Angew. Chem., Int. Ed., 2009, 48, 2741–2745 CrossRef CAS;
(d) A. V. Kondrashina, R. I. Dmitriev, S. M. Borisov, I. Klimant, I. O’Brien, Y. M. Nolan, A. V. Zhdanov and D. B. Papkovsky, A Phosphorescent Nanoparticle-Based Probe for Sensing and Imaging of (Intra)Cellular Oxygen in Multiple Detection Modalities, Adv. Funct. Mater., 2012, 22, 4931–4939 CrossRef CAS;
(e) S. Wang, K. Gu, Z. Guo, C. Yan, T. Yang, Z. Chen, H. Tian and W.-H. Zhu, Self-assembly of a Monochromophore-Based Polymer Enables Unprecedented Ratiometric Tracing of Hypoxia, Adv. Mater., 2018, 1805735 Search PubMed.
-
(a) R. Xu, Y. Wang, X. Duan, K. Lu, D. Micheroni, A. Hu and W. Lin, Nanoscale Metal–Organic Frameworks for Ratiometric Oxygen Sensing in Live Cells, J. Am. Chem. Soc., 2016, 138, 2158–2161 CrossRef CAS;
(b) Y. Yu, M. S. Kwon, J. Jung, Y. Zeng, M. Kim, K. Chung, J. Gierschner, J. H. York, S. M. Borisov and J. Kim, Room-Temperature-Phosphorescence-Based Dissolved Oxygen Detection by Core-Shell Polymer Nanoparticles Containing Metal-Free Organic Phosphors, Angew. Chem., Int. Ed., 2017, 56, 16207–16211 CrossRef CAS;
(c) G. Zhang, G. M. Palmer, M. W. Dewhirst and C. L. Fraser, A Dual-emissive-materials Design Concept Enables Tumor Hypoxia Imaging, Nat. Mater., 2009, 8, 747–751 CrossRef CAS.
-
(a) E. J. McLaurin, A. B. Greytak, M. G. Bawendi and D. G. Nocera, Two-Photon Absorbing Nanocrystal Sensors for Ratiometric Detection of Oxygen, J. Am. Chem. Soc., 2009, 131, 12994–13001 CrossRef CAS;
(b) C. M. Lemon, P. N. Curtin, R. C. Somers, A. B. Greytak, R. M. Lanning, R. K. Jain, M. G. Bawendi and D. G. Nocera, Metabolic Tumor Profiling with pH, Oxygen, and Glucose Chemosensors on a Quantum Dot Scaffold, Inorg. Chem., 2014, 53, 1900–1915 CrossRef CAS;
(c) A. Shamirian, H. Samareh Afsari, A. Hassan, L. W. Miller and P. T. Snee, In Vitro Detection of Hypoxia Using a Ratiometric Quantum Dot-Based Oxygen Sensor, ACS Sens., 2016, 1, 1244–1250 CrossRef CAS.
-
(a) R.-F. Wang, H.-Q. Peng, P.-Z. Chen, L.-Y. Niu, J.-F. Gao, L.-Z. Wu, C.-H. Tung, Y.-Z. Chen and Q.-Z. Yang, A Hydrogen-Bonded-Supramolecular-Polymer-Based Nanoprobe for Ratiometric Oxygen Sensing in Living Cells, Adv. Funct. Mater., 2016, 26, 5419–5425 CrossRef CAS;
(b) Y.-Y. Huang, Y. Tian, X.-Q. Liu, Z. Niu, Q.-Z. Yang, V. Ramamurthy, C.-H. Tung, Y.-Z. Chen and L.-Z. Wu, Luminescent Supramolecular Polymer Nanoparticles for Ratiometric Hypoxia Sensing, Imaging and Therapy, Mater. Chem. Front., 2018, 2, 1893–1899 RSC;
(c) X.-Q. Liu, K. Zhang, Y.-Z. Chen, C.-H. Tung and L.-Z. Wu, Monochromophore-Based Phosphorescence and Fluorescence from Pure Organic Assemblies for Ratiometric Hypoxia Detection, Angew. Chem., Int. Ed., 2020, 59 DOI:10.1002/anie.202007039;
(d) W.-C. Geng, S. Jia, Z. Zheng, Z. Li, D. Ding and D.-S. Guo, A Noncovalent Fluorescence Turn-on Strategy for Hypoxia Imaging, Angew. Chem., Int. Ed., 2019, 58, 2377–2381 CrossRef CAS.
- X. Huang, J. Song, B. C. Yung, X. Huang, Y. Xiong and X. Chen, Ratiometric Optical Nanoprobes Enable Accurate Molecular Detection and Imaging, Chem. Soc. Rev., 2018, 47, 2873–2920 RSC.
-
(a) B. Lei, B. Li, H. Zhang, S. Lu, Z. Zheng, W. Li and Y. Wang, Mesostructured Silica Chemically Doped with RuII as a Superior Optical Oxygen Sensor, Adv. Funct. Mater., 2006, 16, 1883–1891 CrossRef CAS;
(b) Y.-E. L. Koo, Y. Cao, R. Kopelman and S. M. Koo, Real-Time Measurements of Dissolved Oxygen Inside Live Cells by Organically Modified Silicate Fluorescent Nanosensors, Anal. Chem., 2004, 76, 2498–2505 CrossRef CAS;
(c) S. M. Borisov, P. Lehner and I. Klimant, Novel Optical Trace Oxygen Sensors Based on Platinum(II) and Palladium(II) Complexes with 5,10,15,20-Meso-tetrakis-(2,3,4,5,6-pentafluorphenyl)-porphyrin Covalently Immobilized on Silica-gel Particles, Anal. Chim. Acta, 2011, 690, 108–115 CrossRef CAS;
(d) M. Villa, B. D. Secco, L. Ravotto, M. Roy, E. Rampazzo, N. Zaccheroni, L. Prodi, M. Gingras, S. A. Vinogradov and P. Ceroni, Bright Phosphorescence of All-Organic Chromophores Confined within Water-Soluble Silica Nanoparticles, J. Phys. Chem. C, 2019, 123, 29884–29890 CrossRef CAS.
-
(a) S. Bonacchi, D. Genovese, R. Juris, M. Montalti, L. Prodi, E. Rampazzo and N. Zaccheroni, Luminescent Silica Nanoparticles: Extending the Frontiers of Brightness, Angew. Chem., Int. Ed., 2011, 50, 4056–4066 CrossRef CAS;
(b) M. Montalti, L. Prodi, E. Rampazzo and N. Zaccheroni, Dye-doped silica nanoparticles as luminescent organized systems for nanomedicine, Chem. Soc. Rev., 2014, 43, 4243–4268 RSC;
(c) C. Caltagirone, A. Bettoschi, A. Garau and R. Montis, Silica-based nanoparticles: a versatile tool for the development of efficient imaging agents, Chem. Soc. Rev., 2015, 44, 4645–4671 RSC;
(d) D. Genovese, S. Bonacchi, R. Juris, M. Montalti, L. Prodi, E. Rampazzo and N. Zaccheroni, Prevention of Self-Quenching in Fluorescent Silica Nanoparticles by Efficient Energy Transfer, Angew. Chem., Int. Ed., 2013, 52, 5965–5968 CrossRef CAS;
(e) C. Wang, Y. Chen and D. Wu, Preparation and Photophysical Properties of Pyren-doped Silica Fluorescent Nanoparticles, Chem, J. Chin. Univ., 2018, 5, 917–925 Search PubMed;
(f) T. He, X. Yang, Y. Chen, Z. Tong and L. Wu, Triplet-Triplet Annihilation Upconversion Based on Silica Nanoparticles, Acta Chim. Sin., 2019, 77, 41–46 CrossRef CAS.
-
(a) J. Liu, Y. Liu, W. Bu, J. Bu, Y. Sun, J. Du and J. Shi, Ultrasensitive Nanosensors Based on Upconversion Nanoparticles for Selective Hypoxia Imaging in Vivo upon Near-Infrared Excitation, J. Am. Chem. Soc., 2014, 136, 9701–9709 CrossRef CAS;
(b) X.-d. Wang, J. A. Stolwijk, T. Lang, M. Sperber, R. J. Meier, J. Wegener and O. S. Wolfbeis, Ultra-Small, Highly Stable, and Sensitive Dual Nanosensors for Imaging Intracellular Oxygen and pH in Cytosol, J. Am. Chem. Soc., 2012, 134, 17011–17014 CrossRef CAS.
- Q. Li and Z. Li, Molecular Packing: Another Key Point for the Performance of Organic and Polymeric Optoelectronic Materials, Acc. Chem. Res., 2020, 53, 962–973 CrossRef CAS.
-
(a) H.-Q. Peng, L.-Y. Niu, Y.-Z. Chen, L.-Z. Wu, C.-H. Tung and Q.-Z. Yang, Biological Applications of Supramolecular Assemblies Designed for Excitation Energy Transfer, Chem. Rev., 2015, 115, 7502–7542 CrossRef CAS;
(b) Y. Toshitada, Y. Yuji, H. Masahiro, T. Toshiyuki and T. Seiji, Ratiometric Molecular Sensor for Monitoring Oxygen Levels in Living Cells, Angew. Chem., Int. Ed., 2012, 51, 4148–4151 CrossRef;
(c) Y.-Z. Ma, H. Xiao, X.-F. Yang, L.-Y. Niu, L.-Z. Wu, C.-H. Tung, Y.-Z. Chen and Q.-Z. Yang, Bidirectional Singlet and Triplet Energy Transfer via the 2-Ureido-4[1H]-pyrimidinone Quadruple Hydrogen-Bonded Module, J. Phys. Chem. C, 2016, 120, 16507–16515 CrossRef CAS;
(d) H.-Q. Peng, Y.-Z. Chen, Y. Zhao, Q.-Z. Yang, L.-Z. Wu, C.-H. Tung, L.-P. Zhang and Q.-X. Tong, Artificial Light-Harvesting System Based on Multifunctional Surface-Cross-Linked Micelles, Angew. Chem., Int. Ed., 2012, 51, 2088–2092 CrossRef CAS;
(e) P.-Z. Chen, Y.-X. Weng, L.-Y. Niu, Y.-Z. Chen, L.-Z. Wu, C.-H. Tung and Q.-Z. Yang, Light-Harvesting Systems Based on Organic Nanocrystals To Mimic Chlorosomes, Angew. Chem., Int. Ed., 2016, 55, 2759–2763 CrossRef CAS.
- O. Stern and M. Volmer, über die Abklingzeit der Fluoreszenz, Phys. Z., 1919, 20, 183–188 CAS.
- E. Rampazzo, S. Bonacchi, R. Juris, M. Montalti, D. Genovese, N. Zaccheroni, L. Prodi, D. C. Rambaldi, A. Zattoni and P. Reschiglian, Energy Transfer from Silica Core–Surfactant Shell Nanoparticles to Hosted Molecular Fluorophores, J. Phys. Chem. B, 2010, 114, 14605–14613 CrossRef CAS.
- J. Ge, M. Lan, B. Zhou, W. Liu, L. Guo, H. Wang, Q. Jia, G. Niu, X. Huang, H. Zhou, X. Meng, P. Wang, C. S. Lee, W. Zhang and X. Han, A graphene quantum dot photodynamic therapy agent with high singlet oxygen generation, Nat. Commun., 2014, 5, 4596 CrossRef CAS.
- B. R. Smith and S. S. Gambhir, Nanomaterials for In Vivo Imaging, Chem. Rev., 2017, 117, 901–986 CrossRef CAS.
Footnote |
† Electronic supplementary information (ESI) available. See DOI: 10.1039/d0qm00653j |
|
This journal is © the Partner Organisations 2021 |