DOI:
10.1039/D0NJ05152G
(Paper)
New J. Chem., 2021,
45, 85-91
Very thermostable energetic materials based on a fused-triazole: 3,6-diamino-1H-[1,2,4]triazolo[4,3-b][1,2,4]triazole†
Received
19th October 2020
, Accepted 25th November 2020
First published on 25th November 2020
Abstract
3,6-Diamino-1H-[1,2,4]triazolo[4,3-b][1,2,4]triazole (1) and its energetic salts (2–9) were designed and synthesized based on a fused-triazole backbone with two C-amino groups as substituents. Their physicochemical and energetic properties were measured or calculated. Among them, compound 1 exhibits superior thermostability (Td
(onset): 261 °C), surpassing its analogues 3,7-diamino-7H-[1,2,4]triazolo[4,3-b][1,2,4]triazole (DATT, 219 °C) and 3,6,7-triamino-7H-[1,2,4]triazolo[4,3-b][1,2,4]triazole (TATOT, 245 °C). The differences in thermal stabilities were further investigated by determining the lowest bond dissociation energies (BDE) where a positive correlation between the stability of the molecules and the lowest BDE values is observed. The results show that 1 with the highest value for the lowest BDE has a superior thermostability in comparison to DATT and TATOT. The energetic salts (2–9) also exhibit remarkable thermal stabilities as well as low impact and friction sensitivities. The fused-triazole backbone 1H-[1,2,4]triazolo[4,3-b][1,2,4]triazole with two C-amino groups as substituents is shown to be a promising building block for construction of very thermally stable energetic materials.
Introduction
Nitrogen rich five-membered heterocycles have become an important option for designing and synthesizing energetic materials owing to their high positive heats of formation and high densities.1–4 Heterocyclic rings including furazan, pyrazole, triazole, tetrazole and their derivatives provide varied backbones for the construction of energetic molecules.5,6 Among them, 1H-1,2,4-triazole has been shown to be a promising building block in the design of high performance energetic materials. The reported energetic molecules based on 1H-1,2,4-triazole exhibit high densities and remarkable thermal stabilities.7–11 Nineteen different salts based on 3,5-dinitro-1,2,4-triazolates that show low sensitivities and high thermal stabilities were synthesized.12 3-Nitro-1,2,4-triazole-5-one (NTO) is another powerful insensitive triazole-based explosive, whose detonation performance is comparable to RDX and HMX.13–15
Relative to a single triazole ring, fused heterocyclic rings have higher nitrogen content and better thermostability. The numerous C–N bonds and N–N bonds in fused heterocycles contribute markedly to the powerful storage capacity of energetic materials.16 3,6,7-Triamino-7H-[1,2,4]triazolo[4,3-b][1,2,4]triazole (TATOT) is representative of a compound based on a fused triazole backbone.17,18 TATOT and its ionic derivatives exhibit good thermal stability and high detonation performance. In 2017, a combination of the TATOT cation with 5-dinitromethyl-3-trinitromethyl-1H-1,2,4-triazolate resulted in a high-energy salt with a density of 1.90 g cm−3 and an outstanding detonation performance.19 3,7-Diamino-7H-[1,2,4]triazolo[4,3-b][1,2,4]triazole (DATT) and its energetic salts have also been reported as fused heterocyclic compounds with high energy densities.4
The amino group plays an important role in enhancing sensitivity and improving thermal stability via formation of inter-/intra-molecular hydrogen bonds.20 Therefore, 3,6-diamino-1H-[1,2,4]triazolo[4,3-b][1,2,4]triazole (1) was designed with a fused triazole as the backbone and two C-amino groups as substituents for study in this work (Fig. 1). Compound 1 has a backbone similar to TATOT and DATT, but with a different number (TATOT) and positions (DATT) of the amino groups. It is interesting to note that the latter two characteristics make a major difference in the thermostability of energetic molecules. The energetic properties of the monocationic and dicationic salts (2–9) based on 1 have also been investigated.
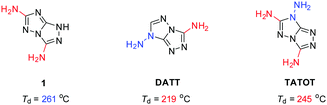 |
| Fig. 1 The structures and decomposition temperatures of energetic compounds (1, DATT and TATOT) based on [1,2,4]triazolo[4,3-b][1,2,4]triazole. | |
Results and discussion
Synthesis
The synthetic route is shown in Scheme 1. 3-Amino-5-hydrazino-1,2,4-triazole dihydrochloride was prepared by reacting 1,3-diamino-1,2,4-triazole with sodium nitrite in dilute HCl and followed by adding SnCl2 in concentrated HCl solution. Then 3-amino-5-hydrazino-1,2,4-triazole dihydrochloride was dissolved in dilute hydrochloric acid (2 M) and cyanogen bromide was added. The reaction mixture was heated to 60 °C and stirred at reflux for 2 h. The reaction mixture was concentrated with air and treated with a saturated NaHCO3 solution to give crude product 1, which was purified further by recrystallization in hot water. The monocationic salts (2–4) and dicationic salts (5–9) were synthesized by reacting 1 with various energetic acids at 60 °C for 2 h in water.
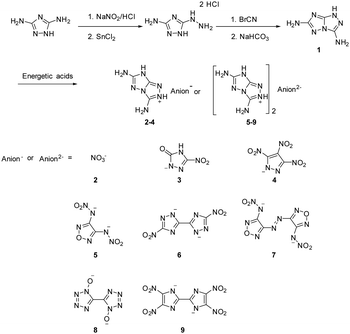 |
| Scheme 1 Synthesis of 1 and its energetic salts (2–9). | |
15N NMR spectroscopy
The 15N NMR spectra (Fig. 2) of 1, 2, and 5 were determined in d6-DMSO. In the spectrum of 1, there are seven signals, the nitrogen atom signals of the two amino groups are observed at the highest field (δ = −337.9 ppm (N6) and δ = −328.0 ppm (N7)). The fused triazole backbone has signals at δ = −211.4 (N1), δ = −170.3 (N2), δ = −180.8 (N3), δ = −156.2 (N4) and δ = −246.2 ppm (N5). In the spectra of 2 and 5, due to the interactions with different anions, the nitrogen signals of the amino groups on the cation are shifted to lower field (δ = −326.9 ppm (N6); δ = −324.0 ppm (N7)). There are only six signals for 2, the signals of N4 and N1 could not be detected due to rapid proton exchange. The chemical shift of the nitrogen atom in the nitrate ion (N8) is found at δ = −3.8 ppm. Due to the symmetric structure of nitramino-furazanate in 5, three signals were found at δ = 12.1 ppm (N8); δ = −21.4 ppm (N10); δ = −176.1 ppm (N9) for the anion. The two signals for nitrogen atoms in amino groups were observed at high field. However, only one signal was detected for the fused-triazole backbone. When two drops of D2O were added (bottom of Fig. 2), one more signal (δ = −189.6 ppm (N3)) was observed although the others in the fused-triazole backbone are still absent.
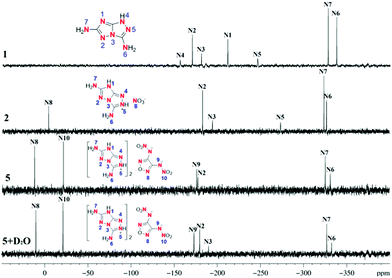 |
| Fig. 2
15N NMR spectra of 1, 2, 5 and 5 + D2O. | |
Crystal structures
Compound 1 crystallizes in the monoclinic space group P21/n with the density of 1.766 g cm−1 at 99.9 K. The crystal structure of 1 is shown in Fig. 3a. All the atoms are essentially in the same plane. The torsion angles are N6–C2–N2–N1 = 176.55°; N2–N1–C1–N5 = −178.44°; N1–C1–N5–C3 = 179.87°; C3–N4–N3–C2 = −177.63°; N1–C1–N3–N4 = −179.62°. The C–N bond lengths of two C-amino groups are 1.348 Å (C2–N6) and 1.373 Å (C3–N7), which are shorter than that (C3–N5 = 1.377 Å) in the fused triazole backbone. Additionally, there are several hydrogen bonds between the molecules in the crystal structure (Fig. 3b). Both C-amino groups participate in hydrogen bonding (N6–H6A⋯N2 2.198 Å, N6–H6B⋯N4 2.040 Å).
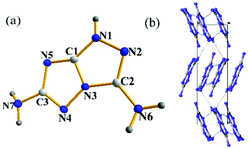 |
| Fig. 3 (a) Molecular structure of 1. (b) Packing diagram of 1 along the a axis. | |
Compound 4·H2O (Fig. 4a) crystallizes as a monoclinic in the monoclinic space group P21/n at 100 K, with a calculated density of 1.794 g cm−1. The bond lengths of the two C-amino groups are N6–C1 = 1.328 Å and C2–N7 = 1.338 Å, respectively. They are shorter than those in 1 owing to the introduction of an anion. The packing diagram of 4·H2O is shown in Fig. 4b viewed along the a axis, which is strongly influenced by strong hydrogen bonds involving intermolecular and intramolecular interactions (N6–H6B⋯O6, N4–H4⋯N8 and O1W–H1WB⋯N2). The others are given in the ESI.†
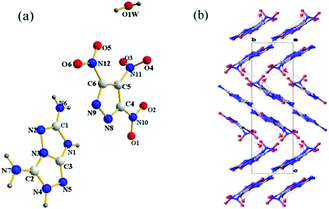 |
| Fig. 4 (a) Molecular structure of 4·H2O. (b) Packing diagram of 4·H2O along the a axis. | |
Physicochemical and energetic properties
The physiochemical properties of 1–9 are summarized in Table 1. All the compounds show good densities between 1.68 to 1.78 g cm−3; among them, 2 and 9 have a density of 1.78 g cm−3, which is comparable to RDX (1.80 g cm−3). All compounds have densities greater than DATT (1.71 g cm−3), except 7. The values of the heats of formation were calculated by using the Gaussian 09 suite of programs.21 Compounds 5–8 have high positive heats of formation. Especially for 8, it has the highest positive heat of formation at 1246.6 kJ mol−1. The heats of formation of all the new molecules exceed DATT (480.3 kJ mol−1) and RDX (70.3 kJ mol−1).
Table 1 Physicochemical and energetic properties of 1–9 compared with RDX, TATOT and DATT
Comp. |
ρ
(g cm−3) |
D
v
(m s−1) |
P
(GPa) |
ΔHfd (kJ mol−1/kJ g−1) |
T
dec
(°C) |
ISf (J) |
FSg (N) |
Density measured by a gas pycnometer at 25 °C.
Calculated detonation velocity.
Calculated detonation pressure.
Calculated molar enthalpy of formation in solid state.
Temperature of decomposition (onset).
Impact sensitivity.
Friction sensitivity.
Ref. 5.
Ref. 16.
|
1
|
1.71 |
7891 |
21.0 |
302.6/2.18 |
261 |
>40 |
>360 |
2
|
1.78 |
8531 |
28.1 |
167.3/0.83 |
219 |
15 |
240 |
3
|
1.76 |
8101 |
24.5 |
291.0/1.08 |
232 |
>40 |
>360 |
4
|
1.74 |
8695 |
32.1 |
810.4/2.25 |
202 |
>40 |
>360 |
5
|
1.72 |
8421 |
27.1 |
1128.7/2.41 |
238 |
20 |
>360 |
6
|
1.72 |
8050 |
23.9 |
1088.9/2.16 |
277 |
36 |
>360 |
7
|
1.68 |
8215 |
25.6 |
1124.8/1.87 |
163 |
40 |
>360 |
8
|
1.75 |
8591 |
27.7 |
1426.6/3.18 |
293 |
20 |
>360 |
9
|
1.78 |
8237 |
26.6 |
915.1/1.54 |
272 |
>40 |
>360 |
RDX |
1.80 |
8795 |
34.9 |
70.3/0.32 |
204 |
7.5 |
120 |
DATTh |
1.71 |
8461 |
25.4 |
480.3/3.45 |
219 |
40 |
360 |
TATOTi |
1.72 |
9385 |
29.7 |
446.7/2.90 |
245 |
40 |
360 |
Thermal stability is one of the key properties for energetic materials, considering their practical applications.22,23 The thermal behavior of these compounds was determined by differential scanning calorimetry (DSC) at a heating rate of 5 °C min−1. Most of the compounds have very high thermal decomposition temperatures (onset), ranging from 202 (4) to 293 °C (8). Generally, the decomposition temperatures of these salts are higher than those of RDX (204 °C) and DATT (219 °C). The combination of 3,6-diamino-fused triazole cations and anions increases a large number of intermolecular and intramolecular interactions improving the thermal stabilities of these energetic salts.
Based on the similar fused triazole as the skeleton, 1, DATT and TATOT have different decomposition temperatures. Compound 1 begins to decompose at 261 °C, which is higher than DATT (219 °C) and TATOT (245 °C). These differences encouraged us to explore the structure further. The thermal stabilities of the energetic materials are closely related to the initial stages of the first decomposition, which can be deduced on the basis of bond dissociation energies (BDE).24 The BDE levels were obtained by using the Gaussian 09 program. Possible initial pyrolysis initiation bonds were considered by breaking the following bonds: two C-amino groups in 1, C-amino and N-amino groups in DATT and two C-amino and an N-amino groups in TATOT. The dissociation energies of the corresponding bonds are shown in Fig. 5. The lowest BDE of 1 (Fig. 5a) is 116.5 kJ mol−1 (C-NH2), while for DATT (Fig. 5b) and TATOT (Fig. 5c), the values are 23.5 kJ mol−1 (N-NH2) and 70.8 kJ mol−1 (N-NH2), respectively. The BDE values of N-amino groups are lower than C-amino groups in this system. Considering their structural similarities, the differences in the thermal stabilities should originate from the presence of N-amino groups on DATT and TATOT. The molecule having the higher value of the lowest BDE, tends to be more stable thermally. Compound 1 with the largest value of the lowest BDE (116.5 kJ mol−1) has the best thermal stability (Td: 261 °C), and DATT, with the smallest value of lowest BDE (23.5 kJ mol−1) exhibits the worst thermal stability (Td: 219 °C)
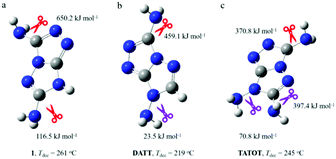 |
| Fig. 5 The bond dissociation energies (BDE) of (a) 1, (b) DATT5 and (c) TATOT.18 | |
The detonation pressures (P), and velocities (Dv) were calculated by using the EXPLO5 (v6.01) program, based on the calculated heats of formation and measured densities.25 The detonation performances are shown in Table 1. The calculated detonation velocities range from 7891 m s−1 to 8695 m s−1 which are superior to TNT (6881 m s−1) and are comparable to RDX (8795 m s−1). The calculated detonation pressures lie in the range from 21.0 GPa to 32.1 GPa, which are higher than TNT (19.5 GPa). Among these molecules, 4 demonstrates outstanding detonation velocity (8695 m s−1) and superior detonation pressure (32.1 Pa).
A fine balance between low sensitivity and high detonation performance is highly desirable for energetic materials.26 The mechanical stimuli were measured by using friction tester and standard BAM drop hammer techniques.27 The impact sensitivities (IS) and friction sensitivities (FS) of all compounds are listed in Table 1. All of the energetic salts are insensitive to both impact and friction stimuli, with impact sensitivity over 15 J and friction sensitivity up to 360 N (except 2). The insensitive properties of 1–9, together with their good thermal stabilities and comparable detonation properties, highlight their application potential as energetic materials.
Conclusions
A series of energetic molecules were designed and synthesized based on heterocyclic triazole-fused skeletons. The physical and chemical properties, including density, decomposition temperature, friction sensitivity, impact sensitivity as well as detonation performance were investigated. Except for 7, the densities of compounds 1–9 are above 1.7 g cm−3; the densities of 2 and 9 are comparable to that of RDX. Compound 4 has the best detonation performance with a detonation velocity of 8695 m s−1 and a detonation pressure of 32.1 GPa. All compounds are highly insensitive to both friction and impact stimuli. The series of compounds have relatively high thermal stabilities with the highest decomposition temperature at 293 °C (8). BDE investigation shows that 1 has the higher value of the lowest BDE value and has a better thermal stability than its analogues DATT and TATOT. These molecules exhibit promising properties and may find applications as energetic materials especially as heat-resistant explosives due to their superior thermal stabilities.
Experimental section
Caution! The compounds in this work are potentially energetic materials that could explode under certain conditions (e.g., impact, friction, or electric discharge). Appropriate safety precautions, such as the use of safety shields in a fume hood and personal protection equipment (safety glasses, face shields, earplugs, as well as gloves) should always be observed when handling these materials.
General methods
All reagents were purchased from AKSci or Alfa Aesar in analytical grade and were used as supplied. The 1H and 13C spectra were recorded on a 300 MHz (Bruker AVANCE 300) nuclear magnetic resonance spectrometer. Chemical shifts for 1H and 13C NMR spectra are given with respect to external (CH3)4Si. [D6] DMSO was used as a locking solvent unless otherwise stated. The 15N spectra were recorded on a 500 MHz (Bruker AVANCE 500) nuclear magnetic resonance spectrometer. IR spectra were recorded using KBr pellets with a FT-IR spectrometer (Thermo Nicolet AVATAR 370). Density was determined at room temperature by employing a Micromeritics AccuPyc II 1340 gas pycnometer. Thermal decomposition (onset) points were recorded using a dry nitrogen gas purge and a heating rate of 5 °C min−1 on a differential scanning calorimeter (DSC, TA Instruments Q2000). Elemental analyses (C, H, N) were performed with a Vario Micro cube Elementar Analyser. Impact and friction sensitivity measurements were made using a standard BAM Fallhammer and a BAM friction tester.
Computational methods
The gas phase enthalpies of formation for 1 was calculated based on G2 method. The solid-state heat of formation was calculated with Trouton's rule according to eqn (1) (T represents either the melting point or the decomposition temperature when no melting occurs prior to decomposition).28 | ΔHsub = 188/J mol−1 K−1 × T | (1) |
For energetic salts, the solid phase enthalpy of formation is obtained using a Born–Haber energy cycle.29 For compounds which are hydrates (4·H2O and 7·2H2O), the solid-phase enthalpy of formation is obtained by adding the gas phase heat of formation of the anhydrous compound to that of water (−241.8 kJ mol−1).30
Crystal structure analysis
Single colorless needle crystals (1) of dimensions 0.13 × 0.13 × 0.10 mm3, and single yellow needle crystals (4·H2O) of dimensions 0.33 × 0.05 × 0.02 mm3 were selected and mounted on a nylon loop with paratone oil on a XtaLAB Synergy, Dualflex, HyPix diffractometer. The crystalS were kept at a steady T = 99.9(3) K and T = 100.00(10) K during data collection, respectively. The structures were solved with the ShelXT31 solution program using dual methods and by using Olex232 as the graphical interface. The model were refined with ShelXL 2018/333 using full matrix least squares minimisation on F2.
Synthesis
Synthesis of 1.
3-Hydrazinium-5-amino-1H-1,2,4-triazolium dichloride34–36 (1.87 g, 10.0 mmol) was dissolved in dilute hydrochloric acid (25 mL, 2 M), cyanogen bromide (1.50 g, 14.0 mmol) was added, and the solution was heated with stirring at 60 °C for 2 h. The solution was held at reflux for an additional 2 h. After cooling to 25 °C, the solution was concentrated to give a white precipitate which was collected by filtration. The white solid was dissolved in distilled water (15 mL). Sodium bicarbonate (0.84 g, 10.0 mmol) was added, and after stirring 15 min at 25 °C, the white solid (1.08 g, 78% yield) was filtered, washed with water (15 mL × 2) and dried in air.
1
.
White solid. Td
(onset): 247 °C. 1H NMR (d6-DMSO): δ 11.50 (br), 6.02 (s, 2H), 5.45 (s, 2H) ppm. 13C NMR (d6-DMSO): δ 169.7, 155.3, 141.3 ppm. 15N NMR (d6-DMSO): δ −156.2, −170.3, −180.8, −211.4, −246.2, −328.0, −337.9 ppm. IR (KBr):
= 3414, 3333, 3150, 1685, 1647, 1617, 1587, 1540, 1491, 1437, 1357, 1255, 1184, 1133, 1088, 1012, 847, 751, 678, 626 cm−1. C3H5N7 (139.12): calcd: C 25.90, H 3.62, N 70.48%. Found: C 25.57, H 3.58, N 69.28%.
General procedure for synthesis of 2–4.
The energetic acid (2.0 mmol) was dissolved in water (10 mL), then 1 (0.28 g, 2.0 mmol) was added while stirring. The reaction mixture was heated to 60 °C and stirred for 2 h. The precipitate formed, was collected by filtration, and washed with water (5 mL × 2), and ethanol (5 mL × 2) and dried in vacuum to give the products 2–4.
2
.
Yellow solid. Td
(onset): 219 °C. 1H NMR (d6-DMSO): δ 12.40 (br), 8.02 (br, 2H), 7.05 (br, 2H) ppm. 13C NMR (d6-DMSO): δ 159.7, 148.2, 140.6 ppm. IR (KBr):
= 3238, 3157, 1690, 1637, 1569, 1527, 1460, 1360, 1177, 1047, 988, 841, 818, 680 cm−1. C3H6N8O3 (202.13): calcd: C 17.83, H 2.99, N 55.44%. Found: C 17.86, H 3.13, N 55.52%.
3
.
White solid. Tm: 185 °C. Td
(onset): 232 °C. 1H NMR (d6-DMSO): δ 6.22 (s, 2H), 5.92 (br, 2H) ppm. 13C NMR (d6-DMSO): δ 168.9, 155.2, 154.7, 148.9, 141.2 ppm. IR (KBr):
= 3563, 3477, 1674, 1507, 1391, 1311, 1175, 1120, 1055, 1022, 976, 839, 782, 711, 701, 682, 632, 606 cm−1. C5H7N11O3 (269.19): calcd: C 22.51, H 2.62, N 57.24%. Found: C 22.41, H 2.71, N 57.51%.
4·H2O
.
White solid. Td
(onset): 222 °C. δ1H NMR (d6-DMSO): δ 13.10 (br), 8.03 (br, 2H), 7.05 (br, 2H) ppm. 13C NMR (d6-DMSO): δ 159.5, 148.2, 146.9, 140.5, 122.0 ppm. IR (KBr):
= 3577, 3443, 3331, 1676, 1641, 1577, 1528, 1460, 1356, 1318, 1299, 1158, 1085, 847, 807, 767, 633 cm−1. C6H8N12O7 (360.20): calcd: C 20.01, H 2.24, N 46.66%. Found: C 20.02, H 2.26, N 46.92%.
General procedure for synthesis of 5–9.
The energetic acid (1.0 mmol) was dissolved in water (10 mL), then 1 (0.28 g, 2.0 mmol) was added with stirring. The reaction mixture was heated at 60 °C and stirred for 2 h. The precipitate formed, was collected by filtration, and washed with water (5 mL × 2), and ethanol (5 mL × 2) and dried in vacuum to give the products 5–9.
5
.
White solid. Td
(onset): 238 °C. 1H NMR (d6-DMSO): δ 8.92 (br, 2H), 7.18 (br, 2H) ppm. 13C NMR (d6-DMSO): δ 163.7, 151.1, 150.5, 140.9 ppm. IR (KBr):
= 3433, 3367, 1711, 1690, 1649, 1517, 1487, 1452, 1413, 1358, 1022, 991, 889, 864, 842, 814, 662 cm−1. C8H12N20O5 (468.31): calcd: C 20.62, H 2.63, N 59.82%. Found: C 20.51, H 2.63, N 59.91%.
6
.
White solid. Td
(onset): 277 °C. 1H NMR (d6-DMSO): δ 11.80 (br), 6.18 (s, 2H), 5.62 (br, 1H) ppm. 13C NMR (d6-DMSO): δ 169.2, 164.6, 154.9, 151.2, 141.2 ppm. IR (KBr):
= 3414, 3331, 3154, 1688, 1617, 1542, 1490, 1441, 1397, 1358, 1256, 1185, 1135, 1088, 1011, 848, 750, 679, 626 cm−1. C10H12N22O4 (504.36): calcd: C 23.81, H 2.42, N 61.10%. Found: C 23.84, H 2.43, N 61.70%.
7·2H2O
.
Orange solid. Td
(onset): 163 °C. 1H NMR (d6-DMSO): δ 13.00 (br), 7.98 (s, 2H), 6.96 (br, 1H) ppm. 13C NMR (d6-DMSO): δ 160.0, 159.2, 152.2, 148.4, 140.6 ppm. IR (KBr):
= 3428, 3365, 3184, 1680, 1606, 1588, 1522, 1457, 1388, 1335, 1272, 1173, 1079, 1050, 1018, 991, 946, 882, 838, 820, 771, 750, 689, 654, 604 cm−1. C10H16N24O8 (600.39): calcd: C 20.00, H 2.69, N 55.99%. Found: C 20.28, H 2.65, N 56.61%.
8
.
White solid. Td
(onset): 292 °C. 1H NMR (d6-DMSO): δ 7.07 (br) ppm. 13C NMR (d6-DMSO): δ 164.8, 151.6, 140.8, 135.1 ppm. IR (KBr):
= 3368, 3194, 1700, 1669, 1517, 1421, 1384, 1362, 1343, 1235, 1168, 1114, 1061, 996, 869, 822, 783, 743, 701, 679 cm−1. C8H12N22O2 (448.33): calcd: C 21.43, H 2.70, N 68.73%. Found: C 21.43, H 2.86, N 67.51%.
9
.
Yellow solid. Td
(onset): 272 °C. 1H NMR (d6-DMSO): δ 7.74 (br) ppm. 13C NMR (d6-DMSO): δ 161.2, 149.2, 142.3, 140.7, 139.7 ppm. IR (KBr):
= 3427, 3340, 3151, 1697, 1678, 1651, 1626, 1543, 1502, 1482, 137, 1302, 1253, 1207, 1115, 1024, 949, 856, 835, 813, 752 cm−1. C12H12N22O8 (592.37): calcd: C 24.33, H 2.04, N 52.02%. Found: C 24.23, H 2.10, N 51.87%.
Conflicts of interest
The authors declare no conflict of interest.
Acknowledgements
Financial support of the Office of Naval Research (N00014-16-1-2089), and the Defense Threat Reduction Agency (HDTRA 1-15-1-0028) is gratefully acknowledged. The Rigaku Synergy S Diffractometer was purchased with support from the National Science Foundation MRI program (1919565). This work was supported by the National Natural Science Foundation of China (No. 21905135), Province the Natural Science Foundation of Jiangsu Province (BK20190458), the Fundamental Research Funds for the Central Universities (No. 30919011270), and the Large Equipment Open Funding of Nanjing University of Science and Technology.
Notes and references
- C. Qi, S. Li, Y. Li, Y. Wang, X. Chen and S. Pang, A novel stable high-nitrogen energetic material: 4,4′-azobis(1,2,4-triazole), J. Mater. Chem., 2011, 21(9), 3221–3225 RSC.
- Q. Lin, Y. Li, C. Qi, W. Liu, Y. Wang and S. Pang, Nitrogen-rich salts based on 5-hydrazino-1H-tetrazole: a new family of high-density energetic materials, J. Mater. Chem. A, 2013, 1(23), 6776–6785 RSC.
-
T. M. Klapötke, Preface to the first English edition: Chemistry of High-Energy Materials, Chemistry of High-Energy Materials, Walter de Gruyter, Berlin, 2nd edn, 2012 Search PubMed.
- J. Chen, J. Tang, H. Xiong, H. Yang and G. Cheng, Combining triazole and furazan frameworks via methylene bridges for new insensitive energetic materials., Energy Mater. Front., 2020, 1(1), 34–39 CrossRef.
- Y. Tang, C. He, H. G. Imler, D. A. Parrish and J. M. Shreeve, High-performing and thermally stable energetic 3,7-diamino-7H-[1,2,4]triazolo[4,3-b][1,2,4] triazole derivatives, J. Mater. Chem. A, 2017, 5(13), 6100–6105 RSC.
- H. Gao, Y. Zhou and J. M. Shreeve, Dinitromethyl groups enliven energetic salts, Energy Mater. Front., 2020, 1(1), 2–15 CrossRef.
- T. Fei, Y. Du and S. Pang, Theoretical design and prediction of properties for dinitromethyl, fluorodinitromethyl, and (difluoroamino)dinitromethyl derivatives of triazole and tetrazole, RSC Adv., 2018, 8(19), 10215–10227 RSC.
- J. C. Oxley, J. L. Smith, E. Rogers and X. Dong, NTO decomposition products tracked with 15N labels, J. Mater. Chem. A, 1997, 101(19), 3531–3536 CAS.
- G. Singh, I. P. S. Kapoor, S. M. Mannan and S. K. Tiwari, Studies on energetic compounds: Part 6 synthesis of ring-substituted arylammonium salts of 3-nitro-1,2,4-triazole-5-one(NTO), J. Energ. Mater., 1998, 16(1), 31–43 CrossRef CAS.
- T. B. Brill, P. E. Gongwer and G. K. Williams, Thermal decomposition of energetic materials. 66. Kinetic compensation effects in HMX, RDX, and NTO, J. Phys. Chem., 1994, 98(47), 12242–12247 CrossRef CAS.
- M. Zhang, C. Li, H. Gao, W. Fu, Y. Li, L. Tang and Z. Zhou, Promising hydrazinium 3-nitro-1,2,4-triazol-5-one and its analogs, J. Mater. Sci., 2016, 51(24), 10849–10862 CrossRef CAS.
- R. Haiges, G. Belanger-Chabot, S. M. Kaplan and K. O. Christe, Synthesis and structural characterization of 3,5-dinitro-1,2,4-triazolates, Dalton Trans., 2015, 44(7), 2978–2988 RSC.
- H. Xiao, X. Ju, L. Xu and G. Fang, A density-functional theory investigation of 3-nitro-1,2,4-triazole-5-one dimers and crystal, J. Chem. Phys., 2004, 121(24), 12523–12531 CrossRef CAS.
- J. Li, S. Jin, G. Lan, X. Ma, J. Ruan, B. Zhang, S. Chen and L. Li, Morphology control of 3-nitro-1,2,4-triazole-5-one (NTO) by molecular dynamics simulation, CrystEngComm, 2018, 20(40), 6252–6260 RSC.
- G. Singh, I. P. S. Kapoor, S. M. Mannan and S. K. Tiwari, Studies on energetic compounds part 7 thermolysis of ring substituted arylammonium salts of 3-nitro-1,2,4-triazole-5-one (NTO), J. Energ. Mater., 1998, 16(2–3), 101–118 CrossRef CAS.
- P. Yin, J. Zhang, D. A. Parrish and J. M. Shreeve, Energetic fused triazoles – a promising C–N fused heterocyclic cation, J. Mater. Chem. A, 2015, 3(16), 8606–8612 RSC.
- K. T. Potts and C. A. Hirsch, 1,2,4-Triazoles. XVIII. synthesis of 5H-s-triazolo[5,1-c]-s-triazole and its derivatives, J. Org. Chem., 1968, 33(1), 143–150 CrossRef CAS.
- T. M. Klapötke, P. C. Schmid, S. Schnell and J. Stierstorfer, 3,6,7-Triamino-[1,2,4]triazolo[4,3-b][1,2,4]triazole: a non-toxic, high-performance energetic building block with excellent stabilit, Chem. – Eur. J., 2015, 21(25), 9219–9228 CrossRef.
- S. Dharavath, J. Zhang, G. H. Imler, D. A. Parrish and J. M. Shreeve, 5-(Dinitromethyl)-3-(trinitromethyl)-1,2,4-triazole and its derivatives: a new application of oxidative nitration towards gem-trinitro-based energetic materials, J. Mater. Chem. A, 2017, 5(10), 4785–4790 RSC.
- A. B. Sheremetev, V. L. Korolev, A. A. Potemkin, N. S. Aleksandrova, N. V. Palysaeva, T. H. Hoang, V. P. Sinditskii and K. Y. Suponitsky, Oxygen-rich 1,2,4-triazolo[3,4-d]-1,2,4-triazolo[3,4-f]furazano[3,4-b]pyrazines as energetic materials, Asian J. Org. Chem., 2016, 5(11), 1388–1397 CrossRef CAS.
-
M. J. Frisch, G. W. Trucks, H. B. Schlegel, G. E. Scuseria, M. A. Robb, J. R. Cheeseman, J. A. Montgomery Jr, T. Vreven, K. N. Kudin, J. C. Burant, J. M. Millam, S. S. Iyengar, J. Tomasi, V. Barone, B. Mennucci, M. Cossi,G. Scalmani, N. Rega, G. A. Petersson, H. Nakatsuji, M. Hada, M. Ehara, K. Toyota, R. Fukuda, J. Hasegawa, M. Ishida, T. Nakajima, Y. Honda, O. Kitao, H. Nakai,M. Klene, X. Li, J. E. Knox, H. P. Hratchian, J. B. Cross, V. Bakken, C. Adamo, J. Jaramillo, R. Gomperts, R. E. Stratmann, O. Yazyev, A. J. Austin, R. Cammi, C. Pomelli, J. W. Ochterski, P. Y. Ayala, K. Morokuma, G. A. Voth, P. Salvador, J. J. Dannenberg, V. G. Zakrzewski, S. Dapprich, A. D. Daniels, M. C. Strain, O. Farkas, D. K. Malick, A. D. Rabuck, K. Raghavachari, J. B. Foresman, J. V. Ortiz, Q. Cui, A. G. Baboul, S. Clifford, J. Cioslowski, B. B. Stefanov, G. Liu, A. Liashenko, P. Piskorz, I. Komaromi, R. L. Martin, D. J. Fox, T. Keith, M. A. Al-Laham, C. Y. Peng, A. Nanayakkara, M. Challacombe, P. M. W. Gill, B. Johnson, W. Chen, M. W. Wong, C. Gonzalez and J. A. Pople, Gaussian 09 (Revision E.01), Gaussian, Inc., Wallingford CT, 2009 Search PubMed.
- Y. Oyumi and T. B. Brill, Thermal decomposition of energetic materials 3. a high-rate, in situ, FTIR study of the thermolysis of RDX and HMX with pressure and heating rate as variables, Combust. Flame, 1985, 62(3), 213–224 CrossRef CAS.
- A. A. Dippold and T. M. Klapötke, Nitrogen-rich bis-1,2,4-triazoles—a comparative study of structural and energetic properties, Chem. – Eur. J., 2012, 18(52), 16742–16753 CrossRef CAS.
- Z. Yu and E. R. Bernstein, Sensitivity and performance of azole-based energetic materials, J. Phys. Chem. A, 2013, 117(42), 10889–10902 CrossRef CAS.
-
M. Sućeska, EXPLO5 v6.01, Brodarski Institute, Zagreb, Croatia, 2013.
- C. Snyder, L. Wells, D. Chavez, G. H. Imler and D. A. Parrish, Polycyclic N-oxides: high performing, low sensitivity energetic materials, Chem. Commun., 2019, 55(17), 2461–2464 RSC.
-
(a)
Recommendations on the Transport of Dangerous Goods, Manual of Tests and Criteria, United Nations, New York, 4th rev. edn, 2003 Search PubMed;
(b) 13.4.2 Test 3(a) (ii) BAM Fallhammer, p. 75;
(c) 13.5.1 Test 3(b) (i): BAM friction apparatus, p. 104.
- M. S. Westwell, M. S. Searle, D. J. Wales and D. H. Williams, Empirical correlations between thermodynamic properties and intermolecular forces, J. Am. Chem. Soc., 1995, 117(18), 5013–5015 CrossRef CAS.
- H. Gao, C. Ye, C. M. Piekarski and J. M. Shreeve, Computational characterization of energetic salts, J. Phys. Chem. C, 2007, 111(28), 10718–10731 CrossRef CAS.
- H. D. B. Jenkins, D. Tudela and L. Glasser, Lattice potential energy estimation for complex ionic salts from density measurements, Inorg. Chem., 2002, 41(9), 2364–2367 CrossRef CAS.
- G. M. Sheldrick, Acta Crystallogr., 2015, A71, 3 CrossRef.
- O. V. Dolomanov, L. J. Bourhis, R. J. Gildea, J. A. K. Howard and H. Puschmann, J. Appl. Crystallogr., 2009, 42, 339 CrossRef CAS.
- G. M. Sheldrick, Acta Crystallogr., 2015, C71, 3 CrossRef.
- K. E. Kleinpeter, Ab initio calculation of the anisotropy effect of multiple bonds and the ring current effect of arenes-application in conformational and configurational analysis, J. Chem. Soc., Perkin Trans. 2, 2001, 1893–1898 Search PubMed.
- P. Yin, J. Zhang, G. H. Imler, D. A. Parrish and J. M. Shreeve, Polynitro-functionalized dipyrazolo-1,3,5-triazinanes energetic polycyclization toward high density and excellent molecular stability, Angew. Chem., Int. Ed., 2017, 56(30), 8960–8964 CrossRef.
- H. Emilsson, A. Lweisson and H. Selanader, Synthesis and antihypertensive activity of some derivatives of diaminoguanidine and 3-hydrazino-4H-1,2,4-triazoles, Acta Pharm. Suec., 1983, 20(3), 161–180 CAS.
Footnotes |
† Electronic supplementary information (ESI) available. CCDC 2036394 and 2036395. For ESI and crystallographic data in CIF or other electronic format see DOI: 10.1039/d0nj05152g |
‡ Y. Tang and Z. An contributed equally to this work. |
|
This journal is © The Royal Society of Chemistry and the Centre National de la Recherche Scientifique 2021 |