DOI:
10.1039/D0NJ04923A
(Paper)
New J. Chem., 2021,
45, 314-320
Self-healing hydrogel sensors with multiple shape memory properties for human motion monitoring†
Received
7th October 2020
, Accepted 20th November 2020
First published on 26th November 2020
Abstract
Shape memory hydrogels offer new opportunities for the development of smart wearables due to their intelligent responsiveness. In this study, a self-healing hydrogel with multiple shape memory properties was prepared via one-step polymerization. The synergy of dynamic electrostatic interactions and imine bonds afforded a physically and chemically dual-crosslinked hydrogel with high stretchability (maximum strain of 556%), tensile fracture strength (63 kPa), and self-healing properties (99% healing efficiency at 60 °C within 4 h). Through the reversible construction of physical crosslinking points, the temporary shape of the hydrogel was fixed by two different types of stimuli, pH and metal ions (Ag+, Zn2+, etc.), with the shortest shape fixation time of 15 s. Furthermore, the hydrogel was used to fabricate resistive strain sensors and capacitive pressure sensors, and both types displayed high sensitivity, stability, and cyclability. The sensors could detect various types of human body movement, such as finger bending, swallowing, and pulse, indicating a promising future in smart wearables and healthcare monitoring.
1. Introduction
Shape memory hydrogels are versatile materials with good adjustability and machinability that are desirable for numerous applications in soft robotics, shape memory toys, and smart wearables.1–5 The shape memory properties of hydrogels originate from a dual-segment system: crosslinks to determine the permanent shape and reversible interaction of stimulus responses to fix the temporary shape. At present, to realize the shape memory property in response to a certain type of stimulus, the majority of hydrogels are constructed with specific trigger mechanisms (light,6 heat,7 pH,8 magnetism,9etc.) by designing complex molecular structures.10–24 If two or more non-interference reversible switches are integrated into one integrated system, triple or multiple shape memory properties could be achieved. However, shape memory hydrogels are susceptible to irreversible damage under external stress, which severely limits their broader application.
Self-healing hydrogels, inspired by the properties of biological self-healing,25–30 have emerged as a promising class of intelligent materials that can spontaneously restore their original properties upon the occurrence of damage and have attracted considerable attention for increasing durability, prolonging equipment life, and reducing maintenance costs.31–36 The self-healing mechanism of hydrogels can be divided into two types: external self-healing and internal self-healing. The external self-healing mechanism is usually realized by microcapsules or pipelines, while the internal self-healing mechanism is chemically driven by non-covalent bonds and reversible covalent bonds, such as hydrogen bonds, metal ligand coordination, host guest interactions, Diels Alder, boron esters, urea chemistry and other reactions.37 Currently, most of these hydrogels show solely shape memory or self-healing properties; only a few researchers have developed hydrogels with both shape memory and self-healing properties.38–40 Wang et al. proposed a new class of redox switchable hydrogel systems using donor (dopamine) acceptor (bipyridinium) complexes as crosslinking units for carboxymethyl cellulose. They demonstrated that the redox-triggered donor acceptor-bridged hydrogels showed switchable stiffness that facilitates them to have both shape memory and self-healing properties.41 Romo-Uribe et al. synthesized a physical gel based on copolymers of N-isopropyl acrylamide–polyhedral oligomeric silsesquioxane, which exhibited thermally activated shape memory and self-healing properties.42 Despite the good shape memory and self-healing properties of these hydrogels, the speed of self-healing and the responsive speed of shape memory properties still need to be improved. Therefore, self-healable hydrogels with fast and multi-stimulus shape memory properties are required for applications in highly sensitive sensors with self-healing function.
In this work, a self-healing hydrogel with multiple-shape memory properties was prepared via one-step polymerization of acrylic acid (AA), oxidized dextran (ODex), 2-methylallylamine (MLA), and Fe3+. The self-healing properties of this system originate from the rapid dynamic dissociation and association of imine bonds and electrostatic interactions under certain conditions. Through the reversible construction of physical crosslinking points, the hydrogel displayed shape memory properties in response to two different stimuli, pH and metal ions (Ag+ or Zn2+). Furthermore, the self-healing shape memory hydrogel was used to fabricate resistive strain and capacitive pressure sensors for the detection of various types of human movement, such as finger bending, swallowing, and even the pulse. The shape memory hydrogel-based sensors could form a temporarily fixed shape and maintained close attachment to the skin even during exercise in the presence of sweat, thereby showing great promise for the monitoring of human body movement.
2. Experimental section
2.1 Materials
AA and N,N′-methylenebisacrylamide (MBAA) were purchased from Shanghai Macklin Biochemical Co. Ltd. Iron(III) chloride hexahydrate (FeCl3·6H2O) was purchased from Shanghai Lingfeng Chemical Reagent Co. Ltd. Ammonium persulfate (APS) was purchased from Jiangsu Qiangsheng Chemical Co. Ltd. Glycerol was purchased from Shanghai Shenbo Chemical Co. Ltd. Dextran, MLA, and ethylenediaminetetraacetic acid disodium salt (EDTA-2Na) were purchased from Sinopharm Group Chemical Reagent Co. Ltd. Sodium periodate, hydrochloric acid, sulfuric acid, sodium hydroxide, silver nitrate, and zinc chloride were purchased from Aladdin. All reagents were used as received without further purification.
2.2 Experimental preparation
Preparation of oxidized dextran.
ODex was prepared according to a method described in the literature.43 Dextran (5 g) was dissolved in 200 mL of deionized water. 4 g of sodium periodate (dissolved in 100 mL water) was then added dropwise under a N2 atmosphere and the resulting solution was stirred for 4 h under dark conditions. The equal stoichiometric ratio of glycol was then added and stirring was continued for a further 15 min to remove unreacted sodium periodate. The reaction mixture was then transferred to a dialysis bag and dialyzed against deionized water at 4 °C for 2 d. The dialysate was then lyophilized to afford ODex. The degree of oxidation was determined through the reaction of quantitative aldehyde groups with tert-butazinate (tBC) via carbazone formation. The content of aldehyde groups was determined to be 30% from the 1H NMR spectrum (Fig. S1, ESI†).
Preparation of hydrogels.
Firstly, a fixed amount of AA aqueous solution was prepared with a mass ratio of AA to water of 20%. Then, various amounts of MLA (5 wt% of AA), MBAA (0–0.5 wt% of AA), ODex (4.5 wt% of AA), and FeCl3·6H2O (0–4.5 wt% of AA) were dissolved in an appropriate amount of deionized water and APS (2 wt% of AA) was then added as a thermal initiator. The resulting solution was deaired with argon gas for 10 min and then poured into a polytetrafluoroethylene mold, which was covered with a glass plate. The hydrogel was then obtained by curing at 60 °C for 3 h. After polymerization, it was washed with cold Milli-Q purified water (about 5 °C) to remove residual chemicals and sealed with plastic wrap for 24 h before use.
Resistive sensor.
Hydrogels exhibit good ionic conductivity. The resistance of the hydrogel was measured using a high-precision LCR meter (Tonghui TH2829C). The change in the relative resistance of a hydrogel can be expressed using the following formula:
ΔR/R0 = (R − R0)/R0 × 100% |
where R0 and R are the resistances without and with applied strain, respectively.
On the basis of the conductivity of the hydrogel, a resistive strain sensor was fabricated and the relative resistance change of the hydrogel under various strains was evaluated.
Capacitive sensor.
We also constructed a capacitive pressure sensor by integrating two hydrogel films with a dielectric layer (a polydimethylsiloxane (PDMS) membrane with a thickness of 0.25 mm and area of 20 mm × 40 mm). To prepare the membrane, PDMS and a crosslinker were mixed at a 10
:
1 ratio followed by degassing to remove the bubbles. Then, the uncured mixture was poured into a mold and cured in an oven at 80 °C for 1 h. The capacitance of the hydrogel was measured using the LCR meter and the relative capacitance was calculated using the following equation:
ΔC/C0 = (C − C0)/C0 × 100% |
where C0 and C are the capacitance without and with applied pressures, respectively.
A capacitive pressure sensor was fabricated from the hydrogel, and the relative capacitance of the hydrogel under various pressures was evaluated.
2.3 Characterization
Nuclear magnetic resonance (1H NMR) hydrogen spectroscopy was performed on oxidized dextran in D2O solvent at room temperature using a Bruker Avance 500 MHz nuclear magnetic resonance spectrometer. The morphology of freeze-dried samples sprayed with 30 s gold at 20 keV acceleration voltage was observed with field emission scanning electron microscopy (FESEM, Hitachi S-4800).
Test of mechanical properties.
The mechanical properties of the hydrogel were tested at room temperature (25 °C) on a universal testing machine (QT-6203s). A rectangular sample with a size of 2 × 45 × 1 mm3 was tested under a tensile rate of 10 mm min−1. The hydrogel used in the compression test was cylindrical (d = 10 mm, h = 12 mm), and the compression strain was tested at a compression rate of 5 mm min−1.
Test of self-healing properties.
The prepared hydrogel (2 × 45 × 10 mm3) was cut into two pieces, then the cut surfaces were recontacted and stored in a glass container sealed with plastic wrap at 60 °C. The healing efficiency is defined as:
where σH is the stress of the hydrogel after healing and σ0 is the stress of the original hydrogel.
Test of shape memory performance.
The hydrogel was made into straight strips (1.5 mm × 1.5 mm × 100 mm) at room temperature (25 °C), and then bent into a W shape and immersed in a sodium hydroxide solution for five minutes to fix the temporary shape. Experiments were carried out to determine the minimum time required to fix the temporary shape by continuously reducing the time immersed in the sodium hydroxide solution while ensuring that no changes were made to the fixed temporary shape. The hydrogel can finally regain its permanent shape (straight strips) after it was placed in 0.1 M sulphuric acid solution. The minimum time needed to fix the temporary shape and restore the permanent shape can be recorded. Thus, the shape memory performance of the hydrogel to pH response was tested. In the same way, various shape memory properties of hydrogels were tested in silver nitrate solution – 0.1 M EDTA solution, 0.1 M zinc chloride solution – 0.1 M EDTA solution, seawater – 0.1 M sulfuric acid solution.
3. Results and discussion
3.1 Design of the hydrogels
The prepared double-network hydrogel consisted of a main chain composed of AA-MLA and a second chain formed from ODex, with Fe3+ as a coordinated metal ion. The P(AA-co-MLA)-ODex/Fe3+ hydrogel was prepared via a one-pot method, as depicted in Fig. 1. The hydrogel displayed good self-healing performance (99% healing efficiency at 60 °C within 4 h) and excellent shape memory performance (with the shortest shape fixation time of 15 s) owing to the electrostatic interactions between the AA carboxyl groups and Fe3+ and the imine bonds between the MLA amino groups and the ODex aldehyde groups. Scanning electron microscopy (SEM) images of the freeze-dried hydrogels (Fig. 1b–d) revealed that they were composed of interconnected porous structures, affording a porous three-dimensional network, which is highly beneficial to stretchability. In the FTIR spectrum of the P(AA-co-MLA)-ODex/Fe3+ hydrogel (Fig. S2, ESI†), the C
N Imine bond stretching vibration at 1636 cm−1 was formed. The absorption peak of O–H on the carboxyl group shift to 3343 cm−1 due to the association with –OH on ODex and the formation of the ionic bond with metal ion. The peak at 1453 cm−1 is attributed to the C–H2 bending vibration. Meanwhile, due to the C–O–C asymmetric stretching vibration, a peak is assigned at 1261 cm−1.
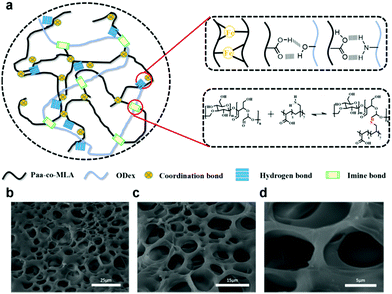 |
| Fig. 1 (a) Preparation of shape memory self-healing hydrogel. (b)–(d) SEM images of the freeze-dried hydrogels. | |
3.2 Mechanical property of the hydrogels
The P(AA-co-MLA)-ODex/Fe3+ hydrogels displayed high flexibility and could withstand various types of deformation, including stretching, twisting, and knotting (Fig. 2a). The tensile properties of the hydrogels were affected by several factors, such as the amounts of MBAA and Fe3+. In an effort to obtain the highest performance, we determined the optimal parameter ratio by independently controlling each variable. Fig. 2b and c present the stress–strain curves for hydrogels with various Fe3+ and MBAA contents. When one of the contents was changed, the other variable used its optimal value. The elongation and fracture stress gradually increased with increasing Fe3+ content. However, when the Fe3+ content was too high, the hydrogel became more brittle, resulting in a significant decrease in strain. Therefore, the stress and strain exhibited a trend of first increasing and then decreasing, reaching a maximum of 63 kPa at 2.8 wt% Fe3+ relative to AA, where the elongation was 556%. Upon increasing the content of MBAA, which serves as the crosslinking agent, the degree of crosslinking in the hydrogels increased, thereby improving the mechanical properties of the hydrogels up to an MBAA content of 0.4 wt% relative to AA, after which the mechanical properties deteriorated. This latter observation may be attributed to decreases in the elasticity and elongation of the hydrogels when the degree of crosslinking exceeded a certain amount. To enhance the persuasive power, we tested the mechanical properties of hydrogels with different concentrations of ODex, as shown in Fig. S3 (ESI†). With the increase of ODex concentration, the mechanical properties of hydrogels first increased and then decreased, mainly because the introduction of ODex increased the cross-linking density of hydrogels. Meanwhile, the concentration of ODex can affect the formation of imine bonds and hydrogen bonds in hydrogels, thus affecting the shape memory and self-healing properties of hydrogels. Therefore, hydrogels with Fe3+, MBAA and ODex contents of 2.8 wt%, 0.4 wt% and 4.5 wt%, respectively, relative to AA were selected as experimental samples to examine the self-healing, shape memory, and some other properties. As shown in Fig. 2d, the hysteresis loop increased with increasing strain. Upon stretching of the hydrogel, more crosslinking points were destroyed as the maximum strain increased, which consumed more energy and led to the increase of the hysteresis loop. As shown in Fig. S5 (ESI†), after the hydrogel was compressed to a certain degree, its interior was compacted, leading to the appearance of a plateau in the compression curve. However, in subsequent tests, the compressed hydrogel spontaneously returned to its original columnar shape after a period of time at room temperature, and this process could be repeated numerous times.
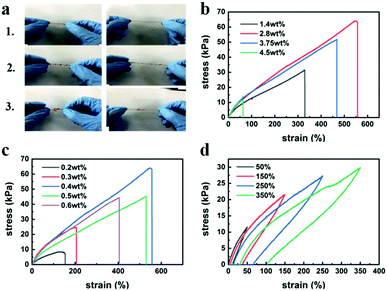 |
| Fig. 2 (a) Ability of the hydrogels to withstand (1) stretching, (2) knotting, and (3) twisting. (b) and (c) Tensile properties of hydrogels with various contents of (b) Fe3+ and (c) MBAA. (d) Tensile loading–unloading curves of hydrogels under various strains. | |
3.3 Shape memory performance of hydrogels
A linear hydrogel sample was folded into a “W” shape and placed in 0.1 M NaOH solution. Under these alkaline conditions, the amino groups formed imine bonds with the aldehyde groups in the hydrogel system. At the same time, the protonation of the carboxyl groups was greatly weakened under the alkaline conditions. Therefore, the electrostatic interactions between the carboxyl groups and Fe3+ ions in the gel were strengthened. Under the combined action of these two mechanisms, the shape could be temporarily fixed. Next, the “W”-shaped sample was placed in 0.1 M H2SO4 solution, which induced cleavage of the imine bonds and protonation of the carboxyl groups, thus weakening the electrostatic interactions between the carboxyl groups and Fe3+ ions and restoring the hydrogel to its original shape. As shown in Fig. 3a, this shape memory behavior could be repeated at least five times. Fig. 3c and e show the shape memory performance of the hydrogels in solutions containing Ag+ and Zn2+ ions owing to the interaction between positive and negative ions. Upon transferring the hydrogels to a solution of EDTA, which binds more readily to the metal ions than the carboxyl groups, the interactions between the metal ions and carboxyl groups and thus the temporary crosslinking points were destroyed with restoration of the original shape. The obtained experimental results demonstrate that the hydrogels possessed shape memory properties with a multi-ion response. Moreover, the shortest fixed time was 15 s, and the recovery time was 5 min, which was better than most of the present shape memory hydrogels.44 Images of the shape memory properties of the hydrogels showed that the shape retention of the hydrogels was excellent even after five cycles, which demonstrated that the shape memory behavior of the hydrogels was extremely repeatable. When the hydrogels were immersed in AgNO3 solution, the Ag+ and Cl− ions in the hydrogels formed a silver-white film on the gel surface owing to the precipitation of AgCl, although this did not affect the shape memory performance. It was also found that the temporary shape of the hydrogels could be fixed in seawater and again recovered in sulfuric acid (Fig. S6, ESI†). It is believed that the soluble salts in seawater are generally interchangeable with their acid salts, the carbonates of which make the seawater alkaline, so that imine bonds can be formed in the hydrogel. In addition, the alkaline environment and metal ions in seawater also make the ionic interactions and binding more stable so as to fix the temporary shape. Furthermore, we tested the shape memory properties by immersing the hydrogels in simulated sweat (3 g L−1 NaCl solution). We found that the hydrogels displayed good shape memory performance in NaCl solution, which demonstrates the feasibility of their application in wearable flexible sensors that fit closely to the skin.
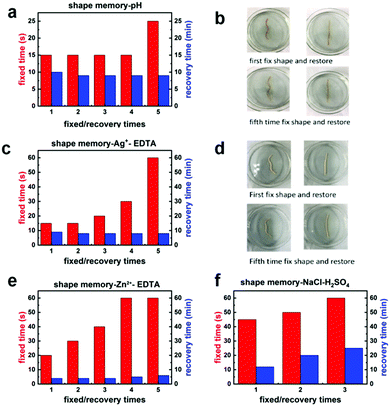 |
| Fig. 3 (a) Shape memory property of the hydrogels in response to pH. (b) Images showing the hydrogels being fixed into temporary shapes and then restored to their original shape in various acids and bases. (c) Shape memory property of a hydrogel in Ag+ solution. (d) Images showing a hydrogel being fixed into a temporary shape in Ag+ solution and then restored to its original shape in EDTA. (e) Shape memory property of a hydrogel in Zn2+ solution. (f) Shape memory property of a hydrogel in simulated sweat (3% NaCl solution). | |
3.4 Self-healing properties of the hydrogels
The electrostatic interactions between the carboxyl groups and Fe3+ ions in the hydrogels endowed these gels with good self-healing properties. To investigate the electrical self-healing ability of the hydrogels, we incorporated a hydrogel into an electronic circuit containing an LED, as depicted in Fig. 4a. The hydrogel was then cut in half using a sharp blade, causing the LED to turn off. Upon placing the two cut surfaces in contact again, the LED immediately turned back on with little change in brightness, indicating immediate restoration of the Fe3+ ion transport channel and electrical conductivity in the hydrogel. To further evaluate the self-healing efficiency of the hydrogels, a hydrogel sample with dimensions of 45 mm × 10 mm × 1.5 mm was prepared. This hydrogel sample was cut in half down the middle and the two cut surfaces were spliced together. Then, the sample was placed in a glass dish and sealed with plastic wrap, and a self-healing test was conducted in a 60 °C oven. At the elevated temperature, the movement of the ions was accelerated and the interaction between the carboxyl groups and Fe3+ ions was enhanced. The mechanical properties of the hydrogel gradually recovered over time, and the degree of self-healing also increased. The fracture stress values of the hydrogel after 1 or 2 h were only 16 and 27 kPa, respectively, corresponding to healing rates of 25% and 43%. After 3 h, the stress at break value had reached 40 kPa, corresponding to a healing rate of 63%. After 4 h, the healing efficiency had reached 99%. When the hydrogel was stretched after healing, no obvious crack was observed at the hydrogel surface, as shown in Fig. 4b, and when it was stretched again after full healing, the hydrogel fracture did not occur in the same place as the original incision.
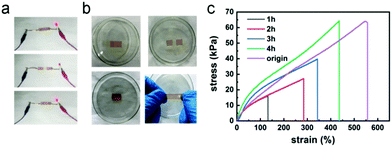 |
| Fig. 4 (a) Conductive and self-healing property of the hydrogels at room temperature. (b) Self-healing property of the hydrogels. (c) Tensile stress–strain curves of hydrogel samples after various healing times. | |
3.5 Resistive and capacitive sensors
To fully exploit the conductivity of the hydrogels, we applied them to the fabrication of resistive and capacitive sensors. For the resistive strain sensor, the two ends of a hydrogel were fixed on a glass slide and connected with conductive copper wire (Fig. S7, ESI†). The resistance of the sensor was then measured using an LCR meter. Upon increasing the strain, the relative resistance of the hydrogel gradually increased. The strain sensitivity of resistance is typically expressed by the strain sensitivity (GF), which is defined as the ratio of relative resistance ((R − R0)/R0) to strain (ε), i.e., ((R − R0)/R0)/ε, where R0 and R are the resistances without and with applied strain, respectively. As shown in Fig. 5a, the strain sensitivity of the hydrogel reached 2.6 at a strain below 200%, and the sensitivity could be increased to 5.5 when the strain was above 200%, which is superior to most existing strain sensors.45–47 In addition, the relative resistance of the hydrogel exhibited good stability and repeatability in 500 cycles of continuous stretching at a strain of 50% (Fig. 5b).
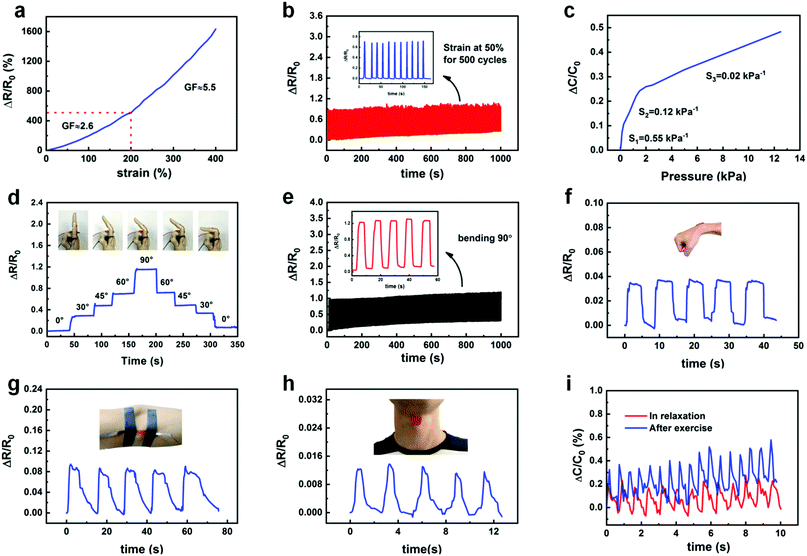 |
| Fig. 5 (a) Variation of the strain sensitivity of the resistive strain sensor with strain. (b) Relative resistance variation of the hydrogel strain sensor upon stretching to 50% strain for 500 cycles. (c) Variation of the sensitivity of the capacitive pressure sensor with pressure. (d) Variation of the relative resistance of the resistive strain sensor adhered to the index finger of a human volunteer at various bending angles. (e) Variation of the relative resistance of the hydrogel during 180 cycles of bending of the index finger. (f)–(h) Variation of the relative resistance of the hydrogel in response to human motion: (f) wrist (bending and straightening), (g) elbow (bending and straightening), and (h) throat (swallowing). (i) Artery wrist pulse output signal for a healthy human volunteer before (red line) and after (blue line) 60 s of movement. | |
For the capacitive pressure sensor, two layers of hydrogel (20 mm × 20 mm × 1.5 mm) were used as the upper and lower conductive active layers, which were separated by a layer of PDMS as the substrate and dielectric layer (Fig. S8, ESI†), and the capacitance was measured using an LCR meter. Upon gradually increasing the load, the relative capacitance also increased in a stable manner (Fig. S9, ESI†). The relative capacitance was restored to the initial value after removing the load. Fig. 5c presents the sensitivity of the capacitive pressure sensor, which is defined as the slope of the trace. The sensitivity of the sensor was 0.55 kPa−1 for the low-pressure range of 0–100 Pa, 0.12 kPa−1 for the high-pressure range of 100–2000 Pa, and 0.02 kPa−1 for pressures exceeding 2000 Pa. Therefore, the capacitive pressure sensor displayed very high sensitivity for the detection of very small pressure changes, indicating its feasibility for measuring minute pressures associated with the human body, such as the pulse.
To evaluate the performance of the hydrogels for use in smart wearables, the resistive strain sensor was attached to the outside of the index finger (Fig. 5d). The electrical resistance of the hydrogel varied upon the natural bending of the finger; a larger bending angle of the finger was associated with higher resistance of the hydrogel. During the bending process, when the bending angle of the finger was kept constant, the deformation of the hydrogel and its relative resistance remained stable. With the gradual straightening of the bent finger, the relative resistance also returned to its original value. Therefore, the bending angle of the finger could be accurately measured from the change in resistance. The resistive strain sensor exhibited high repeatability and sensitivity after 500 cycles of bending to an angle of 90°, as shown in Fig. 5e. These results indicate that the hydrogel-based sensor possessed good durability and stability. To further evaluate the strain sensitivity of the P(AA-co-MLA)-ODex/Fe3+ hydrogel as a strain sensor, the resistive strain sensor was fixed at the wrist, elbow, and throat (Fig. 5f–h). The resistance of the P(AA-co-MLA)-ODex/Fe3+ hydrogel strain sensor again exhibited rapid and repeatable changes in response to movement of the wrist, elbow, and throat (swallowing). We placed a capacitive sensor at the wrist artery pulse to detect small changes in pressure as the pulse was beating. As shown in Fig. 5i, a repeatable and regular pulse pattern was also obtained. By analyzing the output signal frequency of the relative capacitance, the average pulse was determined to be 80 beats per minute, which is equivalent to that of a healthy adult. After 60 s of movement, the amplitude and frequency of the relative capacitance output signal clearly increased. By comparing the frequency before and after movement, the average pulse increased from 80 to 110 beats per minute, confirming the high sensitivity and ultrafast response of the hydrogel-based capacitive sensor.
4. Conclusions
In summary, we developed a self-healing shape memory hydrogel based on imine bonds and electrostatic interactions, which was constructed by physically and chemically dual-crosslinking. By varying the ratio of MBAA and Fe3+, P(AA-co-MLA)-ODex/Fe3+ based hydrogels show a maximum elongation of 556% and a fracture stress of 63 kPa. These hydrogels can be fixed into a temporary shape within 15 s and returned to their original shape within 5 min under optimal conditions. Variation of the pH and the presence or absence of metal ions separately affected the imine bonds and the electrostatic interactions between metal ions and carboxyl groups, which served as a switch for fixing the temporary shape and restoring the original shape. The irreversible covalent bonds could also hold the original shape and the shape memory process could be cycled at least five times. Furthermore, the dynamic nature of the electrostatic interactions also determined the self-healing ability of the hydrogels, which reached 100% within 4 h at 60 °C. Moreover, we applied the developed hydrogels to the preparation of a resistive strain sensor and a capacitive pressure sensor, which displayed high sensitivity and were successfully used to monitor human body movement (bending of the finger, wrist, and elbow, throat swallowing, and pulse). The obtained results demonstrate the feasibility of applying the developed hydrogels to wearable devices and robotics.
Conflicts of interest
There are no conflicts to declare.
Acknowledgements
This work was supported by the National Key Research and Development Program of China (2017YFB0307001), the National Natural Science Foundation of China (91648109), Jiangsu Provincial “333” High-level Talent Training Project, and Jiangsu Province Cultivation base for State Key Laboratory of Photovoltaic Science and Technology.
Notes and references
- J. Shang, X. Le, J. Zhang, T. Chen and P. Theato, Polym. Chem., 2019, 10(9), 1036–1055 RSC.
- F. Ullah, M. B. Othman, F. Javed, Z. Ahmad and H. M. Akil, Mater. Sci. Eng., C, 2015, 414–433 CrossRef CAS PubMed.
- M. Hasnat Kabi, T. Hazama, Y. Watanabe, J. Gong, K. Murase and T. Sunada, J. Taiwan Inst. Chem. Eng., 2014, 45(6), 3134–3138 CrossRef.
- A. Lendlein, M. Balk, N. A. Tarazona and O. E. C. Gould, Biomacromolecules, 2019, 20(10), 3627–3640 CrossRef CAS PubMed.
- A. Lendlein, S. Kelch, K. Kratz and J. Schulte, Angew. Chem., Int. Ed., 2002, 41, 2034–2057 CrossRef CAS.
- J. Huang, L. Zhao, T. Wang, W. Sun and Z. Tong, ACS Appl. Mater. Interfaces, 2016, 8(19), 12384–12392 CrossRef CAS PubMed.
- Z. Jiang, B. Li, S. Zhang, Y. Kang, Y. Xiao and X. Gong, Chem. Commun., 2016, 52(70), 10609–10612 RSC.
- J. Liao, J. Huang, T. Wang, W. Sun and Z. Tong, Chin. J. Polym. Sci., 2017, 35(10), 1297–1306 CrossRef CAS.
- X. Zhou, L. Wang, Y. Xu, W. Du, X. Cai, F. Wang, Y. Ling, H. Chen, Z. Wang and Y. Zheng, RSC Adv., 2018, 8(18), 9812–9821 RSC.
- L. Tang, L. Wen, S. Xu, P. Pi and X. Wen, Chem. Commun., 2018, 54(58), 8084–8087 RSC.
- Z. Li, G. Davidson-Rozenfeld, M. Vázquez-González, M. Fadeev, J. Zhang, H. Tian and I. Willner, J. Am. Chem. Soc., 2018, 140(50), 17691–17701 CrossRef CAS PubMed.
- X. Le, W. Lu, J. Zhang and T. Chen, Adv. Sci., 2019, 6(5), 1801584 CrossRef PubMed.
- Q. Zhao, H. J. Qi and T. Xie, Prog. Polym. Sci., 2015, 49, 79–120 CrossRef.
- Z. Li, V. Wulf, C. Wang, M. Vázquez-González, M. Fadeev, J. Zhang, H. Tian and I. Willner, ACS Appl. Mater. Interfaces, 2019, 11, 34282–34291 CrossRef CAS PubMed.
- L. Wang, Y. Jian, X. Le, W. Lu, C. Ma, J. Zhang, Y. Huang, C. Huang and T. Chen, Chem. Commun., 2018, 54(10), 1229–1232 RSC.
- C. Wu, M. Sun, J. Shieh, C. Chen, C. Huang, C. Dai, S. Chang, W. Chen and T. Young, Ultrasonics, 2018, 157–163 CrossRef PubMed.
- H. Xie, K. K. Yang and Y. Z. Wang, Prog. Polym. Sci., 2019, 95, 32–64 CrossRef CAS.
- Y. Wei, Q. Zeng, M. Wang, J. Huang, X. Guo and L. Wang, Biosens. Bioelectron., 2019, 156–162 CrossRef CAS PubMed.
- Y. Cheng, K. Ren, D. Yang and J. Wei, Sens. Actuators, B, 2018, 255, 3117–3126 CrossRef CAS.
- S. Zhou, T. Tian, J. Wang, S. Wu and T. Xiang, Polym. Chem., 2019, 10(25), 3488–3496 RSC.
- J. Leng, S. Li, M. Yang and J. Hu, J. Mater. Res., 2019, 34(10), 1795–1804 CrossRef CAS.
- J. Zheng, P. Xiao, X. Le, W. Lu, P. Théato, C. Ma, B. Du, J. Zhang, Y. Huang and T. Chen, J. Mater. Chem. C, 2018, 6(6), 1320–1327 RSC.
- G. Cirillo, M. Curcio, U. G. Spizzirri, O. Vittorio, E. Valli, A. Farfalla, A. Leggio, F. P. Nicoletta and F. Iemma, Macromol. Mater. Eng., 2019, 304(5), 1800728 CrossRef.
- W. Dai, H. Guo, B. Gao, M. Ruan, L. Xu, J. Wu, T. B. Kirk, J. Xu, D. Ma and W. Xue, Chem. Eng. J., 2019, 356, 934–949 CrossRef CAS.
- S. Dai, X. Zhou, S. Wang, J. Ding and N. Yuan, Nanoscale, 2018, 10(41), 19360–19366 RSC.
- W. Wang, H. Lai, Z. Cheng, Z. Fan, H. Zhang, J. Wang, S. Yu and Y. Liu, Chem. Commun., 2019, 55(79), 11856–11859 RSC.
- K. Miyamae, M. Nakahata, Y. Takashima and A. Harada, Angew. Chem., Int. Ed., 2015, 54(31), 8984–8987 CrossRef CAS PubMed.
- C. L. Lewis and E. M. Dell, J. Polym. Sci., Part B: Polym. Phys., 2016, 54(14), 1340–1364 CrossRef CAS.
- C. Wang, X. Liu, V. Wulf, M. Vázquez-González, M. Fadeev and I. Willner, ACS Nano, 2019, 13(3), 3424–3433 CrossRef CAS PubMed.
- S. L. Banerjee, T. Swift, R. Hoskins, S. Rimmer and N. K. Singha, J. Mater. Chem. B, 2019, 7(9), 1475–1493 RSC.
- S. Wang, M. Liu, L. Gao, G. Guo and Y. Huo, ACS Appl. Mater. Interfaces, 2019, 11(21), 19554–19564 CrossRef CAS PubMed.
- Z. Li, V. Wulf and C. Wang, ACS Appl. Mater. Interfaces, 2019, 11(37), 34282–34291 CrossRef CAS PubMed.
- F. Zhang, L. Xiong, Y. Ai, Z. Liang and Q. Liang, Adv. Sci., 2018, 5(8), 1800450 CrossRef PubMed.
- S. Dai, X. Hu, X. Zhou, N. Yuan and J. Ding, Synth. Met., 2019, 257, 116177 CrossRef CAS.
- K. Zhang, Z. Zhao, J. Huang, T. Zhao, R. Fang and M. Liu, Sci. China Mater., 2019, 62(4), 586–596 CrossRef CAS.
- L. Tang, S. Liao and J. Qu, ACS Appl. Mater. Interfaces, 2019, 11(29), 26346–26354 CrossRef CAS PubMed.
- S. Dai, S. Wang, X. Dong, X. Xu, X. Cao, Y. Chen, X. Zhou, J. Ding and N. Yuan, J. Mater. Chem. C, 2019, 7(46), 14581–14587 RSC.
- S. Zhou, L. Lu, T. Tian, S. Wu and T. Xiang, Polym. Chem., 2019, 10, 1920–1929 RSC.
- J. Nath, A. Chowdhury and S. K. Dolui, Adv. Polym. Technol., 2018, 37(8), 3665–3679 CrossRef CAS.
- T. Yang, M. Wang, F. Jia, X. Ren and G. Gao, J. Mater. Chem. C, 2020, 8(7), 2326–2335 RSC.
- C. Wang, M. Fadeev, M. Vazquezgonzalez and I. Willner, Adv. Funct. Mater., 2018, 28(35), 1803111 CrossRef.
- A. Romo-Uribe and L. Albanil, ACS Appl. Mater. Interfaces, 2019, 11(27), 24447–24458 CrossRef CAS PubMed.
- H. Xiao, W. Lu, X. Le, C. Ma, Z. Li, J. Zheng, J. Zhang, Y. Huang and T. Chen, Chem. Commun., 2016, 52, 13292–13295 RSC.
- Y. Zhang, J. Liao, T. Wang, W. Sun and Z. Tong, Adv. Funct. Mater., 2018, 28(18), 1707245 CrossRef.
- X. Dong, G. Ge, W. Yuan, W. Zhao, Y. Lu, Y. Zhang, W. Wang, P. Chen, W. Huang and W. Si, J. Mater. Chem. A, 2019, 7, 5949–5956 RSC.
- C. Shao, M. Wang, L. Mei, H. Chang, B. Wang, F. Xu, J. Yang and P. Wan, Chem. Mater., 2018, 30, 3110–3121 CrossRef CAS.
- Z. Gao, Y. Li, X. Shang, W. Hu, G. Gao and L. Duan, Mater. Sci. Eng., 2020, 106, 110168 CrossRef CAS PubMed.
Footnotes |
† Electronic supplementary information (ESI) available. See DOI: 10.1039/d0nj04923a |
‡ These authors contributed equally to this work. |
|
This journal is © The Royal Society of Chemistry and the Centre National de la Recherche Scientifique 2021 |