DOI:
10.1039/D0QM00611D
(Research Article)
Mater. Chem. Front., 2021,
5, 304-314
The synthesis and characterization of Zn(II)/Cd(II) based MOFs by a mixed ligand strategy: a Zn(II) MOF as a dual functional material for reversible dye adsorption and as a heterogeneous catalyst for the Biginelli reaction†
Received
20th August 2020
, Accepted 29th September 2020
First published on 30th September 2020
Abstract
Two mixed ligand MOFs, {[Zn2(5NO2-IP)2(L)2](H2O)}n (ADES-1) and {[Cd2(5NO2-IP)2(L)2(H2O)4](L)(H2O)(CH3OH)6}n (ADES-2) (where 5NO2-IP = 5-nitroisophthalate and L = (E)-N′-(pyridin-3-ylmethylene)nicotinohydrazide), have been synthesized and characterized via various analytical methods, including SXRD analysis. SXRD analysis revealed that both MOFs adopt an identical two-dimensional network topology. ADES-1 with bulk phase purity has been synthesized via a conventional reflux method and its potential applications as a functional material for reversible dye adsorption and as an efficient heterogeneous catalyst for the Biginelli reaction were exploited. ADES-1 exhibited the rapid and efficient adsorption of both cationic and anionic dye molecules with a slight preference for methyl violet (86%) compared to the other dyes that were investigated. A separation study involving a mixture of dyes and the application of ADES-1 as a column filler for the separation of organic dyes from water are also demonstrated. Moreover, the catalytic performance of ADES-1 towards a multi-component Biginelli reaction to yield 3,4-dihydropyrimidin-2(1H)-ones (DHPM) derivative has been studied. Finally, studies of the utilization of ADES-1 for dye adsorption and its catalytic activity revealed good stability and reusability, enabling it to be a potential functional material, and possible dye adsorption and Biginelli reaction mechanisms are also proposed.
Introduction
Over the past two decades, significant progress has been witnessed in the development of porous materials, especially in the area of hybrid organic/inorganic porous materials popularly known as metal–organic frameworks (MOFs), which offer diverse applications and academic interest. Metal–organic frameworks (MOFs) have become very promising materials for a wide range of applications because of their unique features, such as topologies and interesting surface chemistry, porosity, robustness, and chemical and thermal stability. The precise design and fabrication of MOFs by a prudent choice of the metal nodes and functionally decorated ligands can offer anticipated properties because of their versatility and they can find diverse applications in the area of gas sorption/separation, magnetism, chemical probes, catalysis, etc.1–8 The detection as well as the safe and efficient removal of organic aromatic pollutants from industrial wastewater and water bodies remains a challenging task due to its ecological and environmental significance. Generally adopted methods for industrial wastewater treatment rely upon or are handled by the adsorption and/or coagulating out of the pollutants. Dyes, as an important class of non-degradable hazardous organic pollutants are extensively used in many industries, such as textiles, paper, plastics etc. and the discharge of untreated coloured wastewater to the surroundings can cause hazardous effects not only on the ecosystem but also on human health. Hence, cost-effective strategies for the efficient removal of organic dyes from aqueous systems is a challenge and a topic of research interest. A broad spectrum of techniques to remove dye from water includes adsorption, chemical oxidation, coagulation, membrane separation, biodegradation and photo-catalytic degradation. The adsorption method has captured much attention and materials like zeolites, activated carbon, resins, clay, and porous organic and inorganic materials have been explored as adsorbents owing to benefits such as high efficiency, cost-effectiveness and ease of operation.9–14 Characteristic features like chemical stability, tuneable structure, high specific surface area and porosity also provide MOFs with an important spot in the field of adsorption support and separation.15–20
Dyes as industrially important molecules are among the most abundant and frequently observed effluents which are composed of hazardous organic compounds and toxic substances that incessantly pollute our natural water resources. MOFs with the characteristic features mentioned earlier have been particularly exploited as a potential material for the adsorption/separation of hazardous dye molecules and catalytic applications. MOFs are advanced porous materials and their organic linkers can be functionally decorated to tune the adsorbate–MOF supramolecular interactions for the efficient adsorptive removal of hazardous pollutants from water, including dyes. Different supramolecular interactions, such as electrostatic, stacking, hydrogen bonding, and hydrophobic interactions are commonly proposed for the adsorptive mechanism.21–30 The tuneable porosity and framework charge associated with MOFs also play an important role in the adsorption and separation of neutral, anionic or cationic dye molecules.31–36 The chemical stability of MOF in solvents is crucial for the aqueous phase adsorption of dye molecules for practical applications. On the other hand, high surface areas together with regularly distributed active sites enable MOFs to be excellent candidates for catalytic applications. Among catalytic reactions, the Biginelli reaction, the three-component reaction between aldehyde, urea, and ethyl acetoacetate, is one of the most efficient ways to synthesize dihydropyrimidinone derivatives (DHPMs). The growing interest in this reaction is mainly due to Biginelli products (DHPMs) having therapeutic and pharmacological properties.37–39
We have been involved in the design and synthesis of multidimensional MOFs by a dual ligand strategy utilizing N-donor ligands besides multi-carboxylic acids as well as their applications in the areas of sensing hazardous molecules and catalysis. Recently, we have reported some mixed ligand MOFs as heterogenous catalysts for CO2 sequestration and luminescent MOFs for the selective sensing and detection of pollutant molecules.40–43 In continuation of our ongoing research, herein we report Zn(II)/Cd(II)-based 2D MOFs, {[Zn2(5NO2-IP)2(L)2](H2O)}n (ADES-1) and {[Cd2(5NO2-IP)2(L)2(H2O)4](L)(H2O)(CH3OH)6}n (ADES-2), (where 5NO2-IP = 5-nitroisophthalate and L = (E)-N′-(pyridin-3-ylmethylene)nicotinohydrazide) synthesized by a mixed ligand strategy. Single crystals of both MOFs were harvested by a layering method and bulk phase pure compound ADES-1 was synthesized by a reflux method using the respective metal salt and ligand precursors in an appropriate stoichiometry. Both MOFs were characterized by various analytical methods, including SXRD analysis. The present study outlines the synthesis of two MOFs, their characterization, structural details, and the application of ADES-1 for dye adsorption studies in aqueous media and as a heterogeneous catalyst for the Biginelli reaction to afford dihydroprimidinone derivatives, which are significant in the pharmaceutical industry.
Results and discussion
PXRD, IR and TGA analyses
Crystals and bulk product of ADES-1 obtained by a layering/conventional reflux method, and ADES-2 were characterized by different analytical methods besides X-ray crystallography. The bulk phase purity of ADES-1 synthesized by a conventional reflux method was confirmed by comparing the experimental PXRD profile with the simulated SXRD patterns of the crystals obtained by the diffusion method (Fig. S1, ESI†). The chemical compositions of ADES-1 and ADES-2 were also assessed and ascertained based on experimental data acquired from TGA, elemental analysis and SXRD analysis. The FTIR spectra of both MOFs were recorded by dispersing the respective compounds as KBr pellets (Fig. S2, ESI†). FTIR of ADES-1 and ADES-2 showed broad bands in the range 3410–3220 cm−1 with maxima at 3410, 3215 cm−1 and 3417, 3221 cm−1, which can be attributed to the O–H stretching modes of lattice water molecules and the amide –NH group. Strong bands at around 1292 cm−1 can be assigned to the stretching frequency of the nitro group of the bridging ligand 5NO2-IP. Sharp bands at ca. 1359, 1534 cm−1 and 1362, 1514 cm−1, respectively clearly indicate the characteristic carboxylate symmetric and antisymmetric νC
O bands. The difference in antisymmetric and symmetric carbonyl stretching frequencies Δν = 175 and 152 cm−1 indicates the chelating and bidentate coordination mode of carboxylate moieties in both MOFs. In an attempt to measure the thermal behaviour and stability of the MOFs, thermogravimetric analyses (TGA) were performed in the range of 25–800 °C in an inert atmosphere (Fig. S3, ESI†). The TG curve of ADES-1 exhibits a weight loss at 110 °C which corresponds to the expulsion of one lattice water molecule (cal. 3.35%, obs. 3.47%), followed by the gradual collapse of the framework at ∼330 °C. ADES-2 expelled about four methanol and one water molecules present in the lattice in the temperature range 40–150 °C (cal. 14.64%, obs. 14.75%). After the stepwise weight loss with a stable plateau in the range 200–320 °C, decomposition of the encapsulated L followed by the 2D network commences at ca. 340 °C. Surface area and porosity measurement of an activated sample of ADES-1 was performed by gas adsorption experiments at 77 K showing an adsorption–desorption isotherm with marginal hysteresis. The sorption isotherm of both materials is expressed in Fig. S4 and S5 (ESI†). The sorption isotherm of ADES-1 revealed a BET surface area of 10 m2 g−1 indicating the microporous nature of the material. The low surface area and porosity observed in the N2 isotherm may be due to the offset orientation of the alternate 2D networks in ABAB pattern effectively blocking the through channels. The pore size plot and CO2 adsorption isotherm were also measured for ADES-1 and are depicted in Fig. S6 and S7 (ESI†).
Crystal and molecular structures of {[Zn2(5NO2-IP)2(L)2](H2O)}n (ADES-1) and {[Cd2(5NO2-IP)2(L)2](H2O)(L)(CH3OH)6}n (ADES-2)
Two mixed-linker MOFs [X2(5NO2-IP)2(L)2] involving nitro-substituted isophthalate (5NO2-IP) and (E)-N′-(pyridin-3-ylmethylene)nicotinohydrazide (L) ligands with metals X = Zn(II)/Cd(II) were synthesized in bulk for ADES-1. Crystals suitable for SXRD analysis for both MOFs were harvested by a layering method. A summary of the crystallographic parameters/refinement details, selected bond lengths/angles and H-bonding interactions for both MOFs are tabulated and provided in supporting data as Tables S1–S3, respectively (ESI†). Both MOFs crystallize in a triclinic P
space group retaining identical framework topology and the detailed structural discussion is confined mainly to the zinc-based MOF (ADES-1). The asymmetric unit of ADES-1 is composed of one molecule each of the ligand moiety, one independent metal ion along with one water molecule as the solvent of crystallization. As depicted in Fig. 1a, the octahedral coordination around each metal ion is provided by the four carboxylate oxygens from three different 5NO2-IP ligand and pyridyl nitrogens at the axial position from two L moieties. Carboxylate oxygens constitute the square base with the Zn–O bond length ranging from 2.036(3) to 2.359(4) Å and the axial coordination with almost the same Zn–N bond length is provided by L (Zn(1)–N(1) = 2.132 (3) Å; Zn(1)–N(2) = 2.137(4) Å). As mentioned earlier, three 5NO2-IP ligands are involved in coordination with each metal ion and the 5NO2-IP moiety acts as a μ3-type linker.
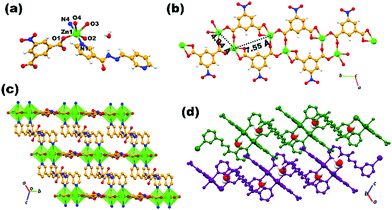 |
| Fig. 1 (a) The coordination environment around Zn(II) metal in ADES-1; (b) the [Zn2(COO)2] SBU observed in the 1D metal–carboxylate chain of ADES-1; (c) the double-lined 2D network in ADES-1; and (d) the ABAB manner of interlayering with lattice water molecules in ADES-1. | |
Thus, 5NO2-IP is involved in the μ3-κ2,κ1,κ1 mode of coordination in which carboxylate oxygens O1 and O2 are involved in the syn–anti mode bridging the neighbouring Zn(II) metals, generating a binuclear [Zn2(COO)2] secondary building unit (SBU), which is further extended as a one-dimensional double chain by chelate coordination of carboxylate oxygens O3 and O4 in connecting the binuclear SBUs (Fig. 1b). The Zn1⋯Zn1 distance within the dimeric unit is 4.04 Å and that between the nearest dimeric clusters of the double chain is 7.55 Å. Interestingly, the nitro group from 5NO2-IP exposed on the periphery of the double chain can probably be involved in supramolecular interaction with analytes and these materials may find application in sensing and adsorption. The double chain oriented along the bc plane is doubly pillared by the N-donor ligand L, generating a double-lined two-dimensional network, as portrayed in Fig. 1c. Axial coordination of the N-donor ligand L results in the [Zn2(5NO2-IP)2]n double chains being separated by a distance of 12.43 Å. The double chain layers are oriented in an offset ABAB manner, engendering a microporous structure with interlayer voids occupied by the lattice water molecules (Fig. 1c and d). Extensive hydrogen-bonding interaction does exist within the two-dimensional network. Supramolecular interactions in the form of hydrogen bonding, involving the carboxylate/nitro group of the zinc-isophthalate chains, amide functionality of the acylhydrazone ligand L and the lattice water molecule is responsible for the offset displacement of the 2D framework. Thus, water hydrogens H8A and H8B as donors to O8 in O–H⋯O interaction with chelated carboxylate oxygen O3 and nitro oxygen O5, as well as O8 as an acceptor in N–H⋯O contact with the amide hydrogen H2A assemble a hydrogen-bonded 3D network in bridging the adjacent sheets via interlayer interactions (Fig. S8, ESI†). In addition to the above-mentioned O–H⋯O and N–H⋯O interaction, weak C–H⋯O intermolecular contact between the pyridyl hydrogen H20 from L and the nitro oxygen O6 of 5NO2-IP stabilize the 3D hydrogen-bonded supramolecular architecture. In the case of ADES-2, the topology of the MOF network is identical and can be labelled as a double-walled rectangular net, as observed in the case of ADES-1. However, the asymmetric unit of ADES-2 occupies an additional half a molecule of L, having a dynamic disorder in which the amide oxygen with half occupancy resides at two positions, imposing a center of symmetry on L (Fig. 2a). Furthermore, L present in the lattice is encapsulated strongly between adjacent [Cd2(COO)2] dimeric clusters of a double-walled rectangular net involving strong π⋯π stacking interaction between the encapsulated pyridyl rings of the lattice and pillared L. Thus, the observed π⋯π stacking distances of the encapsulated ligand moiety are C1g⋯C3g = 3.63 Å and C2g⋯C3g = 3.94 Å, where C1g, C2g and C3g are the centroids of the pyridyl rings N1C9–C13, N4C16–C20 and N6C21–C25, respectively (Fig. S9, ESI†).
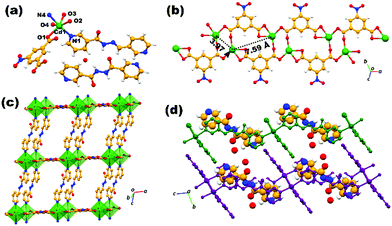 |
| Fig. 2 (a) The coordination environment around Cd(II) metal in ADES-2; (b) the [Cd2(COO)2] SBU observed in the 1D metal–carboxylate chain of ADES-2; (c) the double-lined 2D network in ADES-2; and (d) offset-stacked 2D layers with lattice water molecules and π⋯π stacked L in ADES-2. | |
The Cd⋯Cd distance observed within the dimeric SBU is 3.97 Å and between the neighbouring clusters is 7.59 Å and the pillaring distance of L with the [Cd2(5NO2-IP)2]n double chain is 14.70 Å (Fig. 2b and c). Hydrogens of the lattice water molecule O9 could not be located from the difference Fourier map and O9 acts as an acceptor by N–H⋯O and C–H⋯O contacts in bridging the neighbouring 2D nets. Thus, amide hydrogens H2C and H11C from the pyridyl ring of L are involved in the intermolecular N–H⋯O and C–H⋯O H-bonding (Fig. S10, ESI†). In addition to the above H-bonding, carboxylate oxygens O2 and O3 are making intermolecular C–H⋯O contacts with the pyridyl hydrogens from L in stabilizing the supramolecular assembly (Fig. 2d). Details of all pertinent hydrogen-bonding interaction for ADES-1 and ADES-2 with symmetry are provided in Table S3 (ESI†). It is worth mentioning here some recent reports based on mixed ligand MOFs involving acylhydrazone Schiff base and aromatic dicarboxylates and their structural and functional studies.44–46
Aqueous phase dye adsorption studies using ADES-1
Dyes are industrially important molecules extensively used in the textile, paper, printing, plastic, and leather industries and the discharge of organic dyes as effluents not only pollutes the environment but is also harmful to human beings and the animal kingdom. Dyes are difficult to degrade and stable against light/oxidation, and the removal of dyes from contaminated water is an acute challenge. The development of smart materials for separating and recycling the dyes either by an adsorption process or degradation by a photo-catalytic method is a prime ongoing research area. Considerable attention has been focused on investigating dye adsorption from solution by MOFs, thanks to their unique assets, such as chemical stability, tunability, porosity and topological aspects. Further, adsorption-based protocols are simple, cost-effective, and energy efficient and their specific attributes, including porosity and functionality, make MOFs suitable sorbents for a variety of dye molecules. In particular, ionic MOFs, cationic or anionic in nature, have distinct advantages for the selective adsorption of anionic or cationic dyes by various supramolecular interactions, such as host–guest and/or guest exchange interactions.47–50 Adsorption process of MOFs rely mainly on factors such as size selectivity, ion exchange and electrostatic/supramolecular interactions and also on the nature of the ionic framework, a vital element for the selective adsorption of cationic/anionic dye. There are few reports exploring neutral MOFs for the adsorption of ionic dyes and in the following investigation a functionally decorated highly stable Zn-based 2D neutral MOF and its reversible dye adsorption properties are outlined.
For the adsorption and separation studies, a combination of three cationic dyes, methyl violet (MV), methylene blue (MB), and rhodamine B (RhB) and an anionic dye, methyl orange (MO), were chosen (Scheme 1). As a preliminary check on the adsorption capacity of the MOF material, 100 mg of ADES-1 was added to 10 mL of an aqueous solution of the respective dye (5 × 10−5 M) and allowed to mix thoroughly at room temperature for 3 h under dark conditions by constant stirring. All the dye molecules irrespective of their charge showed a reduction in colour intensity after the stipulated time of 3 h of dye adsorption process in the presence of ADES-1. The reduction in colour strength of the dye molecules after the adsorption process clearly revealed no specific preference for adsorption by ADES-1 based on the nature and charge of the dye molecule (Fig. 3a). All cationic dyes (MV, MB, and RhB) as well as the anionic dye (MO) were adsorbed by ADES-1, which was reflected in naked eye detection as a reduced colour intensity, with a slight preference for MV from this series. The colour of the pristine ADES-1 sample completely changed to violet, blue, orange, or pink as the dye-adsorbed material (ADES-1@dye) in the presence of the respective dyes MV, MB, MO and RhB (Fig. 3b).
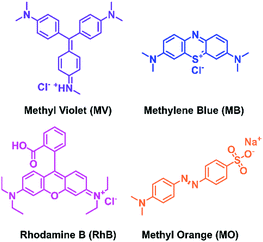 |
| Scheme 1 The chemical structures of the organic dyes used in this work. | |
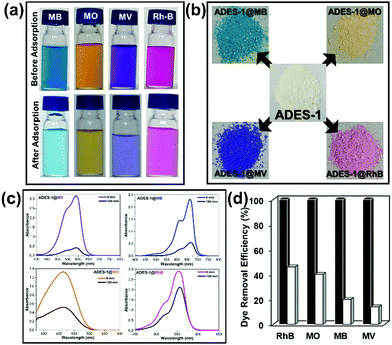 |
| Fig. 3 (a) Digital photographs showing colour changes of dye solutions before and after adsorption by ADES-1; (b) digital photographs of colour changes of the ADES-1 material after the adsorption of the respective dyes; (c) UV-vis spectral changes observed in different dye solutions after 3 h of stirring with ADES-1; and (d) the dye removal efficiency of ADES-1 with the respective dye after 3 h. | |
UV-vis spectrometry was used in the dye adsorption experiment to monitor and evaluate the decrease in dye concentration (Fig. 3c). The observed drops in dye concentration from the initial set concentration of 5 × 10−5 M were 7.17 × 10−6 M, 9.85 × 10−6 M, 1.98 × 10−5 M, and 2.32 × 10−5 M after 3 h of constant stirring for MV, MB, MO and RhB, respectively. The decreasing adsorption capacity by ADES-1 towards the dye molecules in the present investigation is in the order MV > MB > MO > RhB and the calculated adsorption efficiencies (η) from eqn (S2) (ESI†) for MV, MB, MO and RhB are 86%, 80%, 60% and 54%, respectively, indicating no specific adsorption trend based on the charge of the dye molecule (Fig. 3d). To evaluate the amount of dye adsorbed by ADES-1 in a given time interval, a calibration curve using standard aqueous solutions of the respective dyes was implemented (Fig. S11, ESI†). The calculated adsorption amounts of the respective dyes (qe, mg g−1) from eqn (S1) (ESI†) were found to be 1.75, 1.28, 0.99 and 1.28 mg g−1 for MV, MB, MO and RhB. Upon an increase in the concentration of the dye solution (i.e. from 5 × 10−5 M to 10−4 M) the adsorption efficiency also increased with respective calculated adsorption amounts of 4.03, 3.24, 3.26 and 3.33 mg g−1. In brief, the adsorption experiments clearly indicate that the neutral framework ADES-1 is a potential candidate for the efficient removal of hazardous dye pollutants. As mentioned earlier, the colour of the off-white pristine ADES-1 changed upon dye adsorption and the materials obtained are designated ADES-1@MV, ADES-1@MB, ADES-1@MO and ADES-1@RhB. All these ADES-1@dye materials obtained were further characterized by various analytical methods, such as solid-state UV-Vis, FTIR spectra and TG analysis (Fig. S12–S15, ESI†). The morphology and structural integrity of ADES-1 before and after dye adsorption were studied by FE-SEM and PXRD analysis. As depicted in Fig. 4, FE-SEM micrographs of both pristine MOFs showed their microcrystalline nature and upon MB and MO adsorption they showed agglomeration with ADES-1 generating a spherical morphology in the ADES-1@dye composite.
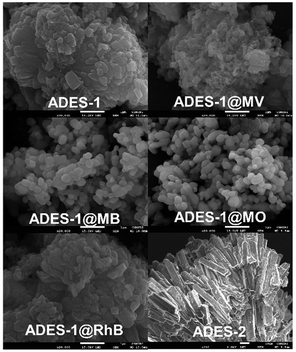 |
| Fig. 4 FE-SEM images of ADES-1 before/after the adsorption of the respective dyes and ADES-2. | |
Adsorption of MV and RhB by ADES-1 also revealed a morphological change with a lower agglomeration rate causing disruption of the microcrystalline nature. Preservation of the framework structure is witnessed from the PXRD data of ADES-1@dye, articulating that the MOF moiety retains its chemical and structural integrity, one of the prime requirements for an adsorption material (Fig. S15, ESI†). Adsorption and selectivity of targeted dyes by developing smart functionally decorated MOF material is a promising area of research not only of academic interest but also considering the functional utility. For example, the recovery of organic dyes from industrial waste by MOF via reversible adsorption is a step towards water preservation and environmental protection.
Among the different pools of dyes used in the present investigation, it is observed that RhB is least adsorbed (54%) by ADES-1 and a separation study involving a mixture of dyes was performed. For this experiment RhB was engaged with the other three dye molecules (MV, MB and MO) in an equimolar ratio (1
:
1, 10 mL) for separate batch studies. To this mixture of organic aqueous dye solution 100 mg of ADES-1 was added, and stirred vigorously for 3 h under dark conditions. The absorption intensity of the respective dyes MV (∼588 nm) MB (∼664 nm), MO (∼464 nm) and RhB (∼556 nm) was monitored with a UV-vis spectrophotometer from the batch of mixtures of dyes for selective adsorption by ADES-1. UV-vis spectra revealed a marginal decrease in absorption intensity of RhB (∼556 nm) but a complete/significant decrease in absorption intensity for MV and MB followed by MO from the mixture of dye solutions (Fig. 5a and b). This is also evidenced by naked eye visualization of the mixed dye solution after 3 h of continuous stirring showing a pink/light orange colour indicating the presence of RhB/RhB + trace of MO in the residual discharged solution (Fig. 5a and b). Spectroscopic and visual detection studies clearly demonstrate the efficiency of ADES-1 for the preferential adsorption of MV from the selected pool of dye molecules explored.
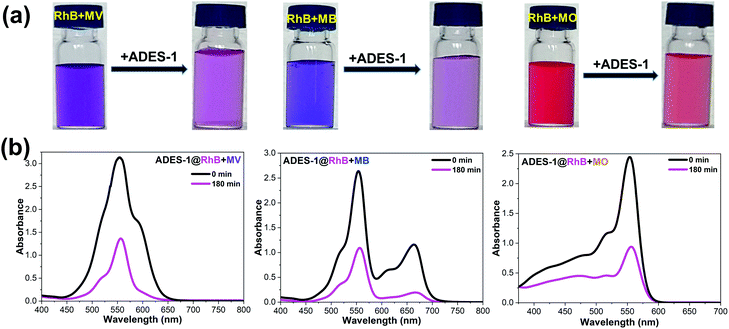 |
| Fig. 5 (a) Digital photographs and (b) UV-vis spectral changes presenting the higher adsorption of MV, MB, and MO over RhB by ADES-1. | |
Inspiration from the dye adsorption results by spectroscopic methods encouraged us to make a more practical application of ADES-1 as a column filler for the separation of organic dyes from water. Column preparation and other details are provided in the Experimental section. Almost complete adsorption of the dyes by ADES-1 was observed when 10−4 M solutions of individual aqueous dye solutions of MV, MB and MO were allowed to pass through the column while only partial adsorption by the column material had taken place in the case of RhB. This clearly echoed the colourless solution collected in the case of MV, MB and MO and the pink solution in the case of RhB after passing through the column (Fig. 6a–d).
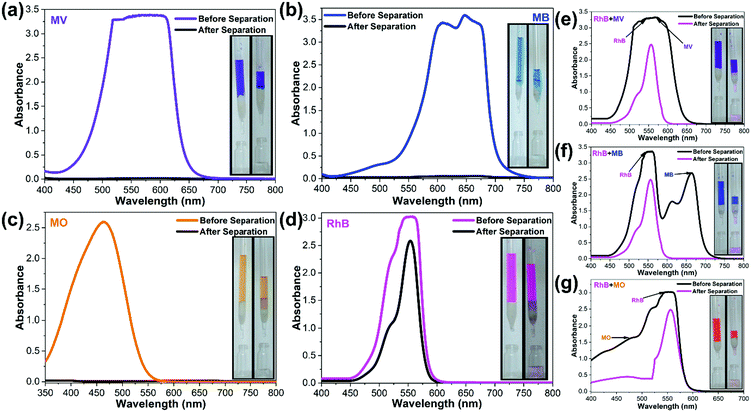 |
| Fig. 6 (a–d) UV-vis spectral and visual colour (inset) changes observed in aqueous solutions of individual dyes before and after separation; and (e–g) UV-vis spectral and visual colour changes (inset) showing the selective separation of MV, MB, and MO from RhB using a column of ADES-1. | |
Further, to supplement the adsorption results from spectroscopic methods of mixed-dye by ADES-1, similar column experiments were conducted by passing streams of dye combinations (RhB + MV, RhB + MB and RhB + MO). The elute collected after passing through the column showed a pale pink colour, indicating the presence of RhB in the discharge solution, reinforcing the separation as well as the capture of MV, MB and MO in the column filler (Fig. 6e–g).
A probable mechanism for dye adsorption based on the crystal structure analysis has been proposed. The low surface area and porosity of ADES-1 ruled out the possibility of dye molecule adsorption by encapsulation. However, the layered gap between the offset-stacked 2D network can probably accommodate the dye molecule involving various supramolecular as well as electrostatic interactions on the surface of the MOF.51
Crystal structure analysis of ADES-1 revealed that the polar nitro group from 5NO2-IP exposed on the periphery of the double chain can create an anionic cloud and is probably involved in electrostatic interaction with the cationic dyes in the adsorption process. Higher adsorption of MV (86%) and MB (80%) compared to RhB (54%) can be justified due to the diffusion of the bulky RhB being too low to make an effective electrostatic interaction. Interestingly, good adsorption of anionic dye MO (60%) can be attributed to the hydrogen-bonding interaction between the amide hydrogen from L with the sulfonate oxygen of the MO on the surface of the ADES-1 host by accommodating the dye molecule between the 2D layers. Since the adsorbent and adsorbate possess an aromatic moiety, π⋯π stacking also probably plays an important role in the adsorption process. A schematic representation of the probable mechanism for cationic and anionic dyes is depicted in Fig. 7. To reinforce this claim we further performed zeta potential measurements of ADES-1 in aqueous solution. The negative zeta potential (−5.8 mV) observed for ADES-1 in aqueous solution clearly demonstrates that strong electrostatic attractions between cationic dye molecules and the highly negative charged surface of ADES-1 play a vital role in the adsorption process. The desorption process is equally important in the case of reversible adsorption of hazardous molecules as a functional material for practical applications. In the present investigation, dye release experiments were performed by soaking the dye-adsorbed materials ADES-1@dye in methanol as a dye-release medium for 90 minutes. Visual images of the progressive release of MB to methanol and the enhancement in the intensity at 652 nm corresponding to MB are shown in Fig. 8a–c. Release studies performed in a similar way for other dyes also revealed the steady release of the dye molecules over time, as shown in digital photographs (Fig. S16–S18, ESI†).
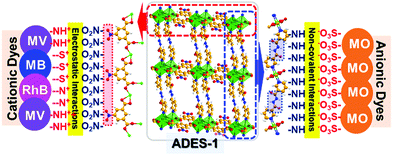 |
| Fig. 7 A schematic representation of the possible dye adsorption mechanism of ADES-1. | |
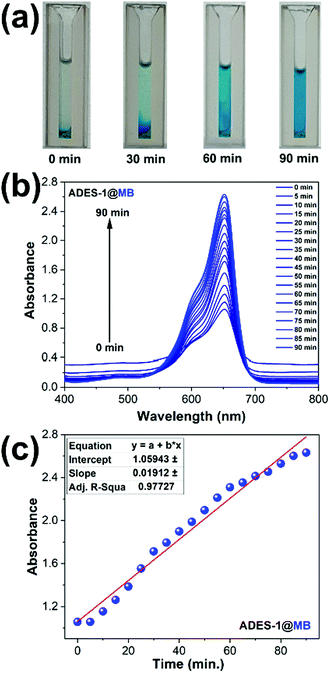 |
| Fig. 8 (a) Digital photographs demonstrating a gradual colour change due to the release of MB in 1 mL of MeOH after soaking 20 mg of ADES-1@MB; (b) time-dependent UV-vis spectra representing the steady increase in absorbance (λmax = 652 nm) due to the release of MB over time; and (c) the linear fit of absorbance vs. time for the release of MB over a 90 min period. | |
Catalytic activity of Zn-MOF in the Biginelli reaction
Application of MOFs as a heterogeneous catalyst to facilitate a wide range of reactions including multi-component reactions to synthesize medicinally important heterocycles is a potential area of ongoing research.52–57 Among multi-component reactions, the Biginelli reaction attracts much attention due to its medicinally important final product, 3,4-dihydropyrimidin-2(1H)-ones (DHPMs), which possesses significant biological properties, such as antitubercular, antiviral, antifungal, antitumor, antibacterial, and anti-inflammatory activities.58–62 Its notable features, such as excellent thermal and chemical stability, the presence of uniformly distributed metallic sites, and the functionally decorated N-donor linker of the framework may favour ADES-1 as an ideal candidate for heterogeneous catalysis. Therefore, we decided to exploit the catalytic performance of ADES-1 towards the multi-component Biginelli reaction involving condensation of aromatic aldehydes, ethyl acetoacetate and urea to yield 3,4-dihydropyrimidin-2(1H)-ones (DHPMs) (Scheme 2).
 |
| Scheme 2 The Biginelli reaction of aldehyde derivatives with ethyl acetoacetate and urea to produce 3,4-dihydropyrimidin-2(1H)-one (DHPM) derivatives. | |
To optimize the reaction conditions, the condensation of benzaldehyde, ethyl acetoacetate and urea was chosen as a model reaction. A series of experiments was conducted to examine the influence of different parameters like the amount of catalyst, temperature, solvent and reaction time. The obtained results are summarized in Table 1 for the model reaction. Initially the model reaction was performed at 80 °C under solvent-free conditions with a catalyst loading of 0.005 mmol and the reaction was completed with poor conversion (22.8%) to yield ethyl 6-methyl-2-oxo-4-phenyl-1,2,3,4-tetrahydropyrimidine-5-carboxylate within 10 min (Table 1, entry 1).
Table 1 Optimization of the reaction conditions for the Biginelli reactiona
Entry |
Catalyst (mmol) |
Temp. (°C) |
Solvent |
Conversion (%) |
Time (min) |
Reaction conditions: benzaldehyde (1.0 mmol), ethyl acetoacetate (1.0 mmol), urea (1.5 mmol), solvent (2.0 mL).
With catalyst ADES-2.
|
1 |
0.005 |
80 |
None |
22.8 |
10 |
2 |
0.010 |
80 |
None |
73.2 |
10 |
3 |
0.015 |
80 |
None |
93.6 |
10 |
4 |
0.020 |
80 |
None |
98.3 |
10 |
5 |
0.025 |
80 |
None |
93.2 |
10 |
6 |
0.020 |
60 |
None |
86.5 |
20 |
7 |
0.020 |
70 |
None |
91.3 |
10 |
8 |
0.020 |
90 |
None |
98.1 |
10 |
9 |
0.020 |
80 |
CH2Cl2 |
82.2 |
10 |
10 |
0.020 |
80 |
Toluene |
76.2 |
120 |
11 |
0.020 |
80 |
EtOH |
86.7 |
60 |
12b |
0.020 |
80 |
None |
97.4 |
10 |
To check the effect of catalyst loading, four different experiments were performed with 0.010, 0.015, 0.020 and 0.025 mmol of catalyst under similar conditions (Table 1, entries 2–5). In the presence of 0.020 mmol of catalyst, the conversion observed was 98.3% within the set reaction time of 10 min and a further increase in catalytic amount tended to decrease the conversion. Further, we also examined the influence of temperature and different solvents like CH2Cl2, toluene and EtOH, while keeping the catalyst amount at 0.020 mmol. Notably, at temperatures lower than 80 °C or in the presence of organic solvents the reaction advanced with a reduction in conversion and employing a longer reaction time (Table 1, entries 6–11). A representative reaction by ADES-2 for the model substrate benzaldehyde with ethyl acetoacetate and urea was conducted, which showed similar efficiency (97.8%) for the product conversion (Table 1, entry 12). From the preliminary optimization reaction, the following conditions were set for the product scope with solvent-free reaction using 0.020 mmol of the ADES-1 catalyst at 80 °C with the substrate concentration mentioned in Table 1. To check the scope and generality of the ADES-1 catalyst for the Biginelli reaction, the condensation of different substituted aromatic aldehydes with ethyl acetoacetate and urea was performed under the set optimized reaction conditions and the obtained results are abridged in Table 2. Indeed, all the aldehydes with electron withdrawing and donating groups gave a good product yield within 8 to 12 min. The formation of the desired Biginelli product, dihydropyrimidinone (DHPMs), was confirmed by 1H NMR and 13C NMR spectroscopy (Fig. S19–S32, ESI†). The ADES-1 catalyst showed good recyclability up to 6 cycles showing no appreciable reduction in product yield (Fig. S33, ESI†). After each cycle, the catalyst was separated by filtration and washed with methanol/water (1/1) followed by acetone and dried to regenerate the active catalyst. The recovered catalyst after six cycles revealed the integrity of the framework, showing identical PXRD and FTIR patterns to the pristine sample (Fig. S34 and S35, ESI†). A plausible mechanism for the Biginelli reaction can be elucidated on the basis of the formation of an N-acyliminium ion intermediate similar to the mechanism proposed by Kappe.63 The crystal structure of the ADES-1 catalyst revealed the presence of weakly coordinated chelated carboxylate which can open up and act as a Lewis acidic site and the existence of amide decorated L as a Lewis basic site can probably concomitantly activate the substrates in the formation of an N-acyliminium ion intermediate from the reaction between aldehyde and urea. Subsequently, nucleophilic attack on the N-acyliminium ion by the enol tautomer of ethyl acetoacetate yielded ureide. In the last step, the ureide intermediate undergoes cyclodehydration to generate the desired DHPM product. Therefore, ADES-1 acts as an efficient heterogeneous catalytic material exhibiting an excellent product yield for both electron donating and withdrawing aromatic substrates in the Biginelli reaction at 80 °C under solvent-free mild reaction conditions, which is comparable with some recently reported MOF catalysts.64–68
Table 2 Summary of Biginelli reactions involving aromatic aldehydes with ethyl acetoacetate and urea for the synthesis of DHPM derivativesa
Entry |
Aldehyde |
Product |
Time (min) |
Yieldb (%) |
Reaction conditions: aromatic aldehyde (1 mmol), ethyl acetoacetate (1 mmol), urea (1.5 mmol) and MOF (0.020 mmol).
Isolated yields.
|
1 |
|
|
10 |
94 |
2 |
|
|
8 |
92 |
3 |
|
|
10 |
91 |
4 |
|
|
12 |
88 |
5 |
|
|
8 |
94 |
6 |
|
|
8 |
93 |
7 |
|
|
10 |
95 |
Conclusions
In summary, the Zn(II)/Cd(II)-based mixed ligand MOFs {[Zn2(5NO2-IP)2(L)2](H2O)}n (ADES-1) and {[Cd2(5NO2-IP)2(L)2(H2O)4](L)(H2O)(CH3OH)6}n (ADES-2), composed of a functionally decorated N-donor ligand L and rigid 5-nitroisophthalate, have been synthesized and characterized via various analytical methods, including SXRD analysis. The crystal structure revealed that both MOFs retain an identical 2D network topology composed of an [M2(5NO2-IP)2]n double-layered motif connected via the terminal nitrogen of L. The bulk material of ADES-1 was produced via a conventional reflux method and the phase purity of this material was established based on a comparison of its PXRD data with simulated SXRD data. Further, the functional utility of ADES-1 was established for the reversible adsorption of dyes and as an efficient heterogeneous catalyst for the Biginelli reaction to yield pharmaceutically important dihydropyrimidinone (DHPM) derivatives. Adsorption studies of mixtures of dyes with ADES-1 as a chromatographic filler for the separation of dyes were also demonstrated. The probable mechanisms for dye adsorption and catalytic activity using ADES-1 were proposed. The concomitant effect of electrostatic/supramolecular interaction between the adsorbent and adsorbate is responsible for the adsorption of a variety of dye molecules. Uniformly distributed metal centres, which can act as Lewis acids, the acyl amide functional group of the N-donor ligand, as a Lewis basic site, and the polar nitro group of the dicarboxylic acid in tandem are instrumental for reversible dye adsorption and the efficient catalytic activity of ADES-1 as a multi-functional material. Overall, the present investigation will be useful for the preparation of multi-functional MOFs as adsorbents for the remediation of hazardous dye molecules and as efficient catalysts for the synthesis of DHPM derivatives via the Biginelli reaction, which has relevance in the pharmaceutical industry.
Conflicts of interest
There are no conflicts to declare.
Acknowledgements
The registration number of this publication is CSIR-CSMCRI – 133/2020. Financial support received from INSA under Visiting Scientist Programme 2019 and SERB-DST under Teachers Associateship for Research Excellence (Ref. No. TAR/2019/000096) (AD), CHARUSAT PhD Scholars’ Fellowship (CPSF) (UP), and CSIR-SRF (BP and PP), and analytical support from AESD&CIF of CSIR-CSMCRI and P. D. Patel Institute of Applied Sciences, Charotar University of Science and Technology are gratefully acknowledged. We thank Dr Vinod Boricha for NMR data, Mr Jayesh Chaudhari for FE-SEM analysis, and Mr Parag R. Vala for N2-adsorption/desorption analysis.
Notes and references
- H.-Y. Guan, R. J. LeBlanc, S.-Y. Xie and Y. Yue, Recent progress in the syntheses of mesoporous metal–organic framework materials, Coord. Chem. Rev., 2018, 369, 76–90 CrossRef CAS.
- H. Furukawa, K. E. Cordova, M. O’Keeffe and O. M. Yaghi, The chemistry and applications of metal-organic frameworks, Science, 2013, 341, 1230444 CrossRef.
- G. Férey, Hybrid porous solids: past, present, future, Chem. Soc. Rev., 2008, 37, 191–214 RSC.
- M. Kurmoo, Magnetic metal-organic frameworks, Chem. Soc. Rev., 2009, 38, 1353–1379 RSC.
- Z. Hu, B. J. Deibert and J. Li, Luminescent metal-organic frameworks for chemical sensing and explosive detection, Chem. Soc. Rev., 2014, 43, 5815–5840 RSC.
- B. Parmar, K. K. Bisht, Y. Rachuri and E. Suresh, Zn(II)/Cd(II) based mixed ligand coordination polymers as fluorosensors for aqueous phase detection of hazardous pollutants, Inorg. Chem. Front., 2020, 7, 1082–1107 RSC.
- S. Ma and H.-C. Zhou, A metal-organic framework with entatic metal centers exhibiting high gas adsorption affinity, J. Am. Chem. Soc., 2006, 128, 11734–11735 CrossRef CAS.
- A. Dhakshinamoorthy and H. Garcia, Catalysis by metal nanoparticles embedded on metal-organic frameworks, Chem. Soc. Rev., 2012, 41, 5262–5284 RSC.
- V. K. Gupta and Suhas, Application of low-cost adsorbents for dye removal – a review, J. Environ. Manage., 2019, 90, 2313–2342 CrossRef.
- V. Vimonses, S. Lei, B. Jin, C. W. K. Chow and C. Saint, Kinetic study and equilibrium isotherm analysis of congo red adsorption by clay materials, Chem. Eng. J., 2009, 148, 354–364 CrossRef CAS.
- M. Maruthapandi, J. H. T. Luong and A. Gedanken, Kinetic, isotherm and mechanism studies of organic dye adsorption on poly(4,4′-oxybisbenzenamine) and copolymer of poly(4,4’-oxybisbenzenamine-pyrrole) macro-nanoparticles synthesized by multifunctional carbon dots, New J. Chem., 2019, 43, 1926–1935 RSC.
-
E. Worch, Adsorption technology in water treatment: fundamentals, processes, and modeling, Walter de Gruyter, GmbH & Co. KG, Berlin, 2012 Search PubMed.
- A. Walcarius and L. Mercier, Mesoporous organosilica adsorbents: nanoengineered materials for removal of organic and inorganic pollutants, J. Mater. Chem., 2010, 20, 4478–4511 RSC.
- A. Mahto, A. Kumar, J. P. Chaudhary, M. Bhatt, A. K. Sharm, P. Paul, S. K. Nataraj and R. Meena, Solvent-free production of nano-FeS anchored graphene from Ulva fasciata: a scalable synthesis of super-adsorbent for lead, chromium and dyes, J. Hazard. Mater., 2018, 353, 190–203 CrossRef CAS.
- C.-C. Wang, J.-R. Li, X.-L. Lv, Y.-Q. Zhang and G. Guo, Photocatalytic organic pollutants degradation in metal–organic frameworks, Energy Environ. Sci., 2014, 7, 2831–2867 RSC.
- N. A. Khan, Z. Hasan and S. H. Jhung, Adsorptive removal of hazardous materials using metal-organic frameworks (MOFs): a review, J. Hazard. Mater., 2012, 244–245, 444–456 Search PubMed.
- J.-R. Li, J. Sculley and H.-C. Zhou, Metal-organic frameworks for separation, Chem. Rev., 2012, 112, 869–932 CrossRef CAS.
- J. Tan, B. Zhou, C. Liang, H. Zinky, H.-L. Zhou and Y.-B. Zhang, Secondary-amine-functionalized isoreticular metal–organic frameworks for controllable and selective dye capture, Mater. Chem. Front., 2018, 2, 129–135 RSC.
- D. Jiang, M. Chen, H. Wang, G. Zeng, D. Huang, M. Cheng, Y. Liu, W. Xue and Z. W. Wang, The application of different typological and structural MOFs-based materials for the dyes adsorption, Coord. Chem. Rev., 2019, 380, 471–483 CrossRef CAS.
- I. Mantasha, S. Hussain, M. Ahmad and M. Shahid, Two dimensional (2D) molecular frameworks for rapid and selective adsorption of hazardous aromatic dyes from aqueous phase, Sep. Purif. Technol., 2020, 238, 116413 CrossRef CAS.
- Z. Hasan and S. H. Jhung, Removal of hazardous organics from water using metal-organic frameworks (MOFs): plausible mechanisms for selective adsorptions, J. Hazard. Mater., 2015, 283, 329–339 CrossRef CAS.
- S. M. Khan, M. Khalid and M. Shahid, What triggers dye adsorption by metal organic frameworks? The current perspectives, Mater. Adv., 2020, 1, 1575–1601, 10.1039/D0MA00291G.
- C. Li, Z. Xiong, J. Zhang and C. Wu, The Strengthening Role of the Amino Group in Metal–Organic Framework MIL-53 (Al) for Methylene Blue and Malachite Green Dye Adsorption, J. Chem. Eng. Data, 2015, 60, 3414–3422 CrossRef CAS.
- A. Ayati, M. N. Shahrak, B. Tanhaei and M. Sillanpaa, Emerging adsorptive removal of azo dye by metal–organic frameworks, Chemosphere, 2016, 160, 30–44 CrossRef CAS.
- S. A. A. Razavi, M. Y. Masoomi and A. Morsali, Host–Guest Interaction Optimization through Cavity Functionalization for Ultra-Fast and Efficient Water Purification by a Metal–Organic Framework, Inorg. Chem., 2018, 57, 11578–11587 CrossRef CAS.
- Z. Wang, J.-H. Zhang, J.-J. Jiang, H.-P. Wang, Z.-W. Wei, X. Zhu, M. Pan and C.-Y. Su, A stable metal cluster-metalloporphyrin MOF with high capacity for cationic dye removal, J. Mater. Chem. A, 2018, 6, 17698–17705 RSC.
- W. Yan, L.-J. Han, H.-L. Jia, K. Shen, T. Wang and H.-G. Zheng, Three Highly Stable Cobalt MOFs Based on “Y”-Shaped Carboxylic Acid: Synthesis and Absorption of Anionic Dyes, Inorg. Chem., 2016, 55, 8816–8821 CrossRef CAS.
- S. Wan, L. Li, J. Liu, B. Liu, G. Li, L. Zhang and Y. Liu, An Imide-Decorated Indium-Organic Framework for Efficient and Selective Capture of Carcinogenic Dyes with Diverse Adsorption Interactions, Cryst. Growth Des., 2020, 20, 3199–3207 CrossRef CAS.
- Z. Wang, C.-Y. Zhu, H.-S. Zhao, S.-Y. Yin, S.-J. Wang, J.-H. Zhang, J.-J. Jiang, M. Pan and C.-Y. Su, Record high cationic dye separation performance for water sanitation using a neutral coordination framework, J. Mater. Chem. A, 2019, 7, 4751–4758 RSC.
- W.-Q. Tong, W.-N. Liu, J.-G. Cheng, P.-F. Zhang, G. Li, L. Hou and Y.-Y. Wang, A new stable luminescent Cd(II) metal–organic framework with fluorescent sensing and selective dye adsorption properties, Dalton Trans., 2018, 47, 9466–9473 RSC.
- Q. Gao, J. Xu and X.-H. Bu, Recent advances about metal–organic frameworks in the removal of pollutants from wastewater, Coord. Chem. Rev., 2019, 378, 17–31 CrossRef CAS.
- J. A. Chiong, J. Zhu, J. B. Bailey, M. Kalaj, R. Subramanian, W. Xu, S. M. Cohen and F. A. Tezcan, An Exceptionally Stable Metal-Organic Framework Constructed from Chelate-based Metal-Organic Polyhedra, J. Am. Chem. Soc., 2020, 142, 6907–6912 CrossRef CAS.
- X. Zhang, B. Xiong, J. Li, L. Qian, L. Liu, Z. Liu, P. Fang and C. He, Dependence of Dye Molecules Adsorption Behaviors on Pore Characteristics of Mesostructured MOFs Fabricated by Surfactant Template, ACS Appl. Mater. Interfaces, 2019, 11, 31441–31451 CrossRef CAS.
- Y.-Z. Li, G.-D. Wang, H.-Y. Yang, L. Hou, Y.-Y. Wang and Z. Zhu, Novel cage-like MOF for gas separation, CO2 conversion and selective adsorption of an organic dye, Inorg. Chem. Front., 2020, 7, 746–755 RSC.
- X. Li, B. Wang, Y. Cao, S. Zhao, H. Wang, X. Feng, J. Zhou and X. Ma, Water
Contaminant Elimination Based on Metal–Organic Frameworks and Perspective on Their Industrial Applications, ACS Sustainable Chem. Eng., 2019, 7, 4548–4563 CrossRef CAS.
- J. Liu, Z. Wang, R. Bi, F. Mao, K. Wang, H. Wu and X. Wang, A polythreaded MnII-MOF and its super-performances for dye adsorption and supercapacitors, Inorg. Chem. Front., 2020, 7, 718–730 RSC.
- C. O. Kappe, Recent Advances in the Biginelli Dihydropyrimidine Synthesis. New Tricks from an Old Dog, Acc. Chem. Res., 2000, 33, 879–888 CrossRef CAS.
- C. O. Kappe, Biologically active dihydropyrimidones of the Biginelli-type—a literature survey, Eur. J. Med. Chem., 2000, 35, 1043–1052 CrossRef CAS.
-
A. Fatima, B. S. Terra, L. S. Neto and T. C. Braga, in Green Synthetic Approaches for Biologically Relevant Heterocycles, ed. G. Brahmachari, Elsevier Inc., Netherlands, 2015, ch. 12, pp. 317–337 Search PubMed.
- P. Patel, B. Parmar, R. S. Pillai, A. Ansari, N. H. Khan and E. Suresh, CO2 Fixation by Cycloaddition of Mono/Disubstituted Epoxides using Acyl Amide Decorated Co(II) MOF as a Synergistic Heterogeneous Catalyst, Appl. Catal., A, 2020, 590, 117375 CrossRef CAS.
- B. Parmar, P. Patel, R. S. Pillai, R. I. Kureshy, N. H. Khan and E. Suresh, Efficient Catalytic Conversion of Terminal/Internal Epoxides to Cyclic Carbonates by Porous Co(II) MOF at Ambient Conditions: Structure Property Correlation and Computational Studies, J. Mater. Chem. A, 2019, 7, 2884–2894 RSC.
- Y. Rachuri, B. Parmar and E. Suresh, Three-Dimensional Co(II)/Cd(II) Metal–Organic Frameworks: Luminescent Cd-MOF for Detection and Adsorption of 2,4,6-Trinitrophenol in the Aqueous Phase, Cryst. Growth Des., 2018, 18, 3062–3072 CrossRef CAS.
- B. Parmar, Y. Rachuri, K. K. Bisht and E. Suresh, Mixed-Ligand LMOF Fluorosensors for Detection of Cr(VI) Oxyanions and Fe3+/Pd2+ Cations in Aqueous Media, Inorg. Chem., 2017, 56, 10939–10949 CrossRef CAS.
- K. Roztocki, I. Senkovska, S. Kaskel and D. Matoga, Carboxylate–Hydrazone Mixed-Linker Metal–Organic Frameworks: Synthesis, Structure, and Selective Gas Adsorption, Eur. J. Inorg. Chem., 2016, 4450–4456 CrossRef CAS.
- K. Roztocki, D. Jędrzejowski, M. Hodorowicz, I. Senkovska, S. Kaskel and D. Matoga, Effect of Linker Substituent on Layers Arrangement, Stability, and Sorption of Zn-Isophthalate/Acylhydrazone Frameworks, Cryst. Growth Des., 2018, 18, 488–497 CrossRef CAS.
- V. Safarifard and A. Morsali, Influence of an amine group on the highly efficient reversible adsorption of iodine in two novel isoreticular interpenetrated pillared-layer microporous metal–organic frameworks, CrystEngComm, 2014, 16, 8660–8663 RSC.
- J. Jia, F. Sun, T. Borjigin, H. Ren, T. Zhang, Z. Bian, G. Zhu and L. Gao, Highly porous and robust ionic MOFs with nia topology constructed by connecting an octahedral ligand and a trigonal prismatic metal cluster, Chem. Commun., 2012, 48, 6010–6012 RSC.
- L. Liu, X.-N. Zhang, Z.-B. Han, M.-L. Gao, X.-M. Cao and S.-M. Wang, An InIII-based anionic metal−organic framework: sensitization of lanthanide (III) ions and selective absorption and separation of cationic dyes, J. Mater. Chem. A, 2015, 3, 14157–14164 RSC.
- B.-Q. Song, X.-L. Wang, Y.-T. Zhang, X.-S. Wu, H.-S. Liu, K.-Z. Shao and Z.-M. Su, Periodic tiling of triangular and square nanotubes in a cationic metal–organic framework for selective anion exchange, Chem. Commun., 2015, 51, 9515–9518 RSC.
- P. Samanta, A. V. Desai, S. Let and S. K. Ghosh, Advanced Porous Materials for Sensing, Capture and Detoxification of Organic Pollutants toward Water Remediation, ACS Sustainable Chem. Eng., 2019, 7, 7456–7478 CrossRef CAS.
- Y. Rachuri, S. Subhagan, B. Parmar, K. K. Bisht and E. Suresh, Selective and reversible adsorption of cationic dyes by mixed ligand Zn(II)
coordination polymers synthesized by reactant ratio modulation, Dalton Trans., 2018, 47, 898–908 RSC.
- F.-J. Zhao, G. Zhang, Z. Ju, Y.-X. Tan and D. Yuan, The Combination of Charge and Energy Transfer Processes in MOFs for Efficient Photocatalytic Oxidative Coupling of Amines, Inorg. Chem., 2020, 59, 3297–3303 CrossRef CAS.
- S. Chand, S. C. Pal, M. Mondal, S. Hota, A. Pal, R. Sahoo and M. C. Das, Three-Dimensional Co(II)-Metal–Organic Frameworks with Varying Porosities and Open Metal Sites toward Multipurpose Heterogeneous Catalysis under Mild Conditions, Cryst. Growth Des., 2019, 19, 5343–5353 CrossRef CAS.
- B. Parmar, P. Patel, R. S. Pillai, R. K. Tak, R. I. Kureshy, N. H. Khan and E. Suresh, Cycloaddition of CO2 with Epoxide Bearing Oxindole Scaffold by MOF Based Heterogeneous Catalyst at Ambient Condition, Inorg. Chem., 2019, 58, 10084–10096 CrossRef CAS.
- Z. Shao, M. Liu, J. Dang, C. Huang, W. Xu, J. Wu and H. Hou, Efficient Catalytic Performance for Acylation-Nazarov Cyclization Based on an Unusual Postsynthetic Oxidization Strategy in a Fe(II)-MOF, Inorg. Chem., 2018, 57, 10224–10231 CrossRef CAS.
- R. A. Agarwal, A. K. Gupta and D. De, Flexible Zn-MOF Exhibiting Selective CO2 Adsorption and Efficient Lewis Acidic Catalytic Activity, Cryst. Growth Des., 2019, 19, 2010–2018 CrossRef CAS.
- A. Dakshinamoorthy and H. Garcia, Metal–organic frameworks as solid catalysts for the synthesis of nitrogen-containing heterocycles, Chem. Soc. Rev., 2014, 43, 5750–5765 RSC.
- M. Y. Wani, A. Ahmad, S. Kumar and A. J. F. N. Sobral, Flucytosine analogues obtained through Biginelli reaction as efficient combinative antifungal agents, Microb. Pathog., 2017, 105, 57–62 CrossRef CAS.
- X. Zhu, G. Zhao, X. Zhou, X. Xu, G. Xia, Z. Zheng, L. Wang, X. Yang and S. Li, 2,4-Diaryl-4,6,7,8-tetrahydroquinazolin-5(1H)-one derivatives as anti-HBV agents targeting at capsid assembly, Bioorg. Med. Chem. Lett., 2010, 20, 299–301 CrossRef CAS.
- M. Matias, G. Campos, A. O. Santos, A. Falcão, S. Silvestre and G. Alves, Potential antitumoral 3,4-dihydropyrimidin-2-(1H)-ones: synthesis, in vitro biological evaluation and QSAR studies, RSC Adv., 2016, 6, 84943–84958 RSC.
- G. Lauro, M. Strocchia, S. Terracciano, I. Bruno, K. Fischer, C. Pergola, O. Werz, R. Riccio and G. Bifulco, Exploration of the dihydropyrimidine scaffold for the development of new potential anti-inflammatory agents blocking prostaglandin E2 synthase-1 enzyme (mPGES-1), Eur. J. Med. Chem., 2014, 80, 407–415 CrossRef CAS.
- T. N. Akhaja and J. P. Raval, 1,3-dihydro-2H-indol-2-ones derivatives: design, synthesis, in vitro antibacterial, antifungal and antitubercular study, Eur. J. Med. Chem., 2011, 46, 5573–5579 CrossRef CAS.
- C. O. Kappe, A Reexamination of the Mechanism of the Biginelli Dihydropyrimidine Synthesis. Support for an N-Acyliminium Ion Intermediate, J. Org. Chem., 1997, 62, 7201–7204 CrossRef CAS.
- A. K. Gupta, D. De, K. Tomar and P. K. Bharadwaj, A Cu(II) metal–organic framework with significant H2 and CO2 storage capacity and heterogeneous catalysis for the aerobic oxidative amination of C(sp3)–H bonds and Biginelli reactions, Dalton Trans., 2018, 47, 1624–1634 RSC.
- S.-Y. Zhao, Z.-Y. Chen, N. Wei, L. Liu and Z.-B. Han, Highly Efficient Cooperative Catalysis of Single-Site Lewis Acid and Brønsted Acid in a Metal–Organic Framework for the Biginelli Reaction, Inorg. Chem., 2019, 58, 7657–7661 CrossRef.
- A. Verma, D. De, K. Tomar and P. K. Bharadwaj, An Amine Functionalized Metal–Organic Framework as an Effective Catalyst for Conversion of CO2 and Biginelli Reactions, Inorg. Chem., 2017, 56, 9765–9771 CrossRef CAS.
- S. Rostamnia and A. Morsali, Basic isoreticular nanoporous metal–organic framework for Biginelli and Hantzsch coupling: IRMOF-3 as a green and recoverable heterogeneous catalyst in solvent-free conditions, RSC Adv., 2014, 4, 10514–10518 RSC.
- J. C. M. Willig, G. Granetto, D. Reginato, F. R. Dutra, E. F. Poruczinski, I. M. de Oliveira, H. A. Stefani, S. D. de Campos, E. A. de Campos, F. Manarin and G. V. Botteselle, A comparative study between Cu(INA)2-MOF and [Cu(INA)2(H2O)4] complex for a click reaction and the Biginelli reaction under solvent-free conditions, RSC Adv., 2020, 10, 3407–3415 RSC.
Footnote |
† Electronic supplementary information (ESI) available. CCDC 2014940 and 2014941. For ESI and crystallographic data in CIF or other electronic format see DOI: 10.1039/d0qm00611d |
|
This journal is © the Partner Organisations 2021 |
Click here to see how this site uses Cookies. View our privacy policy here.