DOI:
10.1039/D0NJ05066K
(Paper)
New J. Chem., 2021,
45, 92-97
A novel bromelain-MnO2 biosensor for colorimetric determination of dopamine†
Received
15th October 2020
, Accepted 19th November 2020
First published on 21st November 2020
Abstract
We report a method for determining dopamine in serum via colorimetric sensing. This new method adopts bromelain-templated MnO2 nanosheets that possess high oxidase activity. 3,3′,5,5′-Tetramethylbenzidine (TMB) can be directly oxidized by bromelain-MnO2 nanosheets to oxTMB products. Without dopamine, the absorbance at 652 nm (A652) of oxTMB products is significantly enhanced. Upon the addition of dopamine, oxidase activity is markedly inhibited because the lamellar structure decomposes to generate Mn2+ ions, and the A652 value gradually decreases. Moreover, the oxidation process of dopamine can be accelerated by Mn2+ ions, and the two hydroxyl groups on the annulated benzene of dopamine can be oxidized to form a light pink benzoquinone compound. Therefore, an economical, visual colorimetric sensing method was developed for the determination of dopamine. When the concentration of dopamine is between 100 nM and 10.0 μM, there is a linear relationship between the A652 value and the dopamine concentration. The limit of detection (LOD) was 39.8 nM. The colorimetric sensing was successfully utilized in blank spike experiments with human serum samples and fetal bovine serum samples, and the dopamine content in human serum samples could be quantitatively and accurately determined.
1. Introduction
Nanozymes have become an ideal substitute for natural enzymes due to their facile synthesis and modification, economic benefits, excellent environmental stability, and readily controllable enzymatic activity.1,2 As effective mimics of nanozymes, many nanocomposites, including carbon nanomaterials3 (carbon dots, graphene, and carbon nanotubes), metal nanomaterials4 (Au, Pt, and Ag), metal organic frameworks (MOFs), metallic oxide nanomaterials5 (Fe3O4 and TiO2), metallic sulfide nanomaterials, and halide nanomaterials, have been widely applied in biosensing.6 However, in current studies using peroxidase to mimic nanozymes, H2O2 is typically required in the reaction.7 H2O2 is a strong oxidant with high corrosivity, biotoxicity, and low stability, limiting its applications. Therefore, the development of nanomaterial oxidase mimics that require no H2O2 is becoming a new research hotspot.
Manganese dioxide (MnO2), which is widely present in natural minerals, has drawn great attention due to its wide availability, low cost, excellent properties and good biocompatibility.8,9 MnO2 has been used in various areas, including bioimaging, cancer therapy, biosensing, zeugmatography, and drug delivery.10,11 In addition, the application as an oxidase mimic has also attracted much attention in recent years.12,13 Due to its laminated structure, which is more stable than that of natural enzymes, MnO2 shows nanozyme activity similar to that of oxidase.14 However, its application is limited because the 2D MnO2 nanosheets have low catalytic activities compared with those of natural enzymes.
To overcome this limitation, Chi et al.15 reported conductive polymer nanocomposites of PANi–MnO2 hybrid nanowires. These nanocomposites have enhanced catalytic activities compared with those of PANi nanowires alone. The authors also established a convenient sensitive colorimetric sensing method for sulfite and ascorbic acid. Additionally, Liu et al.16 designed a 3D support structure by combining MnO2 and platinum nanoparticles (PtNPs) to avoid the aggregation of the PtNPs. As a colorimetric sensor, PtNPs@MnO2 can be used to detect glutathione and dopamine. However, the conventional bottom-up synthesis and the aforementioned composite assembly synthesis of MnO2 are time-consuming processes that require high costs, complex purification and additional separation steps. Furthermore, PtNPs readily aggregate, resulting in low stability. A surface coating may block the active sites of the nanozyme and prevent contact with the substrate.
Biomass materials are widely used as templates for nanomaterial synthesis, primarily for the development of metal nanoclusters or metal nanoparticles rather than two-dimensional nanomaterials. Ge et al.17,18 established a biocompatible method to synthesize MnO2 by adopting human serum albumin (HSA)-templated MnO2 nanosheets. HSA-MnO2 is a new nanozyme with high oxidase activity, high stability, appropriate size and ideal morphology. Additionally, the authors demonstrated the biocompatibility of HSA-MnO2 nanosheets as oxidases to detect glucose and glutathione in biological samples.
Our group designed an innovative fluorescence sensing platform by using nanocomposites composed of bromelain-templated gold nanoclusters (AuNCs) and MnO2 nanosheets. This platform could be used to sensitively detect glucose in human serum, as reported previously.19 Accordingly, we developed a novel colorimetric biosensor platform by synthesizing bromelain-templated MnO2 nanosheets, which had great dispersity and stability. As an oxidase mimic, bromelain-MnO2 (Bro-MnO2) could be used as a catalyst to oxidize 3,3′,5,5′-tetramethyl benzidine to blue oxTMB products with a maximum absorption at 652 nm (A652). As shown in Scheme 1, we developed a novel biosensing platform to detect the concentration of dopamine (DA) depending on the oxidase activity of Bro-MnO2. Upon the addition of dopamine, the redox reaction between the dopamine and MnO2 nanosheets contributed to the degradation of the MnO2 nanosheets, resulting in significant inhibition of their oxidase activity and a marked decrease in A652, with a gradual weakening of the blue color. In this platform, Mn2+ ions catalyze the oxidation of dopamine to a light pink dopamine-o-benzoquinone compound. Therefore, we designed a novel dopamine biosensor for quantitative analysis in serum samples by measuring the absorbance of the system.
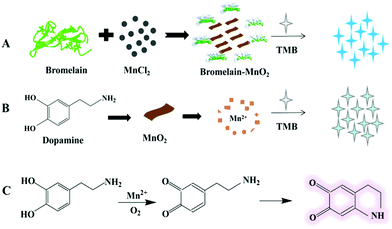 |
| Scheme 1 Principle of the colorimetric method to detect dopamine used the bromelain-MnO2 nanosheets biosensor. | |
2. Experimental
2.1 Materials and instruments
Bromelain, N-ethylmaleimide (NEM), L-adrenaline, norepinephrine(NE) and isoprenaline hydrochloride (IH) were purchased from Shanghai Sangon Biotech; Bovine serum was purchased from Zhejiang Tianhang Biotech; sodium hydroxide, anhydrous sodium acetate, and glacial acetic acid were purchased from Guangdong Guanghua Sci-tech Co. Hydrochloric acid, dopamine, anhydrous manganese chloride (MnCl2), 3,3′,5,5′-tetramethyl benzidine (TMB), ascorbic acid quencher-2 (AAQ-2), fructose, saccharose, lactose, maltose, glucose, urea, cysteine, proline, lysine, folic acid, tryptamine, tryptamine, β-phenethylamine (PEA), histamine, and glycine were purchased from Shanghai Macklin Tech; an enzyme-linked immunosorbent assay (ELISA) quantitation kit was purchased from Shanghai Lianmei Biotech. All chemicals were analytically pure and purity >99.0%. All experimental water was ultra-pure water.
A Titan G2 60-300 transmission electron microscope (FEI, US) and a K-Alpha 1063 X-ray photoelectron spectrometer (Thermo Fisher, US) were used to investigate the nanomaterials. A Shimadzu UV-2550 spectrophotometer (Kyoto, Japan) and a Thermo-Shaker thermostatic incubator (Hangzhou, China) were used to analyze the experimental system.
2.2 Synthesis of Bro-MnO2 nanosheets
Bromelain-templated MnO2 nanosheets were prepared by biotemplate synthesis. A 600 μL volume of 2 mg mL−1 bromelain solution was added to 320 μL of 5 mM MnCl2 under vigorous agitation. Next, 140 μL of 0.1 mM NaOH solution was added to adjust the pH to 9.0, and the solution gradually became light brown. Ultrapure water was used to dilute the volume to 1.5 mL. After 50 min of vigorous agitation at room temperature, Bro-MnO2 nanosheets were produced. Finally, well-dispersed MnO2 nanosheets were obtained by washing and centrifugation 3 times (4000 rpm for 10 min) to eliminate residues. The A385 value was obtained by measuring the ultraviolet spectrum. The concentration of Bro-MnO2 nanosheets was estimated on the basis of: A = εbc.
2.3 Biosensing of dopamine
A total of 80 μL of 100 mmol L−1 NaAc–HAc buffer (pH = 4.75), 15 μL of 5 μmol L−1 Bro-MnO2, 30 μL of 5 mmol L−1 TMB solution, and dopamine solution with different concentrations were added in sequence to an EP reaction tube. After being fully mixed, the mixture was reacted in a constant-temperature mixer at 37 °C for 20 min. The A652 value of the system was measured by UV-2250 UV-Vis spectrophotometer colorimetry and recorded.
2.4 Selectivity
To evaluate the specificity of the biosensors to dopamine, interferents with a concentration of 100 μM, including fructose, saccharose, lactose, maltose, glucose, NaCl, KCl, CaCl2, ZnCl2, NH4Cl, MgCl2, urea, proline, lysine, folic acid, glycine, tyrosine, tryptamine, tryptamine, β-phenethylamine (PEA), histamine, glutathione + NEM, ascorbic acid + 5 mM AAQ-2, L-adrenaline, norepinephrine (NE) and isoprenaline hydrochloride were added to the system, and the UV-Vis spectra were recorded.
2.5 Biological sample feasibility
To estimate the feasibility of the biosensors in practical applications, serum samples from six healthy volunteers were obtained with permission. Serum samples were collected in red tubes (no additives) to avoid interference from other substances. After blood coagulation for 30 min, human serum samples were centrifuged (10
000 rpm, 10 min). Then, dopamine solutions with concentrations of 0.25 μM, 3.75 μM and 7.5 μM were added to blank samples of human serum and bovine serum, and recovery experiments were carried out. Before testing, 0.3 mM NEM was added to remove the effect of thiols in serum. The determination procedures used for these samples were the same as those used for the dopamine determination in Section 2.3. The results of this determination procedure and the ELISA kit were analyzed by SPSS. All experiments complied with relevant laws and institutional guidelines.
3. Results and discussion
3.1 Preparation and surface features of Bro-MnO2
We synthesized Bro-MnO2 by using a biotemplate method. In brief, under basic conditions, bromelain was used as a biotemplate to synthesize templated Bro-MnO2via the spontaneous oxidation of MnCl2 at room temperature in air. TEM was first used to observe the morphology (Fig. 1A); the results indicated that Bro-MnO2 was formed by the interlaced stacking of many irregular 2D networks with laminated structures, a large surface area and surface wrinkles. Analysis by dynamic light scattering (DLS) revealed that the average diameter was 220.9 nm (Fig. S2, ESI†). Due to their uniform network dispersion, the Bro-MnO2 nanosheets had a large contact area and dispersion, which are beneficial for the full reaction with TMB.
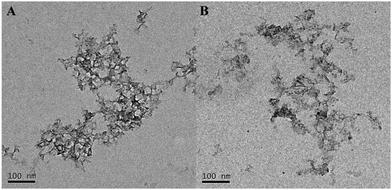 |
| Fig. 1 TEM images of (A) bromelain-MnO2 nanosheets and (B) nanosheets with 10 μM dopamine. | |
Under natural light conditions, the synthesized aqueous solution of Bro-MnO2 was brown (Fig. 2A), and after storage at 4 °C for 30 days (Fig. S3, ESI†), the value of absorbance remains stable and the solution was still clear with no precipitate, indicating that the synthesized Bro-MnO2 nanosheets have good stability.
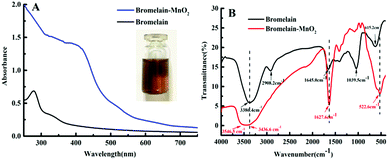 |
| Fig. 2 (A) UV absorption spectrum; (B) Fourier transform infrared spectrum of bromelain (2 mg mL−1) and bromelain-MnO2 (135 μM). | |
The bromelain solution had absorption peaks of phenyl groups between 280 and 290 nm, which was caused by the residues of aromatic amino acids. The UV absorption spectrum of Bro-MnO2 (Fig. 2A) showed the disappearance of the characteristic absorption peak of phenyl groups in bromelain and a broad absorption band in 250–750 nm, with the largest absorption at 385 nm. These results indicated that amino acid groups, for instance –COOH and –NH2, reacted during the synthesis process.20 The secondary protein structure is closely related to hydrogen bonds. For example, α-helices, β-sheets, and β-turns have specific hydrogel bonding properties.21 The structure of Bro-MnO2 was further studied using Fourier transform infrared spectroscopy (FTIR). The tensile vibration of –NH and the bending vibration of –OH corresponded to the strong absorption peak of bromelain at 3386.4 cm−1, and the stretching vibration of –CH2 was verified at 2908.2 cm−1.22
A broad absorption peak appeared at 3546.5–3436.6 cm−1 for Bro-MnO2, which resulted from the stretching vibration of –OH on the surface of the material. Additionally, the FTIR spectrum of Bro-MnO2 showed a broad absorption peak at 522.6 cm−1, which corresponded to Mn–O bonds.23 The amide-I band at 1600–1700 cm−1 is extraordinary sensitive to structural variations in proteins and is closely related to the stretching vibration of C–O and C–N in peptide bonds.24,25 The peak intensity of the amide-I band increased as the absorption peak shifted from 1645.0 cm−1 to 1627.6 cm−1. In addition, the amide-II band of bromelain at 1500–1600 cm−1 and the amide-III band at 1230–1240 cm−1 decreased with the disappearance of the absorption peak at 1039.5 cm−1, showing that the formation of Bro-MnO2 was mainly related to the secondary structure of bromelain proteins, such as α-helices and β-sheets.
The X-ray photoelectron spectroscopy (XPS) spectrum of MnO2 showed all expected elements (Fig. S4, ESI†), including C1s, N1s, O1, Mn2p and Mn3s. To further study the valence state of Mn, we observed the characteristic absorption peak at 653.54 eV, which corresponds to Mn2p1/2; the other absorption peak at 641.94 eV corresponds to Mn2p3/2. The Mn3s frequency spectrum showed ΔE ≈ 4.95 eV. Compared with results reported in previous studies,24,26 the peak separation of MnO and Mn2O3 differed, suggesting the presence of Mn4+ in the laminated structure. The results verified the successful preparation of MnO2.
3.2 Working principle of the Bro-MnO2 biosensor
The Bro-MnO2 nanosheets were further analyzed. Due to the high oxidase activity, TMB could be directly oxidized to form blue products without H2O2. The oxidase activity was verified by the absorption peak at 652 nm. As shown in Fig. 3A, TMB (e) was a colorless and transparent solution without any characteristic absorption peaks at 350–750 nm, while the Bro-MnO2 sheets (d) were light brown with a maximum absorption peak at 385 nm. In the presence of O2, the Bro-MnO2 nanosheets catalyzed the formation of a deep blue color from TMB within 5 min, and the oxTMB products were verified by the maximum absorption peak at 652 nm.
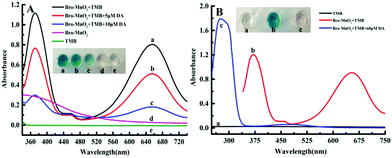 |
| Fig. 3 Dopamine-induced reduction of bromelain-MnO2 oxidase activity by UV absorption: (A) absorption spectrum under acidic conditions of pH 4.75, 100 mM NaAC–HAC: (a) Bro-MnO2 + TMB, (b) Bro-MnO2 + TMB + 5 μM DA, (c) Bro-MnO2 + TMB + 10 μM DA, (d) 5 μM Bro-MnO2, (e) TMB. (B) UV-Vis absorption diagram of quinone compounds catalyzed by Mn2+ in the system: (a) TMB, (b) TMB + Bro-MnO2, (c) TMB + Bro-MnO2 + 60 μM DA. | |
The morphology and size of nanomaterials have a very important influence on their catalytic performance. As observed in the TEM images (Fig. 1B), the Bro-MnO2 nanosheets dispersed from a network aggregate into many small lamella as dopamine was added, resulting in a significant reduction in size. The UV absorption spectrum (Fig. 3B) showed that the blue color of the solution faded when dopamine solution was added at different concentrations, with a corresponding decrease in the absorption peak at 652 nm. These results suggested that the redox reaction between the dopamine and MnO2 sheets caused the decomposition of the MnO2 laminated structure, leading to a reduction in size and hence the inhibition of oxidase activity. In addition, high-concentration dopamine solution could completely reduce MnO2 to Mn2+. Mn2+ ions could accelerate the oxidation of dopamine, leading to the formation of benzoquinone compounds. The solution was light pink and exhibited absorption corresponding to oxidized dopamine at 280 and 480 nm, while the absorption peak of oxTMB at 652 nm completely disappeared. In summary, dopamine could be quantified by using Bro-MnO2 biosensor colorimetry, either by visually observing the color change or by measuring the A652 value.27
3.3 Colorimetric sensing of dopamine based on Bro-MnO2
A MnO2–TMB color rendering platform was used to develop the Bro-MnO2 nanozyme colorimetric sensing method, which was applied in dopamine determination. First, we studied the influence of the experimental conditions on the signal response and found that the best response (Fig. S5 and S6, ESI†) was obtained under the following conditions: (A) the volume of NaAC–HAC buffer (pH = 4.75, 100 mM) was 80 μL; (B) the concentration of TMB was 0.375 mM; (C) the concentration of MnO2 nanosheets was 5.0 μM; (D) the reaction temperature was 37 °C; and (E) the reaction time was 20 min. Under the aforementioned best experimental conditions, the UV absorption spectra further showed the feasibility of the biosensing of dopamine depending on Bro-MnO2 nanosheets.
The oxidation efficiency of TMB decreased under the influence of the reductive analyte dopamine (Fig. 4), leading to a gradual reduction in oxTMB concentration and a steady decline in the A652 value. Therefore, dopamine could be analyzed quantitatively. When the concentration of dopamine gradually increased between 0 and 10 μM, a gradually disappearance of blue color was observed with the naked eye and corresponded with a decrease in the A652 value. Similarly, the absorption intensity of oxTMB depended on the concentration of dopamine. When the dopamine concentration was in the range of 0.1–10 μM, a good linear correlation with the difference in absorbance (ΔA652) was observed: ΔA652 = 0.0672CDA + 0.0496, R2 = 0.997. According to the limit of detection (LOD) = 3stb/k, the LOD was 39.8 nM. Parallel determinations (n = 11) of dopamine solution with concentrations of 0.5 μmol L−1, 3.75 μmol L−1 and 7.5 μmol L−1 yielded relative standard deviations (RSD) of 0.23%, 0.34%, and 0.42%, respectively, indicating good repeatability of this method. A simple, fast, and efficient biosensing platform for detecting dopamine was therefore established. Compared with the recently reported method that utilized enzyme mimic colorimetry to detect dopamine (Table 1), this method had a relatively low LOD. The reasons might be as follows: (1) this method used the oxidase activity of Bro-MnO2 without the addition of H2O2, which significantly enhanced the consistency of the color rendering platform and the reaction rate of the dopamine and MnO2 sheets. (2) Mn2+ ions were generated that could catalyze the oxidation of dopamine. This reaction mechanism greatly improved the selectivity and response speed of the sensor. Therefore, this method shows great potential in colorimetric sensing analysis of dopamine and can meet actual needs.
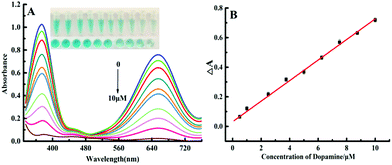 |
| Fig. 4 (A) Observed UV absorption spectra at dopamine concentrations of 0.1, 1.0, 2.5, 3.75, 5.0, 6.25, 7.5, 8.75, and 10.0 μM. (B) The relationship between ΔA and concentration of dopamine. | |
Table 1 Comparison of developed methods for dopamine determination
Nanomaterials |
Technique |
Range of linearity (μM) |
LOD (μM) |
Ref. |
p-ProH/GCE |
Electrochemistry |
1–100 |
0.3 |
28
|
GO–BAMB–Co(OH)2 |
Electrochemistry |
3–20 |
0.4 |
29
|
25–100 |
|
g-C3N4/GO/GCE |
Electrochemistry |
0.25–40 |
0.07 |
30
|
Graphene quantum dots |
Fluorescence |
0.25–50 |
0.09 |
31
|
MPA-CuInS2 QDs |
Fluorescence |
0.5–40 |
0.2 |
32
|
Cyan-emitting carbon dots (CDs) |
Fluorescence |
0–40 |
0.18 |
33
|
Cu–Mn–O/C-dots |
Colorimetry |
0–75 |
0.5 |
34
|
Fluorescence |
0–100 |
0.125 |
PtNPs@MnO2 nanocomposite |
Colorimetry |
0.1–1.1 |
0.025 |
16
|
NiCo2S4–rGO |
Colorimetry |
0.5–100 |
0.42 |
35
|
S-CDs@AuNPs |
Colorimetry |
0.81–16.80 |
0.77 |
36
|
Paper-based analytical devices (PADs) |
Colorimetry |
0.527–4.75 |
0.37 |
37
|
Bromelain-MnO2 |
Colorimetry |
0.1–10 |
0.04 |
This work |
3.4 Selectivity of dopamine determination
In the complex environment of biological samples, high selectivity for the target is an important basis for evaluating the application of biosensors. We studied the influence of different interfering substances on dopamine colorimetric sensing by using a relative error not exceeding ±5% as the standard. Na+, K+, Ca2+, Zn2+, NH4+, Mg2+, fructose, saccharose, lactose, maltose, glucose, urea, cysteine, proline, lysine, folic acid, tryptamine, tryptamine, β-phenethylamine (PEA), histamine, and glycine at a concentration of 100 μM (100 times higher than the dopamine solution concentration) was added to the system, as well as uric acid and glycine at a concentration of 80 μM (80 times higher than the dopamine solution concentration), 1 mM ascorbic acid (AA) + 5 mM AAQ-2. These additives had no obvious interference effects on the sensor (Fig. 5). Glutathione had some interference in this system, but the interference could be reduced after the addition of a masking agent (N-ethylmaleimide, NEM). The determination of dopamine was not influenced by the addition of NEM. The high concentration of ascorbic acid has serious interference with this assay system. The need to enter 5 mM AAQ-2 can reduce the interference without affecting the determination of dopamine (Fig. S7, ESI†).
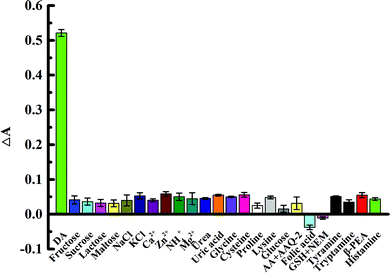 |
| Fig. 5 Intensity of the response of the biosensor to dopamine over coexisting substances: dopamine (1 μM), uric acid and glycine (80 μM), AA (50 μM), GSH (100 μM) with 0.3 mM NEM; the other interfering substances were present at 100 μM. | |
Therefore, these results indicate good selectivity and sensitivity for the determination of dopamine using biosensors and great potential for the quantitative analysis of dopamine in biological samples.
3.5 Biological sample analysis
To verify the feasibility of the biosensor, dopamine was tested in human serum and bovine serum by spiking blank samples. The content of dopamine was quantitatively tested by using the method in Section 2.5. Verification of the colorimetric sensing reliability, as shown in Table S1 (ESI†), indicated that the recovery rate of dopamine was 96.0% to 97.2% in 1% human serum samples. For the bovine serum samples, the recovery rate was 102% to 104%, and the RSD was 1.23% to 2.36%. These results indicated the good accuracy of the proposed biosensor for serum samples.
To further confirm the reliability of the assay results, we collected serum samples from 6 healthy volunteers. We performed paired-sample T tests and utilized an ELISA kit or this method to test the samples (Table S2, ESI†). The data were analyzed by SPSS software. The statistical analysis results showed t = 0.319 and v = 5 in the 95% confidence interval. By comparison with the theoretical value t(5,95%) = 2.571, we obtained P > 0.05. Since H0 cannot be rejected according to a = 0.05, the results of the two testing methods for dopamine in serum showed no significant difference. Hence, these Bro-MnO2-based biosensors could be used for serum sample testing and exhibited great potential in clinical applications.
4. Conclusions
Bromelain-templated MnO2 nanosheets were developed for low-cost, fast, and high-selectivity colorimetric sensing; this method can be used to test dopamine in serum samples. The MnO2 nanosheets synthesized by biotemplating are environmentally friendly and can be easily prepared at a very low cost. Furthermore, MnO2 has outstanding oxidase activity. It can be reduced to Mn2+ by dopamine, which accelerates the auto-oxidation of dopamine and produces a light pink benzoquinone compound. The rapid testing of dopamine in serum samples with good selectivity was realized. However, this sensor mainly relies on the occurrence of redox reactions. High concentrations of strongly reducing substances such as glutathione and ascorbic acid, which have an impact on the measured data, need to be added to reduce the corresponding masking agent. In conclusion, this method represents a new strategy for biosensing applications.
Conflicts of interest
Compliance with ethical standards, the authors claim that there are no competing interests.
Acknowledgements
The authors express sincere gratitude for the support of the National Natural Science Foundation of China (No. 81473021), the Foundation for Graduate Research and Innovation Project of Hunan Province (No. CX2017B557), and the Key Laboratory Project in Hengyang (2018KJ110).
Notes and references
- J. Wu, X. Wang, Q. Wang, Z. Lou, S. Li, Y. Zhu, L. Qin and H. Wei, Chem. Soc. Rev., 2019, 48, 1004–1076 RSC.
- M. Liang and X. Yan, Acc. Chem. Res., 2019, 52, 2190–2200 CrossRef CAS.
- N. K. S. Soltani, T. Shean, Y. Choonga and U. Rashid, New. J. Chem., 2020, 44, 9581–9606 RSC.
- L. B. G. Fernández, A. Villanuevaa and R. Pleixats, New J. Chem., 2020, 44, 6130–6141 RSC.
- T. W. R. Xie, S. Bai, Z. Wang, F. Chai, L. Chen and S. Zang, New J. Chem., 2020, 44, 9684–9690 RSC.
- F. Attar, M. G. Shahpar, B. Rasti, M. Sharifi, A. A. Saboury, S. M. Rezayat and M. Falahati, J. Mol. Liq., 2019, 278, 130–144 CrossRef CAS.
- L. J. Yu Wu, Xin Luo, W. Q. Xu, X. Q. Wei, H. J. Wang, H. Y. Yan, B. Z. X. W. L. Gu, D. Du, Y. H. Lin and C. Z. Zhu, Small, 2019, 15, 1–7 Search PubMed.
- U. A. Syed, M. Husnain, A. Yaqub, F. Shahzad and N. Abbas, New J. Chem., 2020, 44, 6096–6120 RSC.
- J. Chen, H. Meng, Y. Tian, R. Yang, D. Du, Z. Li, L. Qu and Y. Lin, Nanoscale Horiz., 2019, 4, 321–338 RSC.
- Z. Zhao, H. Fan, G. Zhou, H. Bai, H. Liang, R. Wang, X. Zhang and W. J. J. O. T. A. C. S. Tan, J. Am. Chem. Soc., 2014, 136, 11220–11223 CrossRef CAS.
- K. Xing, J. Ge, W. X. Wang, X. Geng, X. P. Shen, J. L. Tang, L. B. Qu, Y. Q. Sun and Z. H. Li, New J. Chem., 2019, 43, 9466–9471 RSC.
- Y. Q. Deng, W. Huang and Y. He, Biosens. Bioelectron., 2017, 91, 89–94 CrossRef.
- E. Z. Sheng, Y. T. Tan, Y. Xiao, Z. X. Li and Z. H. Dai, Food Chem., 2020, 331, 1–6 CrossRef.
- X. L. C. Y. Xiong, H. G. Liu, M. R. Li, B. L. Li, S. S. Jiao, W. Zhao, C. Duan, L. Dai and Y. H. Ni, J. Electrochem. Soc., 2019, 166, A3965–A3971 CrossRef.
- T. Liu, J. Tian, L. Cui, Q. Liu, L. Wu and X. Zhang, Colloids Surf., B, 2019, 178, 137–145 CrossRef CAS.
- J. Liu, L. Meng, Z. Fei, P. J. Dyson and L. Zhang, Biosens. Bioelectron., 2018, 121, 159–165 CrossRef CAS.
- J. Ge, K. Xing, X. Geng, Y.-L. Hu, X.-P. Shen, L. Zhang and Z.-H. J. M. A. Li, Microchimica Acta, 2018, 185, 559 CrossRef.
- C. Ren, J. Ge, X. Chen, Q. Wu, L. Zhang, J. Jiang, C. Cui, S. Wan and W. Tan, Talanta, 2019, 195, 40–45 CrossRef.
- S. Liu, C. Lv, R. Liu, G. Yang, S. Li, L. Zuo and P. Xue, New J. Chem., 2019, 43, 13143–13151 RSC.
- S. Niu, Y. Fang, K. Zhang, J. Sun and J. Wan, Instrum. Sci. Technol., 2017, 45, 101–110 CrossRef CAS.
- F. Mohamed Tap, F. A. Abd Majid, H. F. Ismail, T. S. Wong, K. Shameli, M. Miyake and N. B. Ahmad Khairudin, Molecules, 2018, 23, 73 CrossRef.
- F. M. Croisfelt, J. A. Ataide, L. L. Tundisi, L. C. Cefali, M. de Araújo Rebelo, J. L. D. Sánchez, T. G. da Costa, R. Lima, A. F. Jozala and M. V. Chaud, Eur. Polym. J., 2018, 105, 48–54 CrossRef CAS.
- Z. Lin, M. Li, S. Lv, K. Zhang, M. Lu and D. Tang, J. Mater. Chem. B, 2017, 5, 8506–8513 RSC.
- K. Li, C. Chen, H. Zhang, X. Hu, T. Sun and J. Jia, Appl. Surf. Sci., 2019, 496, 143662 CrossRef CAS.
- T. Lefèvre and M. Subirade, J. Colloid Interface Sci., 2003, 263, 59–67 CrossRef.
- Y. Xiao, W. Huo, S. Yin, D. Jiang, Y. Zhang, Z. Zhang, X. Liu, F. Dong, J. Wang and G. Li, J. Colloid Interface Sci., 2019, 556, 466–475 CrossRef CAS.
- F. Tian, J. Zhou, B. Jiao and Y. He, Nanoscale, 2019, 11, 9547–9555 RSC.
- D. Kong, Q. Zhuang, Y. Han, L. Xu, Z. Wang, L. Jiang, J. Su, C.-H. Lu and Y. Chi, Talanta, 2018, 185, 203–212 CrossRef CAS.
- A. Ejaz, Y. Joo and S. Jeon, Sens. Actuators, B, 2017, 240, 297–307 CrossRef CAS.
- L. Zhang, C. Liu, Q. Wang, X. Wang and S. J. M. A. Wang, Microchim. Acta, 2020, 187, 149 CrossRef CAS.
- J. Zhao, L. Zhao, C. Lan and S. Zhao, Sens. Actuators, B, 2016, 223, 246–251 CrossRef CAS.
- S. Liu, F. Shi, X. Zhao, L. Chen and X. Su, Biosens. Bioelectron., 2013, 47, 379–384 CrossRef CAS.
- M. Lan, S. Zhao, X. Wei, K. Zhang, Z. Zhang, S. Wu, P. Wang and W. Zhang, Dyes Pigm., 2019, 170, 107574 CrossRef CAS.
- J. Wang, R. Du, W. Liu, L. Yao, F. Ding, P. Zou, Y. Wang, X. Wang, Q. Zhao and H. Rao, Sens. Actuators, B, 2019, 290, 125–132 CrossRef CAS.
- Y. Wang, L. Yang, Y. Liu, Q. Zhao, F. Ding, P. Zou, H. Rao and X. Wang, Microchim. Acta, 2018, 185, 496 CrossRef.
- M. Amiri, S. Dadfarnia, A. M. H. Shabani and S. Sadjadi, J. Pharm. Biomed. Anal., 2019, 172, 223–229 CrossRef CAS.
- C. Liu, F. A. Gomez, Y. Miao, P. Cui and W. Lee, Talanta, 2019, 194, 171–176 CrossRef CAS.
Footnote |
† Electronic supplementary information (ESI) available: Optimization of experimental factors, recovery results for spikes and methodological verification results. See DOI: 10.1039/d0nj05066k |
|
This journal is © The Royal Society of Chemistry and the Centre National de la Recherche Scientifique 2021 |
Click here to see how this site uses Cookies. View our privacy policy here.