DOI:
10.1039/D0QM00625D
(Research Article)
Mater. Chem. Front., 2021,
5, 315-323
High-performance ionic conductive poly(vinyl alcohol) hydrogels for flexible strain sensors based on a universal soaking strategy†
Received
26th August 2020
, Accepted 28th September 2020
First published on 29th September 2020
Abstract
Conductive materials with predominant mechanical properties and high sensitivity have promising applications in various fields such as fabrication of electric skins, wearable sensors, and soft robotics. High-performance and flexible strain sensors based on conductive hydrogels have attracted significant attention because of their potential applications in voice recognition and human–machine interfaces. Inspired by the mechanism of neural tissue signal transmission, herein, a high-strength and relatively high-sensitivity ionic conductive hydrogel was developed by utilizing the completely physically crosslinked polyvinyl alcohol (PVA) and a simple inorganic salt solution soaking strategy. The ionic conductive hydrogels exhibited intriguing remoldability and excellent mechanical properties such as superb tensile strength (8.03 MPa), high toughness (28.7 MJ m−3), and high elastic modulus (1 MPa) because of their high-density hydrogen bonding and chain entanglement networks. By integrating the PVA–NaCl gel into a flexible strain sensor, the sensor displayed high conductivity (7.14 S m−1) at room temperature and subzero temperature, high accuracy (GF = 0.989), and sensitive strain responsiveness in a wide strain detection window of 0.2–400%. These results imply good performance for monitoring and distinguishing various human daily activities and slight physiological signals. Furthermore, the as-made PVA sol could convert to gel state just by being injected on a flexible substrate under an ice-bath and the conductive hydrogels displayed excellent biocompatibility and improved cell proliferation. Therefore, the as-formulated hydrogel sensor can have promising applications in wearable devices, such as sports monitoring, healthcare monitoring or voice recognition.
1. Introduction
In recent years, flexible, wearable, and human friendly intelligent devices have drawn considerable attention for applications in electronic skins (e-skins), implantable sensors, and healthcare monitoring devices.1–9 These wearable sensors can synchronously detect the changes in external deformations in real-time and convert them into electrical signals with high accuracy and sensitivity.10,11 However, traditional flexible devices prepared by combining conductive materials and polymer films as well as elastomers are rigid and brittle with low sensitivity. The unsatisfactory stretchability and sensitivity limit their practical applications.4,12,13 Conductive hydrogels with excellent flexibility, stretchability, and sensitivity are promising candidates for the next-generation electronics. There are two primary strategies to prepare conductive hydrogels. One is the addition of intrinsically conductive nanofillers to the hydrogel matrix, such as metal nanoparticles, carbon nanotubes, graphenes, polyaniline, and polypyrrole.14–19 Another approach is to utilize the conductive properties of ions to achieve conductivity in hydrogels, which is usually obtained by using polyelectrolytes or adding solvated deliquescent salts (typically, NaCl, KCl, and LiCl).7,20–24 For instance, Zhang et al. developed a biocompatible polyvinyl alcohol (PVA) hybrid hydrogel sensor by the addition of single-wall carbon nanotubes, which could detect human daily activities such as finger bending, chewing, and breathing.25 Wu et al. prepared a ionic hydrogel sensor with good biocompatibility and adjustable adhesion by integrating nanoclay into the hydrogel matrix, which could quickly and precisely detect subtle signal changes.8 Li et al. fabricated a stretchable and conductive elastomer based on PVA/PEI hydrogels by introducing solvated deliquescent salts (LiCl), which displayed high stretchability, conductivity, and biosafety.3 However, most of these conductive materials exhibit limited mechanical strength and low sensitivity that also in a relatively narrow strain window, which seriously restricts their potential applications.26
Several techniques such as addition of effective nanomaterials or increasing the crosslink density have been proposed to enhance the mechanical strength of conductive hydrogels.27–29 Introduction of nanomaterials can improve the mechanical properties to a certain extent. However, these nanomaterials do not easily disperse uniformly in the hydrogel matrix and their long-term biocompatibility should also be considered when applied to implantable sensors or healthcare monitoring.30 The increase in cross-link density can enhance the mechanical properties of ionic conductive hydrogels; however, the ion migration rate also decreases simultaneously. Most of the reported traditional conductive hydrogels displayed low mechanical strength and suffered from rapid deterioration of signal detection capability under repeatedly large strains. Hence, it is challenging to ameliorate both high conductivity and mechanical properties at the same time. Inspired by biological tissues, the addition of highly water-soluble salts to a functional polymer matrix with complicated structures is ideal for creating high-strength ionic conductors. However, these complex functional materials are difficult to synthesize, and there is energy wastage as well as high production expenses, which may impede their potential applications. Hence, it is required to utilize the cost-effective, easily synthesizable, and easy molding materials to obtain highly conductive and high-strength ionic conductive hydrogels. The commercially available PVA hydrogel has excellent biocompatibility and can be easily prepared. The preparation process of the PVA hydrogel sheds light on the design and preparation of a hydrogel matrix with adequate strength and high sensitivity via freezing/thawing treatment and usage of inorganic salt solution.25,31
Herein, we integrate inorganic salts (NaCl) into a physically crosslinked PVA network using a universal soaking strategy to endow PVA hydrogels with a relatively high sensitivity, mechanical strength, and ionic conductivity. The superior mechanical properties of the PVA–N hydrogel can be ascribed to ample inter-molecular hydrogen bonds and the crystallization of PVA chains as well as the chain-entanglement networks due to the salting-out effect. Moreover, the migration of Na+ and Cl− ions into the hydrogel network endows PVA–N gels with excellent ionic conductivity and a relatively high sensitivity, for applications in ultrasensitive sensors such as human-motion detectors, voice recognition sensors, and wearable devices. Moreover, the tunable structures, mechanics, and sensitivity of PVA–N hydrogels can be easily obtained by adjusting the crystallization times and soaking time in situ, which can be used to match different and complex requirements. All test results indicated that these conductive gels retain sub-zero conductivity, a broad strain window, and excellent biocompatibility. Therefore, the as-prepared ionic conductive hydrogel is an excellent candidate for flexible strain sensors.
2. Experimental
2.1. Materials
Poly(vinyl alcohol) (MW: 14
600–18
600, 99%) was purchased from Aladdin, and sodium chloride (99%) was supplied by Sigma-Aldrich. Methylene blue and rhodamine B were obtained from Sigma-Aldrich and deionized water was used in the experiment (18.2 MΩ cm resistivity).
2.2. Preparation of ionic conductive hydrogels
Briefly, a desired amount of PVA was uniformly dispersed in an aqueous solution by heating to 96 °C under vigorous stirring for about 3 h. After standing defoaming, the homogeneous solution was quickly transferred into a sealed mold. PVA hydrogels were obtained by placing in a refrigerator at −20 °C for 4 h, followed by thawing at 25 °C for 12 h. By changing the PVA contents, PVA-x hydrogels were obtained (x refers to the weight percentage: 3%, 7%, and 10%). Then, the PVA-x hydrogel were immersed in a saturated salt solution for different times to formulate PVA–N-y ionic conductive hydrogels (y refers to the soaking time) (Table S1, ESI†).
2.3. Instruments and methods
Fourier transform infrared (FTIR) spectroscopy.
The structure of hydrogels was analyzed by Fourier transform infrared spectroscopy (Tensor II, Bruker) at 25 °C.
Wide-angle X-ray diffraction (WAXD).
The lyophilized samples were investigated by wide-angle X-ray diffraction (WXRD) (Rigaku TTR-III) at room temperature in the 2θ range of 5–60°.
Morphological observation.
The morphology of samples was observed using a scanning electron microscope (SEM, QUANTA 200, FEI). Before observation, the samples were freeze-dried. Then, the cross-sections were carefully observed using an SEM.
Rheological measurements.
Dynamic rheological measurements were conducted using a Rheometric Scientific HAAKE Rheo-Stress 600 instrument equipped with 20 mm parallel plates at room temperature. The strain deformation was fixed at 0.5% (linear viscoelastic range) to avoid any nonlinear response. The frequency sweeps were conducted at angular velocities ranging from 0.01 Hz to 10 Hz.
Mechanical tests.
Hydrogels were cut into rectangular shapes (30 mm × 20 mm × 1 mm) for the tensile tests at room temperature. Tensile stress tests and sequential loading–unloading cycle were conducted using a tensile tester (UTM, Shenzhen Suns Technology Stock Co. Ltd) at a constant velocity of 50 mm min−1. The elastic modulus (E) was calculated from the slope of the stress–strain curve (5–20%) and the toughness (fracture energy) was total area under the stress–strain curve.
2.4. Characterization of electrical properties
The electrical conductivity (σ) was studied using an electrochemical workstation (LK2005A). The conductivity of the hydrogel was calculated using the following formula (1): |  | (1) |
where L is the distance between adjacent electrodes (cm), R is the resistance of hydrogel (Ω) and S is the cross-sectional area of the hydrogel (cm2). The variation in electrical resistance of the hydrogel was obtained using the following formula (2): |  | (2) |
where R0 and R are the resistances without and with applied strain, respectively.
2.5. Low-field 1H NMR
Low-field NMR tests were performed using a Bruker Minispec mq20 low-field spectrometer. The minispec had a typical π/2 pulse length of about 3 μs and a receiver dead time of about 13 μs.
2.6. Magic-sandwich echo (MSE)
In general, free induction decay (FID) of proton NMR signal misses the initial decaying signals due to the dead time of a low-field NMR spectrometer. Magic-sandwich echo (MSE) pulse sequence was used to recover the lost FID signals.32,33 In multiphase materials, the rigid components with strong proton dipolar couplings have a fast decay of proton signals, while the mobile component possesses a slow decay of FID signals because of the averaging effect of molecular motions. Therefore, the refocused low-field MSE-FID NMR signal decay signals can be fitted to the following eqn (3):34 | I(t) = fr exp(−(t/T2r)2) + fi exp(−(t/T2i)ni) + fs exp(−(t/T2s)ns) | (3) |
where fr, fi, and fs are the relative contents of rigid components, intermediate components, and soft components, respectively, and fr + fi + fs = 1. T2r, T2i, and T2s indicate the relaxation time of rigid component, intermediate component, and mobile components, respectively (T2r < T2i < T2s).
2.7. Cell viability
Cytotoxicity of hydrogel extracts toward cells was analyzed by an MTT assay.35,36 The HeLa cells were cultured in Dulbecco's modified Eagle's medium (DMEM), containing 10% fetal bovine serum (FBS). Then, 100 μL of the HeLa cell solution was added into 96-well culture plates at a density of 8 × 104 cells per mL. The hydrogels were submerged in 70% ethanol for 60 min and washed thoroughly with sterilized PBS buffer. Next, the hydrogel was immersed in DMEM (10 mg mL−1) for 24 h to obtain the hydrogel extracts. The wells without hydrogel extracts were set as control. After incubation for 48 h, the medium was removed and replaced with 50 μL MTT solution (1 mg mL−1) for 3 h. Then, the 100 μL per well DMSO was added to completely dissolve the crystals. The absorbance of each well at 570 nm was measured using a microplate reader (Multiskan, Ascent). The relative cell viability is expressed as follows (4): | 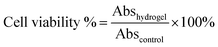 | (4) |
where Abshydrogel and Abscontrol are the absorbances for cells cultured in hydrogel extracts and DMEM, respectively. The proliferation of cells was observed using a fluorescence microscopy (Olympus IX51) after staining with the AO/EB dye.
3. Results and discussion
Fig. 1a shows the fabrication process of PVA–N hydrogel. PVA powders of varied contents were first stirred at 96 °C for 3 h (Table S1, ESI†). After thrice freeze–thaw treatment, the PVA hydrogel was formed. Then, the ionic conductive PVA–N hydrogel was prepared by immersion into a saturated saline solution. In the XRD spectra of lyophilized hydrogels (Fig. 1b), typical PVA crystalline peaks at 20.18° and 23.07° were obtained and the peak intensity was also increased with the increase in freezing/thawing cycles. To judge the effect of freezing/thawing and soaking time on the mobility of amorphous PVA chains, MSE experiment was performed and the results are presented in Fig. 1c. The acquisition time of the low-field MSE-FID NMR signal was cut at 200 μs because of the magnetic field inhomogeneity.37 The crystallization of PVA chains (corresponding to the rigid components) have a fast decay of FID signals, while water of the hydrogel system (corresponding to the soft components) possess a slow decay of proton signals because of the averaging effect of the molecular motion. The intermediate decay corresponds to the response of the intermediate components, which can be ascribed to the interaction between PVA chains and water molecules. As listed in Table S2 (ESI†), with the increase of freezing/thawing times, the fraction of rigid domain in the PVA hydrogel increased from 5.04% to 10.07% due to the crystallization of PVA chains. These results indicated that the hydrogel system had a denser network structure, which is consistent with the cross-sectional morphology of PVA hydrogels with various freezing/thawing cycles (Fig. 2d–f). After treatment with NaCl solution for different times, the fraction of rigid domain increased to 29.57% due to the salting-out effect, shielded electrostatic repulsions, and increased interchain hydrophobic interactions. The rigid domain can form intermolecular aggregates, which can serve as junction points in the hydrogel network.29,38 The fraction of soft components in PVA-3rd-F/T and PVA-3–N-10 were 84.2% and 57.93%, respectively, which is consistent with the water content of the PVA hydrogel at no soaking and soaking balance state (Fig. S3c, ESI†). The FTIR spectra of lyophilized PVA and PVA–N hydrogels (Fig. 1d) display typical characteristic stretching bands of the hydroxyl group at 3447 cm−1, indicating the successful fabrication of PVA hydrogel.
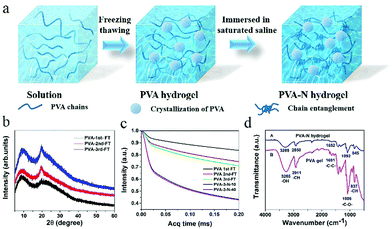 |
| Fig. 1 (a) Schematic fabrication of PVA–N hydrogel. (b) XRD spectra for lyophilized hydrogels with various freezing/thawing cycles. (c) Low-field MSE-FID NMR signal of PVA hydrogels with various freezing/thawing cycles and soaking time at room temperature. (d) FTIR patterns spectra for lyophilized PVA gels and PVA–N gels. | |
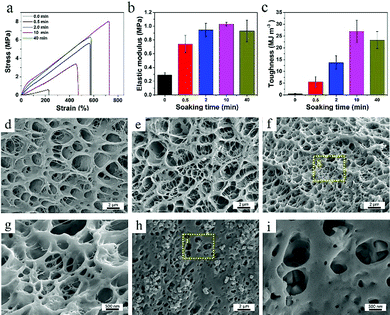 |
| Fig. 2 (a) Tensile strain–stress curves of PVA–N hydrogels with different soaking times. (b and c) Corresponding elastic modulus and toughness. (d–f) SEM images of PVA hydrogel with various freezing/thawing cycles. (g) Magnified images of PVA-3rd-F/T hydrogel. (h) SEM images of PVA–N hydrogel. (i) Magnified images of PVA–N hydrogel. | |
The crystallization time and PVA chain content have significant influence on the network structure of hydrogel, resulting in a great change in mechanical strength (see Fig. S1, ESI†). With the increase in crystallization times, the tensile stress increased from 24.8 kPa to 587 kPa, while the elastic modulus increased from 16.7 kPa to 292 kPa due to an increase in the crystalline region of PVA hydrogel. Meanwhile, the microstructures of PVA hydrogel became more tight and uniform, as observed in the SEM images (Fig. 2d–f). When crystallization process was three times and the weight percentage of PVA was 10%, the PVA–N hydrogel exhibited the highest tensile strength (587 kPa) and elastic modulus (292 kPa) (Fig. S1c and d, ESI†). This prepared PVA–N hydrogel was used in the following study. Fig. 2a–c displays the tensile curves, elastic modulus, and toughness of PVA–N hydrogels with different soaking times. After immersion into the saturated NaCl solutions for different times, the mechanical strength of PVA–N hydrogels was dramatically enhanced. For instance, the tensile stress was increased from 0.587 MPa to 8.03 MPa, which is higher than that for the previously reported PVA ionic conductive hydrogels.3,5,31,39–41 The elastic modulus was also increased from 0.3 to 1.03 MPa. The toughness (curve area) values that are affected by the fracture stress and fracture strain reached the maximum value of 28.7 MJ m−3 at 10 min of soaking time (Fig. S2a and b, ESI†), which is already higher than those for most of the published hydrogels.2,42,43 In order to ensure the maximum strength, the PVA hydrogel immersed for 10 min was selected for subsequent testing. Moreover, changing soaking time can also regulate the solid contents of PVA–N hydrogels and the transparent PVA–N hydrogels can turn opaque along with the salting-out effect and increased interchain hydrophobic interactions (Fig. S3a, ESI†). With the increase in soaking time, the solid content of hydrogels was gradually increased from 13.8 wt% to 49.2 wt% (Fig. S3b, ESI†) and the microstructures as well as pore sizes of hydrogels became much denser than that of PVA hydrogel, as shown in Fig. 2h and i. Therefore, the structure and mechanics of PVA hydrogel can be effectively tuned by regulating the crystallization times and soaking times.
Owing to an abundance of hydrogen bonding interactions and PVA crystallization points, the PVA–N hydrogel displayed excellent mechanical properties. Fig. 3 displays the mechanical properties of PVA–N hydrogel. As shown in Fig. 3a and b, the PVA–N hydrogels can withstand various deformations such as bending, twisted stretching, and knotting. Notably, the two crossed gel strips could be elongated to almost three times of its original length without rupture. Moreover, a series of successive loading–unloading measurements were performed to evaluate the energy dissipation mechanism of PVA–N gel. During the stretching process, the PVA–N hydrogel dissipated energy, leading to a large hysteresis. As shown in Fig. 3c and d, the PVA–N hydrogels displayed different hysteresis loops and the loops increased gradually with the increase in strain, which contributed to more physical dynamic cross-linking points via destruction and recombination, causing an increase in the hysteresis loop. As shown in Fig. 3e and f, the hysteresis energy per unit volume of PVA–N hydrogel increased from 0.3 MJ m−3 at 50% strain to 13.2 MJ m−3 at 500% strain. Moreover, the dissipation coefficient increased as setting strain increased and reached a balanced state at 300%, indicating that the partly unzipped PVA crystalline domains undergoing deformation and hydrogen bonding showed powerful energy dissipation in a composite hydrogel.
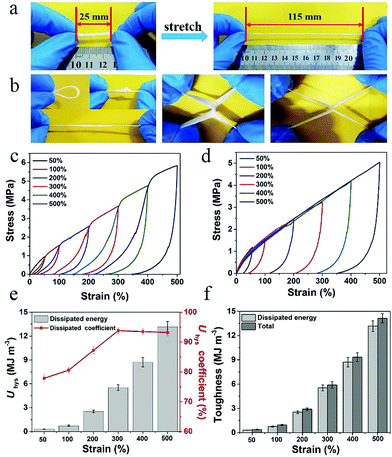 |
| Fig. 3 (a) PVA–N hydrogel was stretched and (b) twisted and knotted. (c) Loading–unloading tests of PVA–N hydrogel under varied setting strains without resting intervals and (d) loading–unloading cycle behaviors of PVA–N hydrogel under varied setting strains (50%, 100%, 200%, 300%, 400%, and 500%); (e) corresponding dissipated energy and dissipation coefficient. (f) Calculated total and dissipated energy of PVA–N hydrogel under different strains. | |
To investigate the viscoelastic characteristics of PVA and PVA–N hydrogels, oscillatory rheology tests were performed and the results are presented in Fig. 4. In the linear region, the storage modulus (G′) was higher than loss modulus (G′′), implying an excellent elastic behavior for both PVA and PVA–N hydrogels. As shown in Fig. 4b, G′ and G′′ of PVA and PVA–N hydrogels possessed frequency dependence at room temperature. The G′ and G′′ values of PVA–N hydrogel were consistently higher than those of PVA hydrogel for the entire frequency range, indicating an excellent stability and elastic solid-like behavior of the hydrogels. Besides, the loss modulus (G′′) of PVA–N hydrogel was higher than the storage modulus (G′) of PVA hydrogel, demonstrating that the rheological properties of two gel materials were different, which is consistent with the results of tensile tests that the mechanical strength of PVA–N hydrogel was clearly better than that of PVA hydrogel.
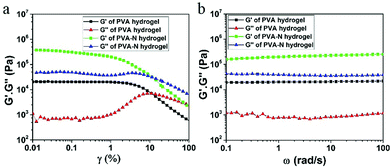 |
| Fig. 4 Dynamic storage modulus and loss modulus of PVA and PVA–N hydrogels as a function of (a) strain and (b) frequency. | |
In the process of preparing the PVA–N hydrogels, the step of immersion in salt solution not only enhanced the mechanical properties of PVA hydrogel but also endowed the gel with excellent conductivity because of the migration of a large number of Na+ and Cl− ions in the network. Moreover, salt inhibits the crystallization of water, which is responsible for the decrease in the freezing point of water in PVA–N gels. As shown in Fig. 5g and h, the PVA–N hydrogel possesses excellent conductive performance at room temperature and sub-zero temperature. Hence, it may be used as ionic wires to play music in the sub-zero temperature range (Fig. 5i and Video S1, ESI†). Due to the fully physical crosslink network of PVA hydrogel, the hydrogel could be reshaped into different shapes and PVA sol would be an ideal ink for casual writing on a glass under an ice-bath at about −2 °C (Fig. S4, ESI†). After soaking into salt solution, the PVA–N conductive hydrogel could be used as a switch to control the on and off of LED lights (Video S2, ESI†). The strain sensing of the fabricated hydrogel was tested by using it in a complete circuit connecting a LED light (Fig. 5). As shown in Fig. 5a, when the gel was elongated from 0% to 100%, the brightness of LED was synchronously reduced, signifying that the conductivity of PVA–N ionic hydrogel was affected by the deformation. The conductivity of PVA–N hydrogels was about 7.14 S m−1 and increased with the soaking time, which is a reasonably high electrical conductivity at 25 °C.44 (Fig. 5e). In order to ensure the optimal mechanical strength and conductivity, the sample immersed in a saturated salt solution for 10 min was selected for subsequent testing. Fig. 5b shows the change in relative resistance as a function of strain. The results indicate that relative resistance exhibited a step-like trend with a step-by-step stretching cycle by application of 50% and 100% stretched strains. The lightness of LED was consistent with the cut and contact states, as shown in Fig. 5c. The bulb was extinguished as the hydrogel was cut into two parts. After contacting, the circuit was re-established and the bulb glowed again as the brightness diminished slightly, which can be ascribed to the gaps and misalignments in the contact process. The curves of relative resistance in Fig. 5d further suggest that the contact process led to an increase in the relative resistance. Fig. 5f shows the chart for comparison between this work and references in terms of ionic conductivity versus tensile stress. The PVA–N hydrogel constructed in this work can achieve higher ionic conductivity (7.14 S m−1) and higher tensile stress (8.03 MPa), outperforming those of previously reported conductive (organo) hydrogels.20,31,40,45–56
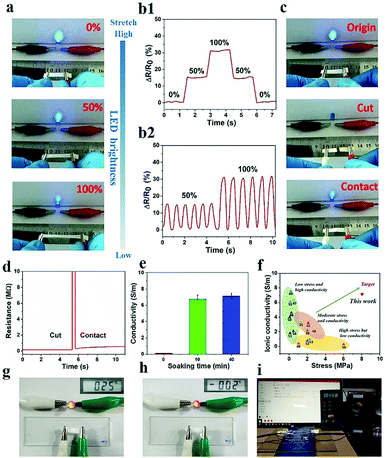 |
| Fig. 5 (a) Photographs of changes in LED brightness and (b1–2) variation in the relative resistance along with strain at 50% and 100%. (c) Photographs of changes in LED brightness and (d) relative resistance variation at original, cut, and re-contact state. (e) Electromechanical properties of PVA–N hydrogels with different soaking times of NaCl. (f) Ashby plot of ionic conductivity and tensile stress with other reported ionic conductive (organo) hydrogels (ref. 20, 31, 40 and 45–56). (g and h) Conductive performance of the PVA–N hydrogel at 25 °C and −2 °C. (i) Conductivity of PVA–N hydrogels as ionic wires in the sub-zero temperature range. | |
The prepared hydrogel not only possessed excellent conductivity, but also retained superb sensitivity to a wide strain window (0.2–400%). As shown in Fig. 6a–d, the PVA–N hydrogel quickly responded to different strains without delay. The strain sensitivity of PVA–N hydrogel can be assessed by the common parameter gauge factor (GF), which is the ratio of relative resistance variation and the applied strain, according to the equation: GF = (ΔR/R0)/ε = [(R − R0)/R0]/ε, where R0 and R are the resistance in the original state and real-time resistance under the stretching state, respectively. Fig. 6d shows that the GF value of PVA–N ionic hydrogel increased with the increase in strain. The relative resistance increased linearly at first with a gauge factor of 0.42 (0–80%) increased to 0.807 (80–200%), and then, reached 0.989 (200–400%). Fig. 6e shows that the GF value of the sensor increased more rapidly in the low strain regime (0–5%) and increased to 0.989 at the strain of 400%. Fig. 6f further indicates that the ionic hydrogel had a good linear relationship with a slight deformation (0–5%), and the GF value reached 0.357, signifying that the sensor possessed high sensitivity and improved conductivity as compared to similar previously reported soft hydrogel strain sensors.4,40,41,57,58 Besides, stability of the ionic hydrogel was tested by applying cyclic uniaxial force at 50% strain for about 300 cycles (Fig. 6g and h). The relative resistance changed with the cyclic loading of strain. No clear loss of signals was observed in the inset curve, indicating that the sensor possessed excellent stability and repeatability to external strain.
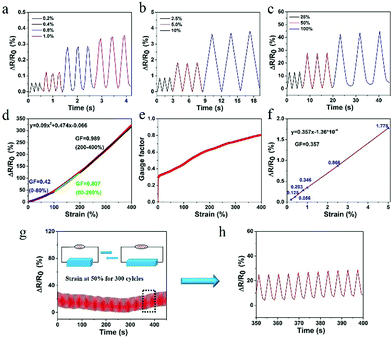 |
| Fig. 6 (a–c) Change in the relative resistance of sensors for different cycling tensile strains (0.2–100%). (d) Change in the relative resistance of sensors as a function of tensile strain (0–400%) and (e) the effects of various applied strains on the gauge factor. (f) Relative resistance variation of the hydrogels under subtle strains. (g and h) Stability of relative resistance changes of the hydrogels under a strain of 50%. | |
The flexible hydrogel sensor was fixed on different parts of human body and connected to the digital source meter to monitor the changes in relative resistance in real-time. The PVA–N ionic hydrogel sensor exhibited excellent responsiveness to both large and subtle physiological movements. Fig. 7a and b show the resistance variation of the PVA–N hydrogel attached onto the elbow and wrist of a volunteer. The results indicate repeatable and regular frequency signal changes for flexion and extension movements. The deformation of the elbow joint was significantly larger than that of the wrist, thus, there was a clear difference in the trend of change in relative resistance. Besides, the sensor can be applied to detect full-range joint motions in real time. As shown in Fig. 7c, the relative resistance of hydrogel sensor increased with the finger bending angle from 30°, 60°, to 90°, which could be attributed to the elongation of the PVA–N hydrogel, leading to an increase in the relative resistance (Video S3, ESI†). The resistance variation of neck bending was also recorded by the hydrogel sensor (Fig. 7d). The different in frequency resistance signal patterns could be ascribed to the speed of bending and degree of deformation. Moreover, Fig. 7e and f exhibit the relative resistance variations in PVA–N hydrogel sensors with inhale/exhale and knee joints at different bending states, respectively. The PVA–N hydrogel sensor demonstrated a relatively high sensitivity and fast and repeatable responsiveness for the detection of different and complex human motions, which makes it a promising candidate for wearable smart devices and biosignal monitoring devices.
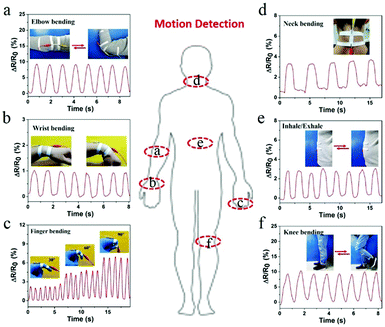 |
| Fig. 7 Real-time relative resistance variations of hydrogel sensors attached to different human joints: (a) elbow; (b) wrist; (c) finger; (d) neck; (e) breathe; and (f) knee joint. | |
Other than the detection of macroscopic deformation of human body motions, the PVA–N ionic sensor may also be applied to measure subtle muscle movements involved in speaking. Fig. 8a and b show the real-time resistance changes of hydrogel sensor attached onto the throat during light coughing and swallowing. The real-time relative resistance change of swallowing was greater than that of light coughing, indicating that the movement of throat knot during swallowing up and down was greater than during light cough. Besides, the sensor demonstrated different curve changes for the same word “Thank you” tested by different volunteers. As shown in Fig. 8c and d, the curves display three highly similar characteristic peaks and valleys. Compared with voices of boys, the voices of girls have a higher pitch; thus the peak of curve is sharper, indicating that the prepared strain sensor may be used to recognize the timbre. Moreover, the hydrogel sensor could also recognize different English alphabets and phrases from different languages, as shown in Fig. S5 (ESI†) and Fig. 8e. All these four words were spoken by the same person and recorded three times. All these mean the same “Greetings” in four languages, namely, Chinese, English, Korean, and Japanese, respectively. The three pronunciations of each curve possess good repeatability, and different curves were distinguishable. All these results indicated that the prepared strain sensor has stable, repeatable, and sensitive subtle muscle movement detection capability. Such a sensor may find special applications in phonation recognition, automatic translation, and voice control switches for people who are unable to speak.
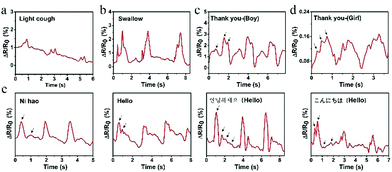 |
| Fig. 8 (a and b) Subtle muscle movements of the throat with light cough and swallow, and (c and d) the throat as different person says complicated phrase ‘‘thank you’’. (e) Subtle muscle movements of throat as the same person says the phrase ‘‘hello’’ three times in different languages as Chinese, English, Korean, and Japanese, respectively. | |
To evaluate the cytocompatibility of PVA–N hydrogel, HeLa cells were chosen to observe the proliferation on the surface of hydrogels by fluorescence microscopy, as shown in Fig. 9. The results indicate that the cells grew well on the surface of hydrogels. Additionally, the cell viability was more than 80% for all the culturing times and increased with time, manifesting that PVA–N hydrogel was non-toxic and conducive to cell reproduction, which is important for the application in soft electronic skins, intelligent sensors, and so on.
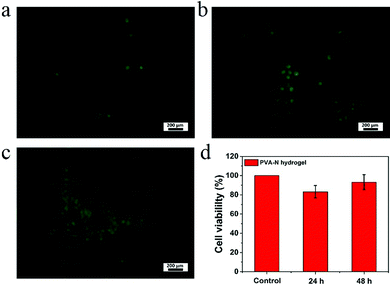 |
| Fig. 9 Fluorescence images of HeLa cells incubated on the surface of PVA–N hydrogel after (a) 0 h, (b) 24 h, and (c) 48 h of culture. (d) In vitro cytocompatibility of HeLa cells, as measured by an MTT assay. | |
4. Conclusions
In summary, a high-performance ionic conductive hydrogel has been developed based on a universal soaking strategy. The prepared PVA–N hydrogel possessed intriguing remoldability and excellent mechanical properties (e.g., high strength, toughness, and high elastic modulus), which can be attributed to the high-density hydrogen bonding network, dense crystalline domains, and chain entanglement network. Uniform porous networks were observed in the conductive hydrogel, which acted as a pathway for the ionic transport. As a flexible strain sensor, the hydrogel had a stable, repeatable, and extremely sensitive strain responsiveness in a wide strain detection window (0.2–400%). These results make the sensor suitable for both varied human daily activities and monitoring of slight physiological movements. Moreover, the as-made PVA sol could convert into gel state just by being injected on a flexible substrate under an ice-bath, which could be processed into different PVA–N hydrogels. Furthermore, the hydrogel displayed excellent biocompatibility and cell affinity. To sum up, this work provided a technology for the fabrication of fast, adjustable, high-strength, and relatively high-sensitivity ionic conductive hydrogels, which can have potential applications in wearable devices, such as sports monitoring, healthcare monitoring or voice recognition.
Besides, bio-adhesiveness is an important performance for the practical application of such materials. Future work of this system will focus on developing a suitable solution to effectively improve its bioadhesion while maintaining its good sensitivity.
Conflicts of interest
There are no conflicts to declare.
Acknowledgements
This work was funded by the Key Program of National Natural Science Foundation of China (21534005).
References
- Z. Lei and P. Wu, A supramolecular biomimetic skin combining a wide spectrum of mechanical properties and multiple sensory capabilities, Nat. Commun., 2018, 9, 1134 CrossRef.
- S. Xia, S. Song, F. Jia and G. Gao, A flexible, adhesive and self-healable hydrogel-based wearable strain sensor for human motion and physiological signal monitoring, J. Mater. Chem. B, 2019, 7, 4638–4648 RSC.
- C. Wang, K. Hu, C. Zhao, Y. Zou, Y. Liu, X. Qu, D. Jiang, Z. Li, M. R. Zhang and Z. Li, Customization of Conductive Elastomer Based on PVA/PEI for Stretchable Sensors, Small, 2020, 1904758 CrossRef CAS.
- X. Jing, H.-Y. Mi, Y.-J. Lin, E. Enriquez, X.-F. Peng and L.-S. Turng, Highly Stretchable and Biocompatible Strain Sensors Based on Mussel-Inspired Super-Adhesive Self-Healing Hydrogels for Human Motion Monitoring, ACS Appl. Mater. Interfaces, 2018, 10, 20897–20909 CrossRef CAS.
- S. Zhang, Y. Zhang, B. Li, P. Zhang, L. Kan, G. Wang, H. Wei, X. Zhang and N. Ma, One-Step Preparation of a Highly Stretchable, Conductive, and Transparent Poly(vinyl alcohol)-Phytic Acid Hydrogel for Casual Writing Circuits, ACS Appl. Mater. Interfaces, 2019, 11, 32441–32448 CrossRef CAS.
- Y. Liu, K. He, G. Chen, W. R. Leow and X. Chen, Nature-Inspired Structural Materials for Flexible Electronic Devices, Chem. Rev., 2017, 117, 12893–12941 CrossRef CAS.
- W. Kong, C. Wang, C. Jia, Y. Kuang, G. Pastel, C. Chen, G. Chen, S. He, H. Huang, J. Zhang, S. Wang and L. Hu, Muscle-Inspired Highly Anisotropic, Strong, Ion-Conductive Hydrogels, Adv. Mater., 2018, 30, 1801934 CrossRef.
- X. Di, Y. Kang, F. Li, R. Yao, Q. Chen, C. Hang, Y. Xu, Y. Wang, P. Sun and G. Wu, Poly(N-isopropylacrylamide)/polydopamine/clay nanocomposite hydrogels with stretchability, conductivity, and dual light- and thermo-responsive bending and adhesive properties, Colloids Surf., B, 2019, 177, 149–159 CrossRef CAS.
- X. Di, C. Hang, Y. Xu, Q. Ma, F. Li, P. Sun and G. Wu, Bioinspired tough, conductive hydrogels with thermally reversible adhesiveness based on nanoclay confined NIPAM polymerization and a dopamine modified polypeptide, Mater. Chem. Front., 2020, 4, 189–196 RSC.
- C. Wang, X. Li, E. Gao, M. Jian, K. Xia, Q. Wang, Z. Xu, T. Ren and Y. Zhang, Carbonized Silk Fabric for Ultrastretchable, Highly Sensitive, and Wearable Strain Sensors, Adv. Mater., 2016, 28, 6640–6648 CrossRef CAS.
- X. Wang, Z. Liu and T. Zhang, Flexible Sensing Electronics for Wearable/Attachable Health Monitoring, Small, 2017, 13, 1602790 CrossRef.
- H. Qin, R. E. Owyeung, S. R. Sonkusale and M. J. Panzer, Highly stretchable and nonvolatile gelatin-supported deep eutectic solvent gel electrolyte-based ionic skins for strain and pressure sensing, J. Mater. Chem. C, 2019, 7, 601–608 RSC.
- B. Yang and W. Yuan, Highly Stretchable and Transparent Double-Network Hydrogel Ionic Conductors as Flexible Thermal-Mechanical Dual Sensors and Electroluminescent Devices, ACS Appl. Mater. Interfaces, 2019, 11, 16765–16775 CrossRef CAS.
- C. J. Han, H. P. Chiang and Y. C. Cheng, Using Micro-Molding and Stamping to Fabricate Conductive Polydimethylsiloxane-Based Flexible High-Sensitivity Strain Gauges, Sensors, 2018, 18, 618 CrossRef.
- Y. Liu, K. Xu, Q. Chang, M. A. Darabi, B. Lin, W. Zhong and M. Xing, Highly Flexible and Resilient Elastin Hybrid Cryogels with Shape Memory, Injectability, Conductivity,
and Magnetic Responsive Properties, Adv. Mater., 2016, 28, 7758–7767 CrossRef CAS.
- S. Chun, W. Son and C. Choi, Flexible pressure sensors using highly-oriented and free-standing carbon nanotube sheets, Carbon, 2018, 139, 586–592 CrossRef CAS.
- M. Zhang, C. Wang, H. Wang, M. Jian, X. Hao and Y. Zhang, Carbonized Cotton Fabric for High-Performance Wearable Strain Sensors, Adv. Funct. Mater., 2017, 27, 1604795 CrossRef.
- S. Lin, H. Yuk, T. Zhang, G. A. Parada, H. Koo, C. Yu and X. Zhao, Stretchable Hydrogel Electronics and Devices, Adv. Mater., 2016, 28, 4497–4505 CrossRef CAS.
- S. Luo and T. Liu, SWCNT/graphite nanoplatelet hybrid thin films for self-temperature-compensated, highly sensitive, and extensible piezoresistive sensors, Adv. Mater., 2013, 25, 5650–5657 CrossRef CAS.
- Y. Wang, L. Zhang and A. Lu, Transparent, Antifreezing, Ionic Conductive Cellulose Hydrogel with Stable Sensitivity at Subzero Temperature, ACS Appl. Mater. Interfaces, 2019, 11, 41710–41716 CrossRef CAS.
- J. Wu, Z. Wu, S. Han, B.-R. Yang, X. Gui, K. Tao, C. Liu, J. Miao and L. K. Norford, Extremely Deformable, Transparent, and High-Performance Gas Sensor Based on Ionic Conductive Hydrogel, ACS Appl. Mater. Interfaces, 2019, 11, 2364–2373 CrossRef CAS.
- J. Y. Sun, C. Keplinger, G. M. Whitesides and Z. Suo, Ionic skin, Adv. Mater., 2014, 26, 7608–7614 CrossRef CAS.
- C. Kim, H. Lee, K. H. Oh and J. Sun, Highly stretchable, transparent ionic touch panel, Science, 2016, 353, 682–687 CrossRef CAS.
- K. Tian, J. Bae, S. E. Bakarich, C. Yang, R. D. Gately, G. M. Spinks, M. In Het Panhuis, Z. Suo and J. J. Vlassak, 3D Printing of Transparent and Conductive Heterogeneous Hydrogel-Elastomer Systems, Adv. Mater., 2017, 29, 1604827 CrossRef.
- M. Liao, P. Wan, J. Wen, M. Gong, X. Wu, Y. Wang, R. Shi and L. Zhang, Wearable, Healable, and Adhesive Epidermal Sensors Assembled from Mussel-Inspired Conductive Hybrid Hydrogel Framework, Adv. Funct. Mater., 2017, 27, 1703852 CrossRef.
- P. Calvert, Hydrogels for Soft Machines, Adv. Mater., 2009, 21, 743–756 CrossRef CAS.
- P. Lin, S. Ma, X. Wang and F. Zhou, Molecularly Engineered Dual-Crosslinked Hydrogel with Ultrahigh Mechanical Strength, Toughness, and Good Self-Recovery, Adv. Mater., 2015, 27, 2054–2059 CrossRef CAS.
- Y. Yang, X. Wang, F. Yang, L. Wang and D. Wu, Highly Elastic and Ultratough Hybrid Ionic-Covalent Hydrogels with Tunable Structures and Mechanics, Adv. Mater., 2018, 30, 1707071 CrossRef.
- Y. Yang, X. Wang, F. Yang, H. Shen and D. Wu, A Universal Soaking Strategy to Convert Composite Hydrogels into Extremely Tough and Rapidly Recoverable Double-Network Hydrogels, Adv. Mater., 2016, 28, 7178–7184 CrossRef CAS.
- C. Ma, X. Le, X. Tang, J. He, P. Xiao, J. Zheng, H. Xiao, W. Lu, J. Zhang, Y. Huang and T. Chen, A Multiresponsive Anisotropic Hydrogel with Macroscopic 3D Complex Deformations, Adv. Funct. Mater., 2016, 26, 8670–8676 CrossRef CAS.
- Y. Zhou, C. Wan, Y. Yang, H. Yang, S. Wang, Z. Dai, K. Ji, H. Jiang, X. Chen and Y. Long, Highly Stretchable, Elastic, and Ionic Conductive Hydrogel for Artificial Soft Electronics, Adv. Funct. Mater., 2019, 29, 1806220 CrossRef.
- W. K. Rhim, A. Pines and J. S. Waugh, Time-Reversal Experiments in Dipolar-Coupled Spin Systems, Phys. Rev. B: Solid State, 1971, 3, 684–696 CrossRef.
- M. Mauri, Y. Thomann, H. Schneider and K. Saalwachter, Spin-diffusion NMR at low field for the study of multiphase solids, Solid State Nucl. Magn. Reson., 2008, 34, 125–141 CrossRef CAS.
- A. Papon, K. Saalwächter, K. Schäler, L. Guy, F. Lequeux and H. Montes, Low-Field NMR Investigations of Nanocomposites: Polymer Dynamics and Network Effects, Macromolecules, 2011, 44, 913–922 CrossRef CAS.
- M. Mehdizadeh, H. Weng, D. Gyawali, L. Tang and J. Yang, Injectable citrate-based mussel-inspired tissue bioadhesives with high wet strength for sutureless wound closure, Biomaterials, 2012, 33, 7972–7983 CrossRef CAS.
- M. Cencer, Y. Liu, A. Winter, M. Murley, H. Meng and B. P. Lee, Effect of pH on the rate of curing and bioadhesive properties of dopamine functionalized poly(ethylene glycol) hydrogels, Biomacromolecules, 2014, 15, 2861–2869 CrossRef CAS.
- R. Zhang, S. Yu, S. Chen, Q. Wu, T. Chen, P. Sun, B. Li and D. Ding, Reversible cross-linking, microdomain structure, and heterogeneous dynamics in thermally reversible cross-linked polyurethane as revealed by solid-state NMR, J. Phys. Chem. B, 2014, 118, 1126–1137 CrossRef CAS.
- S. Ladet, L. David and A. Domard, Multi-membrane hydrogels, Nature, 2008, 452, 76 CrossRef CAS.
- S. Pan, M. Xia, H. Li, X. Jiang, P. He, Z. Sun and Y. Zhang, Transparent, high-strength, stretchable, sensitive and anti-freezing poly(vinyl alcohol) ionic hydrogel strain sensors for human motion monitoring, J. Mater. Chem. C, 2020, 8, 2827–2837 RSC.
- H. Chen, X. Ren and G. Gao, Skin-Inspired Gels with Toughness, Antifreezing, Conductivity, and Remoldability, ACS Appl. Mater. Interfaces, 2019, 11, 28336–28344 CrossRef CAS.
- Y. J. Liu, W. T. Cao, M. G. Ma and P. Wan, Ultrasensitive Wearable Soft Strain Sensors of Conductive, Self-healing, and Elastic Hydrogels with Synergistic “Soft and Hard” Hybrid Networks, ACS Appl. Mater. Interfaces, 2017, 9, 25559–25570 CrossRef CAS.
- Q. Zhang, X. Liu, X. Ren, F. Jia, L. Duan and G. Gao, Nucleotide-Regulated Tough and Rapidly Self-Recoverable Hydrogels for Highly Sensitive and Durable Pressure and Strain Sensors, Chem. Mater., 2019, 31, 5881–5889 CrossRef CAS.
- Y. Zhu, S. Liu, X. Shi, D. Han and F. Liang, A thermally responsive host–guest conductive hydrogel with self-healing properties, Mater. Chem. Front., 2018, 2, 2212–2219 RSC.
- X. Zhang, N. Sheng, L. Wang, Y. Tan, C. Liu, Y. Xia, Z. Nie and K. Sui, Supramolecular nanofibrillar hydrogels as highly stretchable, elastic and sensitive ionic sensors, Mater. Horiz., 2019, 6, 326–333 RSC.
- Y. Ye, Y. Zhang, Y. Chen, X. Han and F. Jiang, Cellulose Nanofibrils Enhanced, Strong, Stretchable, Freezing Tolerant Ionic Conductive Organohydrogel for Multi Functional Sensors, Adv. Funct. Mater., 2020, 2003430 CrossRef CAS.
- F. Lin, Z. Wang, Y. Shen, L. Tang, P. Zhang, Y. Wang, Y. Chen, B. Huang and B. Lu, Natural skin-inspired versatile cellulose biomimetic hydrogels, J. Mater. Chem. A, 2019, 7, 26442–26455 RSC.
- G. Chen, J. Huang, J. Gu, S. Peng, X. Xiang, K. Chen, X. Yang, L. Guan, X. Jiang and L. Hou, Highly tough supramolecular double network hydrogel electrolytes for an artificial flexible and low-temperature tolerant sensor, J. Mater. Chem. A, 2020, 8, 6776–6784 RSC.
- J. Xu, R. Jin, X. Ren and G. Gao, Cartilage-inspired hydrogel strain sensors with ultrahigh toughness, good self-recovery and stable anti-swelling properties, J. Mater. Chem. A, 2019, 7, 25411–25448 Search PubMed.
- L. Guan, S. Yan, X. Liu, X. Li and G. Gao, Wearable strain sensors based on casein-driven tough, adhesive and anti-freezing hydrogels for monitoring human-motion, J. Mater. Chem. B, 2019, 7, 5230–5236 RSC.
- Y. Yang, L. Guan, X. Li, Z. Gao, X. Ren and G. Gao, Conductive Organohydrogels with Ultrastretchability, Antifreezing, Self-Healing, and Adhesive Properties for Motion Detection and Signal Transmission, ACS Appl. Mater. Interfaces, 2018, 11, 3428–3437 CrossRef.
- Q. Rong, W. Lei, J. Huang and M. Liu, Low Temperature Tolerant Organohydrogel Electrolytes for Flexible Solid-State Supercapacitors, Adv. Energy Mater., 2018, 8, 1801967 CrossRef.
- Z. Qin, D. Dong, M. Yao, Q. Yu, X. Sun, Q. Guo, H. Zhang, F. Yao and J. Li, Freezing-Tolerant Supramolecular Organohydrogel with High Toughness, Thermoplasticity, and Healable and Adhesive Properties, ACS Appl. Mater. Interfaces, 2019, 11, 21184–21193 CrossRef CAS.
- J. Song, S. Chen, L. Sun, Y. Guo, L. Zhang, S. Wang, H. Xuan, Q. Guan and Z. You, Mechanically and Electronically Robust Transparent Organohydrogel Fibers, Adv. Mater., 2020, 32, 1906994 CrossRef CAS.
- Y. Ding, J. Zhang, L. Chang, X. Zhang, H. Liu and L. Jiang, Preparation of High-Performance Ionogels with Excellent Transparency, Good Mechanical Strength, and High Conductivity, Adv. Mater., 2017, 29, 1704253 CrossRef.
- Z. Liu, Y. Wang, Y. Ren, G. Jin, C. Zhang, W. Chen and F. Yan, Poly(ionic liquid) hydrogel-based anti-freezing ionic skin for a soft robotic gripper, Mater. Horiz., 2020, 7, 919–927 RSC.
- H. Liu, X. Wang, Y. Cao, Y. Yang, Y. Yang, Y. Gao, Z. Ma, J. Wang, W. Wang and D. Wu, Freezing-Tolerant, Highly Sensitive Strain and Pressure Sensors Assembled from Ionic Conductive Hydrogels with Dynamic Cross-Links, ACS Appl. Mater. Interfaces, 2020, 12, 25334–25344 CrossRef CAS.
- R. Tong, G. Chen, D. Pan, J. Tian, H. Qi, R. A. Li, F. Lu and M. He, Ultrastretchable and Antifreezing Double-Cross-Linked Cellulose Ionic Hydrogels with High Strain Sensitivity under a Broad Range of Temperature, ACS Sustainable Chem. Eng., 2019, 7, 14256–14265 CrossRef CAS.
- S. Hong, Y. Yuan, C. Liu, W. Chen, L. Chen, H. Lian and H. Liimatainen, A stretchable and compressible ion gel based on a deep eutectic solvent applied as a strain sensor and electrolyte for supercapacitors, J. Mater. Chem. C, 2020, 8, 550–560 RSC.
Footnote |
† Electronic supplementary information (ESI) available. See DOI: 10.1039/d0qm00625d |
|
This journal is © the Partner Organisations 2021 |