DOI:
10.1039/D0NJ04867D
(Paper)
New J. Chem., 2021,
45, 208-216
CNT-Br/PEDOT:PSS/PAAS three-network composite conductive hydrogel for human motion monitoring
Received
3rd October 2020
, Accepted 23rd November 2020
First published on 10th December 2020
Abstract
Conductive hydrogels are promising flexible conductors for human motion monitoring. However, this kind of wearable strain sensor has often shown weak mechanical properties. To overcome the inherent mechanical weakness and improve the electrical conductivity, CNT-Br/PEDOT:PSS/PAAS triple-network penetrating conductive hydrogels (CBP) are fabricated. The n-butylimidazolium bromide salt-functionalized MWCNTs (CNT-Br) as conductive fillers form a three-dimensional conductive path through the crosslinking agent XR-100, penetrating with the skeleton of crosslinking sodium polyacrylate. The conductive liquid PEDOT:PSS is further evenly dispersed in the penetrating double network to further enhance the electrical conductivity of the hydrogels. CNT-Br without obvious agglomeration significantly improved the thermal stability and mechanical properties of CBP. Moreover, CNT-Br enabled the PEDOT:PSS components to be more evenly dispersed in the PAAS matrix. Due to the good conductive paths inside the hydrogels, the maximum electrical conductivity (CBP10) increases to 10.35 mS cm−1, which is 415% higher than that of pure P1. Our work provides a method to prepare conductive hydrogels with high electrical conductivity and excellent mechanical properties, which can be used for human joint motion detection.
Introduction
Wearable and flexible physical sensors1,2 featuring good mechanical properties and excellent electrical properties have attracted a myriad of research interest in recent years due to their potential applications in human motion and physiological signal monitoring.3,4 External stimuli, such as pressure,5–7 temperature,8 and strain,9–12 can be transformed by these physical sensors into signals that can be measured or recorded.
Conductive hydrogels13 are one of the promising soft conductors due to their good biocompatibility, rubber-like flexibility, and metal-like conductivity.14 Recently, a number of tough and conductive hydrogels have been developed by incorporating conducting polymers15,16 such as polyaniline (PAni), polypyrrole (PPy), and poly(3,4 ethylenedioxythiophene) (PEDOT). Conducting polymers have an intrinsically unstable conjugated backbone, which when doped with appropriate ions can be used to pass both electronic and ionic charges.17 Although there are many kinds of conductive polymers, most of them are insoluble in water, which is determined by the characteristics of the polymers, which limits the applicability of some scenarios. Bayer Company introduced negatively charged sodium polystyrene sulfonate (PSS) into a positively charged conjugated polymer chain poly 3,4-ethylene dioxythiophene (PEDOT) to synthesize a water-soluble PEDOT:PSS conductive liquid, which has good biocompatibility and ultra-high conductivity (even reaches 1 × 103 S cm−1).
However, most hydrogels are easily damaged during stretching, as they have poor tensile properties due to their inherent structural heterogeneity and the lack of an effective energy dissipation mechanism.18 To overcome the inherent mechanical weakness, conductive hydrogels are obtained by incorporating conducting carbon-based nanomaterials including carbon black,19 graphene,20 and carbon nanotube (CNTs)21 into aqueous gels mainly via physical compositing or chemical crosslinking, which show improved mechanical properties because of a nanocomposite-reinforced mechanism.22 However, the electrical conductivity of nanomaterial incorporated hydrogels is a concern due to the random distribution and easy aggregation of nanomaterials in polymer networks, which weakens the conductivity and mechanical properties of the hydrogels. Modifying nanomaterials with functional groups is an effective approach to improve the dispersion of nanomaterials and strengthen interfacial interactions with the polymer network, thus enhancing the physical properties of nanomaterial-reinforced hydrogels.23
Kun Wang et al.24 co-deposited multi-walled carbon nanotubes coated with PEDOT/PSS and an alginate gel on a microwave neural electrode, and then PEDOT/PSS was electrochemically grown through the coating material to form a kind of soft interpenetrating network (IPN) for the improvement of the neural interface. Zexing Deng et al.25 prepared conductive elastic flexible multifunctional hydrogels with self healing ability based on β-cyclodextrin, N-isopropylacrylamide, multiwalled carbon nanotubes and nanostructured polypyrrole, which had great potential applications in pressure-dependent sensors, large-scale human motion monitoring sensors, and self-healing electronic devices. Liu et al.26 produced a highly conductive p-type PEDOT:PSS fiber by a gelation process, which was 3 orders of magnitude higher than that of previous hydrogel fibers. Polat et al.27 investigated gelatin-based conductive hydrogels doped with CNTs, PEDOT:PSS and Ag nanoparticles for use in biopotential measurements.
Moreover, few studies have focused on conductive hydrogels with modified CNTs and PEDOT:PSS conductive liquid as the conducting medium, and the mechanism of the interaction between the two conductive media has been poorly studied. In this paper, a crosslinked sodium polyacrylate skeleton of the conductive hydrogel was used, in which a modified CNT microcrosslinked conductive network was constructed and PEDOT:PSS conductive liquid was added to permeate the entire network, and finally a CNT-Br/PEDOT:PSS/PAAS triple-network penetrating conductive hydrogel was prepared.
For this kind of conductive hydrogel, we studied the thermal stability, mechanical properties, electrical conductivity and response of the hydrogel to the human joint motion. The synergistic effect of the modified CNT with PEDOT:PSS conductive liquid was also investigated by scanning electron microscopy and X-ray energy dispersion spectroscopy.
Experimental
Materials
Sodium polyacrylate (PAAS) was purchased from Shanghai Lingfeng Chemical Reagent Co., Ltd. Multi-walled carbon nanotubes (purity >97%, length 5–15 μm, and diameter 10–20 nm) were purchased from Shenzhen Nanotech Port Co. Ltd (Shenzhen, China). 3,4-Ethylenedioxythiophene (EDOT) and sodium p-styrene sulfonate (SSNa) were purchased from Shanghai Aladdin Biochemical Technology Co., Ltd. Potassium persulfate (K2S2O8, KPS), ferric sulfate (Fe2(SO4)3), sodium dodecyl sulfonate (SDS), sodium-type 732 cation exchange resin, and chlorine-type 717 anion exchange resin were obtained from Sinopharm Chemical Reagent Co., Ltd. Crosslinking agent XR-100 (trimethylolpropane tris[2-methyl-1-aziridinepropionate], CAS. No. 64265-57-2), was obtained from Shanghai Xirun Chemical Co., Ltd.
Synthesis of CNT-Br
Fig. 1 shows our previous modification method of the CNTs.28 Firstly, multiwalled carbon nanotubes (MWCNTs) were chemically oxidized by using HNO3 and H2SO4 (a ratio of 1
:
3) in an ultrasound environment, which were filtered and washed to obtain carboxylic acid-functionalized MWCNTs (CNT-COOH). Carboxylation of the CNT surface was realized by opening the unstable π–π conjugated structure ring on the carbon tube surface. Secondly, CNT–COOH was acyl chlorinated with sulfoxide chloride and then amidated with N-(3-aminopropyl)-imidazole to obtain imidazole-functionalized MWCNTs (CNT-Mi). Finally, CNT-Mi reacted with 1-bromobutane to form n-butylimidazolium bromide salt-functionalized MWCNTs (CNT-Br).
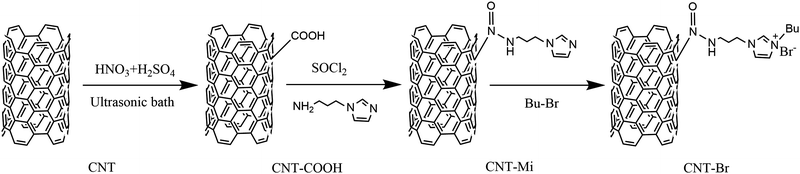 |
| Fig. 1 Synthetic route of ionic liquid functionalized CNTs. | |
Synthesis of PEDOT:PSS conductive liquid
Firstly, 150 g sodium-type 732 exchange resin and 150 g chlorine-type 717 exchange resin were added into 300 ml of 5% sodium chloride solution separately to soak for 12 h. Then, the upper layer liquid was poured out and the resin was rinsed with deionized water several times until the upper layer liquid was nearly colorless. Afterwards, the pre-prepared sodium-type 732 exchange resin and chlorine-type 717 exchange resin were added into 300 ml of 5% NaOH solution and 300 ml of 5% HCl solution correspondingly to soak for 6 h, which were washed repeatedly with deionized water until the decanted liquid appeared neutral. The immersion environment of the two above-mentioned resins was then swapped to do exactly the same. Finally, the 2 kinds of resin were dried for the next use.
After the anion and cation exchange resin was pretreated, 1 g EDOT, 5 g SSNa, 130 ml of deionized water, 0.054 g Fe2(SO4)3, and 0.02 g SDS were added into a 250 ml three-necked flask, which was shaken to obtain a slightly milky white turbid liquid. Then electric agitation was started with 3000 rpm speed for about 5 minutes until the solution obviously became milky white. Subsequently, 2 g K2S2O8 was slowly dropped into the mixture with a low stirring speed about 1500 rpm, and then the rotation speed was adjusted back to 3000 rpm. Meanwhile, the solution started to turn clear blue. After continuous stirring at room temperature for 24 h, 30 g sodium-type 732 cation exchange resin and 30 g chlorine-type 717 anion exchange resin were added into the reaction solution and stirred for 3 h. Finally, the thick blue viscous PEDOT:PSS conductive liquid was filtered with a medical gauze.
Synthesis of the CNT-Br/PEDOT:PSS/PAAS conductive hydrogel
According to the experimental formula in Table 1, different masses of CNT-Br and CNT were added into a beaker of 15 ml of ethanol, which were evenly dispersed by ultrasonic dispersion for 5 minutes. After that, 50 g PEDOT:PSS conductive liquid, 200 g deionized water, and 0.8 g XR-100 cross-linking agent were added into the mixture and evenly dispersed by ultrasonic dispersion for 5 min. Some carboxyl groups on the surface of CNT-Br were cross-linked to form a microcrosslinked network structure, and the solution became uniform blue–black. Then, 3 g PAAS was slowly dropped into the beaker and partially cross-linked. Ultrasonic stirring was continued for 4 h, and PEDOT:PSS conductive liquid was further dispersed in the cross-linked CNT-Br and the cross-linked PAAS. Fig. 2 shows the schematic synthesis diagram of the CNT-Br/PEDOT:PSS/PAAS three-network conductive hydrogel. Then, the reaction solution was placed at room temperature for 12 h for defoaming, and dried in an oven at 50 °C. Finally, the samples taken out from the oven were placed in an environment of 25 °C and 60% relative humidity for 24 h, and then stored in a sealed bag.
Table 1 Formula of the experiment
Sample |
CNT-Br/mg |
CNT/mg |
PEDOT:PSS/g |
EtOH/ml |
H2O/ml |
XR-100/g |
PAAS/g |
P1 |
0 |
0 |
50 |
15 |
200 |
0.8 |
3.0 |
CP6 |
0 |
180 |
50 |
15 |
200 |
0.8 |
3.0 |
CBP4 |
120 |
0 |
50 |
15 |
200 |
0.8 |
3.0 |
CBP6 |
180 |
0 |
50 |
15 |
200 |
0.8 |
3.0 |
CBP8 |
240 |
0 |
50 |
15 |
200 |
0.8 |
3.0 |
CBP10 |
300 |
0 |
50 |
15 |
200 |
0.8 |
3.0 |
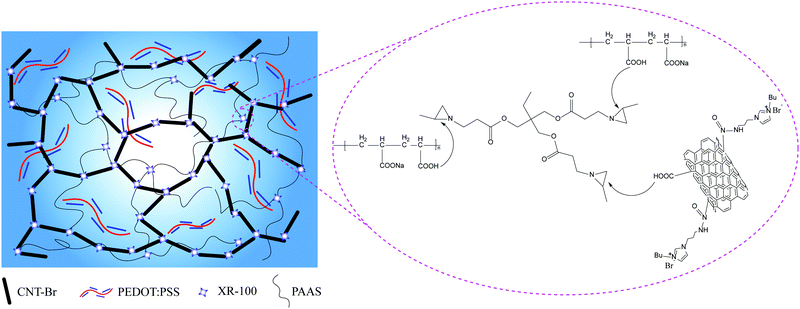 |
| Fig. 2 Schematic synthesis diagram of CNT-Br/PEDOT:PSS/PAAS triple-network conductive hydrogels. | |
CBP4, CBP6, CBP8, and CBP10 were the samples where the weight percentages of CNT-Br to PAAS were 4 wt%, 6 wt%, 8 wt%, and 10 wt%, respectively. And, the P1 sample without a filler and the CP6 sample with 6 wt% CNT filler content were synthesized for experimental comparison.
Characterization
UV-Vis spectroscopy analysis.
3 ml of PEDOT:PSS conductive solution was dissolved in 20 ml of deionized water to prepare a light blue dilute solution. Then, 6 ml of the above-mentioned solution was taken into a cuvette, and the absorbance was tested with a Lambda 950 UV-Visible photometer. The wavelength range was 200–800 nm.
X-ray energy dispersion spectroscopy (EDS) analysis.
EDS is an important method for analyzing the types of micro-components. EDS was used to qualitatively analyze the elements of the synthesized PEDOT:PSS and for the scanning elemental analysis of the SEM feature area of the CNT-Br/PEDOT:PSS/PAAS triple-network conductive hydrogels.
Thermogravimetric analysis (TGA).
Thermal analysis of CP6 and CBP was carried out by using a TG209 F1 thermogravimetric analyzer. The test temperature range was 25–800 °C, and the temperature was increased at a rate of 20 °C min−1 in an air atmosphere. The weight loss of the dry samples was analyzed.
Analysis by a contact angle measuring instrument (CA).
The surface hydrophilicity of CNT-Br/PEDOT:PSS/PAAS triple-network conductive hydrogels was characterized by using a JC2000D3 contact angle measuring instrument. The contact angle values at 5 different places were measured and the average value was taken.
Scanning electron microscopy (SEM) analysis.
The CNT-Br/PEDOT:PSS/PAAS triple-network conductive hydrogels were freeze-dried using a freeze dryer and brittle fracture was carried out in a liquid nitrogen environment. The SEM model of JCM-6000 was used to scan the samples at an accelerated voltage of 15 keV.
Mechanical performance analysis.
The CNT-Br/PEDOT:PSS/PAAS triple-network conductive hydrogels were cut into rectangular splines with a utility knife. And the effective length, width and thickness of the splines were 30 mm, 10 mm and 1 mm, respectively. The mechanical properties of the samples were tested with a small tensile machine CMT2203 at room temperature, and the tensile rate was 1 mm s−1.
Four-probe conductivity analysis.
The sample was made into a size of 30 mm × 10 mm × 1 mm, and the conductivity of CNT-Br/PEDOT:PSS/PAAS was tested by using a four-probe conductivity meter RTS-9.
Tensile fatigue test.
The CNT-Br/PEDOT:PSS/PAAS hydrogels were cut into a size of 30 mm × 10 mm × 1 mm and connected to a digital multimeter at both ends of the sample. The original resistance was recorded as R0. The sample was stretched to a point where the shape variable is 50%, at which point the resistance is marked as R, and repeatedly stretched for 200 times.
Capacitor performance analysis.
The CNT-Br/PEDOT:PSS/PAAS hydrogels were cut into a size of 30 mm × 10 mm × 1 mm, and were directly attached to the part of the human body to be tested, and electrodes were connected to both ends of the sample. The resistance change ratio ΔR/R0 was measured with an electrochemical workstation, where R0 is the resistance value in the natural state and ΔR is the resistance difference between the deformed resistance value and the natural resistance value. This study was performed in strict accordance with the NIH guidelines for the care and use of laboratory animals (NIH Publication No. 85-23 Rev. 1985) and was approved by the Institutional Animal Care and Use Committee of the National Tissue Engineering Center (Shanghai, China).
Results and discussion
UV absorption spectrum analysis of PEDOT:PSS conductive liquid
A UV absorption test was carried out on the aqueous solution of PEDOT:PSS in order to analyze the conjugated system structure of PEDOT:PSS and the transition structure and types of valence electrons. As shown in Fig. 3, the sharp peak at 224 nm corresponds to the π–π* electronic transition on the PEDOT thiophene ring, and the broad absorption peak at 268 nm is attributed to the π–π* electronic transition of the entire conjugated molecular chain of PEDOT, showing clear characteristics of the PEDOT:PSS UV absorption spectrum.
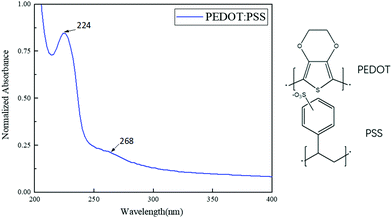 |
| Fig. 3 UV-Vis absorbance spectra of PEDOT:PSS. | |
TGA analysis of CNT-Br/PEDOT:PSS/PAAS triple-network conductive hydrogels
It can be seen from Fig. 4 that the thermal stability of PAAS is very poor and the three hydrogels basically show two main weight loss stages. The first weight loss stage is from 25 °C to 250 °C, and the thermal weight loss is about 20%; the second weight loss stage is from 250 °C to 400 °C, and the thermal weight loss is about 17%.
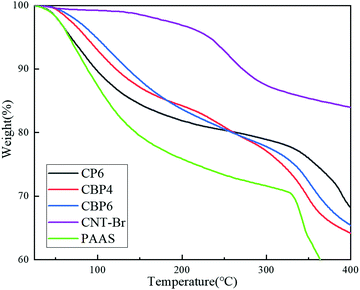 |
| Fig. 4 TGA of CP6, CBP4, CBP6, PAAS and CNT-Br. | |
In the first weight loss stage, all regions of CBP were heated uniformly because of the good dispersibility of CNT-Br which can block the immediate heating of the carboxyl groups, making its decomposition rate slower.29,30 Due to the agglomeration effect of the CNT and part of PEDOT:PSS particles in CP6, the exposed carboxyl groups on PAAS were not fully protected and immediately began to degrade.31 Therefore, CP6 had a faster thermal decomposition rate in the first stage.
In the second weight loss stage, the carboxyl groups in CP6 that were not blocked by the CNT had been completely decomposed in the first stage. The thermal stability of the CNTs played a more important role in the second stage. For CBP, the thermal stability of CNT-Br/PEDOT:PSS/PAAS improved at both stages with the increase of CNT-Br content, which indicated the excellent compatibility of CNT-Br as a filler with PAAS and PEDOT:PSS media.
SEM analysis of CNT-Br/PEDOT:PSS/PAAS triple-network conductive hydrogels
Fig. 6a shows the EDS spectrum of the red marker in Fig. 5a. The elemental sulphur is the characteristic element of PEDOT:PSS, indicating that PEDOT:PSS was not evenly dispersed in the sample of P1. After the addition of sodium polyacrylate, too many free sodium ions in the system prevented the combination of negatively charged PSS and positively charged PEDOT, resulting in the insolubility and agglomeration of part of PEDOT in the system.
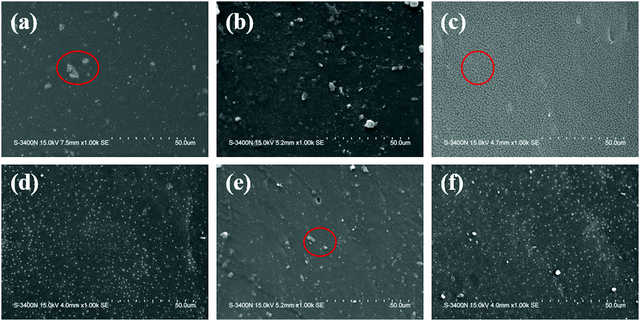 |
| Fig. 5 SEM images of CNT-Br/PEDOT:PSS/PAAS triple-network conductive hydrogels.(a) P1; (b) CP6; (c) CBP4; (d) CBP6; (e) CBP8; and (f) CBP10. | |
As can be seen from Fig. 5b, a large amount of white agglomeration appears, which indicates that the large surface energy of the CNT makes it incompatible with PEDOT:PSS and the PAAS polymer matrix. Even in ultrasonic agitation, CNTs still cannot be dispersed well.
As shown in Fig. 5c, the surface is very smooth and there are no white agglomerated particles when the addition amount of CNT-Br is 4%. The fact not only indicates that CNT-Br disperses well in PAAS, but also promotes the dispersal of PEDOT:PSS in PAAS. When modified carbon nanotubes were added, the negatively charged free bromide ions introduced into the system achieved electrical neutralization with lots of free sodium ions, and PSS could be combined with PEDOT again to improve the dispersibility. It can be seen from Fig. 6c that the three elements N, Br and S are uniformly dispersed in the whole system. N and Br are the characteristic elements of CNT-Br, and S is the characteristic element of PEDOT:PSS. The above facts further indicate that the three components in CBP4 can form a uniform and dense structure.
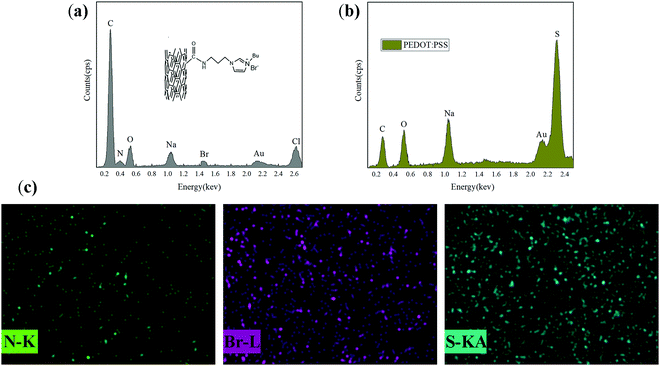 |
| Fig. 6 EDS spectra of CNT-Br/PEDOT:PSS/PAAS triple-network conductive hydrogels. (a) EDS spectrum of the red circle in Fig. 5a; (b) EDS spectrum of the red circle in Fig. 5e; (c) EDS mapping spectra of the red circle in Fig. 5c. | |
As can be seen from Fig. 5d–f, the white agglomeration points gradually appear with the increase of the CNT-Br content. Fig. 6b shows the EDS spectrum of the red marker in Fig. 5e, and the Br element can prove that the agglomeration point is CNT-Br. However, even if the addition amount of CNT-Br was increased to 10%, the agglomeration of CNT-Br was much better than that of CNT in CP6, which further demonstrated the excellent dispersion of CNT-Br and its compatibility with the matrix.
Contact angle analysis of CNT-Br/PEDOT:PSS/PAAS triple-network conductive hydrogels
As shown in Fig. 7, sample P1 which added only PEDOT:PSS has good hydrophilicity, and the water contact angle is only 55°. The addition of CNT with high surface energy built a rough surface of micro-nano structure on the surface of the hydrogel.32 The increased surface roughness of the hydrogel made the hydrogel hydrophobic, so the water contact angle of CP6 increased sharply to 92°.
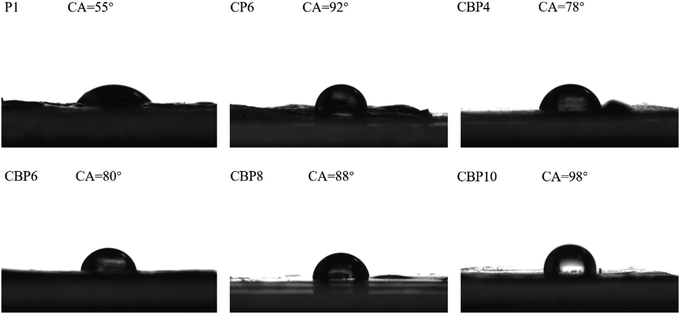 |
| Fig. 7 Water contact angles on the surfaces of CNT-Br/PEDOT:PSS/PAAS triple-network conductive hydrogels. | |
The modified CNT had lower surface energy, which resulted in better dispersion in hydrogels. Compared with the CP6 hydrogel, CNT-Br with the same amount of addition had better dispersibility in the CBP6 hydrogel system, resulting in lower surface roughness of the hydrogel and less hydrophilicity reduction, with a contact angle of 80°. However, with the increase of the CNT-Br amount, CNT-Br in the hydrogel was more likely to aggregate, resulting in a gradual increase of the surface roughness of the hydrogel. The hydrophilicity of CBP10 with higher surface roughness decreased greatly, and the contact angle was 98°, but it was only 6° higher than CP6.
Analysis of the mechanical properties for CNT-Br/PEDOT:PSS/PAAS triple-network conductive hydrogels
Fig. 8 shows the stress–strain curves of CNT-Br/PEDOT:PSS/PAAS triple-network conductive hydrogels. The tensile strength of P1 was only 1.8 MPa and the elongation at break of that was 338%, showing poor tensile strength and toughness. The tensile strength of CP6 containing 6% CNT increased to 2 MPa and the elongation at break decreased to 287%. Although the addition of rigid particles of the CNT slightly improved the tensile strength, at the same time, many agglomeration points of CNT were generated in the matrix (as shown in Fig. 5b), which made the hydrogel prone to stress concentration and it showed poor toughness during the tensile process.
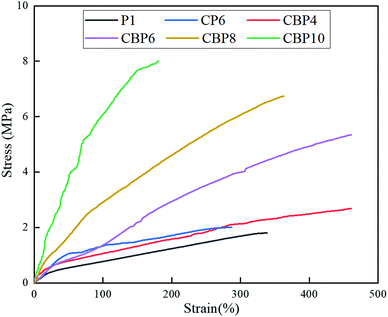 |
| Fig. 8 Stress–strain curves of CNT-Br/PEDOT:PSS/PAAS triple-network conductive hydrogels. | |
But the hydrogels showed better mechanical properties when the rigid particles were replaced by CNT-Br. Compared with pure P1, the tensile strength of CBP4 increases to 2.69 MPa, and the elongation at break also increases to 462%. CNT-Br serving as reinforcing particles not only distributes evenly in the PAAS matrix, but also promotes the uniform dispersion of PEDOT:PSS, which plays an important role of forming a fully penetrating three-network composite hydrogel. In the process of hydrogel stretching, external forces can be evenly dispersed in the material, resulting in the material being uniformly stretched, so that the tensile strength and elongation at break are enhanced. The tensile strength of CBP10 can reach 8.02 MPa, but the elongation at break of that decreased to 181%. Although the tensile strength of the hydrogel increased significantly with the increase of the CNT-Br content, its toughness decreased gradually.
Analysis of the electrical properties of CNT-Br/PEDOT:PSS/PAAS triple-network conductive hydrogels
As shown in Fig. 9a, the conductivity of P1 is 2.01 mS cm−1. Combined with Fig. 5a, it can be seen that PEDOT:PSS is not fully dispersed in the PAAS matrix, leading to the failure to form a complete conductive network. A complete conductive path is still not formed in the CP6 sample with 6% CNT addition because of the aggregation of the CNT, resulting in the conductivity to be only increased by 10%.
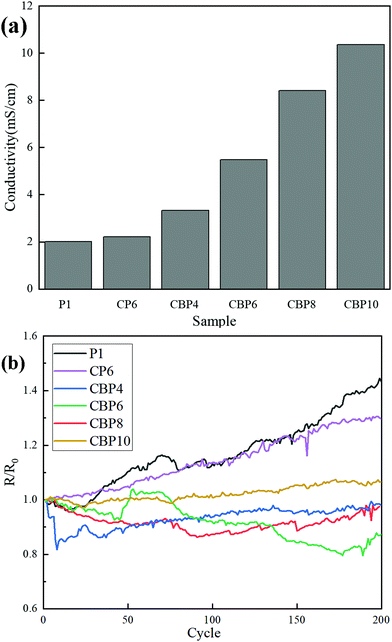 |
| Fig. 9 (a) Conductivity of CNT-Br/PEDOT:PSS/PAAS triple-network conductive hydrogels and (b) resistance change in 200 fatigue stretching cycles of CNT-Br/PEDOT:PSS/PAAS triple-network conductive hydrogels. | |
With the increase of the CNT-Br addition amount, the conductivity of CBP continues to grow. The conductivity of CBP4 is 3.34 mS cm−1 which is higher than that of CP6 with 6% CNT, and the conductivity of CBP10 is 10.35 mS cm−1, which is increased by 415% compared to P1. This is because CNT-Br not only uniformly disperses in the PAAS matrix, but also promotes the uniform dispersion of PEDOT:PSS at the same time, which helps the components in CBP (CNT-Br, PEDOT:PSS and PAAS) to form a penetrating three-network composite hydrogel.
Fig. 9b shows the resistance change in 200 fatigue stretching cycles when the strain is 50%. The rate of change in the resistance is 43% for P1 (added with pure PEDOT:PSS). This is because many microscopic PAAS and PEDOT:PSS conductive paths are broken during the stretching process. The CP6 hydrogel has low tensile strength due to the agglomeration of conductive CNT particles in CP6. And the conductive path is prone to fracture during the stretching process, leading to a resistance change rate of 30% after 200 cycles of stretching. Good conductive paths can be formed owing to the good dispersibility of CNT-Br and the promotion of the dispersibility of PEDOT:PSS, and the conductive paths are not easy to break during the external force stretching process. Therefore, the resistance value is relatively stable, and there is no obvious fluctuation.
Capacitor performance analysis of CNT-Br/PEDOT:PSS triple-network conductive hydrogels
The CBP4 samples which were made into standard test samples were directly attached to the human joint to be tested. And the electrodes were connected to both ends of the sample to test the electrical performance change curve during human joint exercise. Fig. 10a shows the effect of the finger movement on the resistance of the CBP4 sample. This reveals that the resistance change rate gradually increases with the increase of the finger bending angle. The resistance change rate of the finger joint at 30° and 60° is 29% and 50%, respectively. The resistance value increases sharply from 60° to 90°, and the resistance change rate reaches 128% when the finger bending angle is at 90°, and it reaches 151% at 120°. Fig. 10b presents the strain characteristics of the wrist joint. The resistance change rate at the wrist bend is about 65% after repeated stretching and bending 20 times. Fig. 10c shows the strain characteristics of the elbow. The resistance change rate of the elbow bending is about 45% after repeated stretching and bending 20 times. The capacitor performance analysis of the CBP sample provides data support for the CBP capacitance sensor to monitor human joint motion.
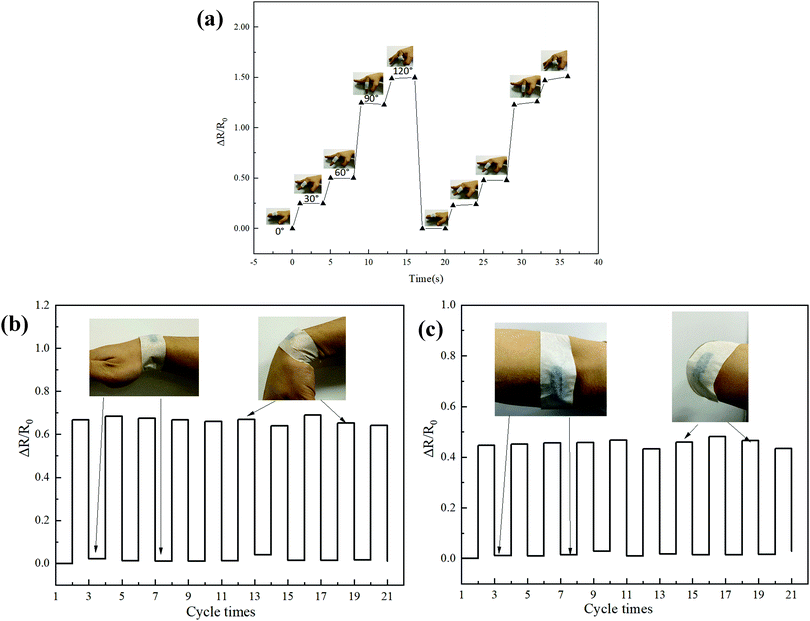 |
| Fig. 10 Electronic properties of strain sensors based on CNT-Br/PEDOT:PSS/PAAS triple-network conductive hydrogels. (a) Strain characteristics on fingers; (b) strain characteristics on the wrist; (c) strain characteristics on the elbow. | |
Conclusions
A three network composite conductive hydrogel with excellent mechanical properties based on CNT-Br, PEDOT:PSS, PAAS, and XR-100 has been successfully prepared. We demonstrated that CNT-Br did not agglomerate as easily as CNT, and it promoted the uniform distribution of PEDOT:PSS conductive liquid in hydrogels. And, the CBP hydrogels showed excellent thermal stability because CNT-Br prevented immediate heating of carboxyl groups on the hydrogel skeleton. Moreover, the excellent dispersibility of CNT-Br reduced the stress concentration of the CBP hydrogels in the process of stretching, which have better tensile strength and elongation at break. Particularly, the synergetic action of CNT-Br and PEDOT:PSS enabled the CBP hydrogels to form good conductive pathways, which can detect the motion of human joints according to the rate of electrical resistance change with human joint bending angle. These conductive hydrogels can be expected to have great potential applications in intelligent electronic devices and wearable strain sensors.
Conflicts of interest
There are no conflicts to declare.
Acknowledgements
The authors sincerely acknowledge the “Shanghai Leading Academic Discipline Project (B502)” and the “Shanghai Key Laboratory Project (08DZ2230500).”
References
- G. F. Cai, J. X. Wang, K. Qian, J. W. Chen, S. H. Li and P. S. Lee, Adv. Sci., 2017, 4, 1600190 CrossRef.
- M. Amjadi, K. U. Kyung, I. Park and M. Sitti, Adv. Funct. Mater., 2016, 26, 1678–1698 CrossRef CAS.
- S. Xia, S. X. Song, F. Jia and G. H. Gao, J. Mater. Chem. B, 2019, 7, 4638–4648 RSC.
- M. H. Liao, P. B. Wan, J. R. Wen, M. Gong, X. X. Wu, Y. G. Wang, R. Shi and L. Q. Zhang, Adv. Funct. Mater., 2017, 27, 1703852 CrossRef.
- Y. Si, L. H. Wang, X. Q. Wang, N. Tang, J. Y. Yu and B. Ding, Adv. Mater., 2017, 29, 1700339 CrossRef.
- Z. Chen, T. Ming, M. M. Goulamaly, H. M. Yao, D. Nezich, M. Hempel, M. Hofmann and J. Kong, Adv. Funct. Mater., 2016, 26, 5061–5067 CrossRef CAS.
- M. A. Darabi, A. Khosrozadeh, R. Mbeleck, Y. Q. Liu, Q. Chang, J. Z. Jiang, J. Cai, Q. Wang, G. X. Luo and M. Xing, Adv. Mater., 2018, 30, 1870023 CrossRef.
- Y. Shi, C. B. Ma, L. L. Peng and G. H. Yu, Adv. Funct. Mater., 2015, 25, 1219–1225 CrossRef CAS.
- Y. Liu, Y. Hu, J. J. Zhao, G. Wu, X. M. Tao and W. Chen, Small, 2016, 12, 5074–5080 CrossRef CAS.
- J. D. Pegan, J. Zhang, M. Chu, T. Nguyen, S. J. Park, A. Paul, J. Kim, M. Bachman and M. Khine, Nanoscale, 2016, 8, 17295–17303 RSC.
- Y. Y. Qin, Q. Y. Peng, Y. J. Ding, Z. S. Lin, C. H. Wang, Y. Li, J. J. Li, Y. Yuan, X. D. He and Y. B. Li, ACS Nano, 2015, 9, 8933–8941 CrossRef CAS.
- S. Ryu, P. Lee, J. B. Chou, R. Z. Xu, R. Zhao, A. J. Hart and S. G. Kim, ACS Nano, 2015, 9, 5929–5936 CrossRef CAS.
- Z. X. Deng, H. Wang, P. X. Ma and B. L. Guo, Nanoscale, 2020, 12, 1224–1246 RSC.
- B. Chua, S. P. Desai, M. J. Tierney, J. A. Tamada and A. N. Jina, Sens. Actuators, A, 2013, 203, 373–381 CrossRef CAS.
- L. Han, X. Lu, M. H. Wang, D. L. Gan, W. L. Deng, K. F. Wang, L. M. Fang, K. Z. Liu, C. W. Chan, Y. H. Tang, L. T. Weng and H. P. Yuan, Small, 2017, 13, 1601916 CrossRef.
- Y. Y. Lee, H. Y. Kang, S. H. Gwon, G. M. Choi, S. M. Lim, J. Y. Sun and Y. C. Joo, Adv. Mater., 2016, 28, 1636–1643 CrossRef CAS.
- R. A. Green, S. Baek, L. A. Poole-Warren and P. J. Martens, Sci. Technol. Adv. Mater., 2010, 11, 014107 CrossRef.
- S. Wang, S. P. Dai, H. Yan, J. N. Ding and N. Y. Yuan, Mater. Res. Express, 2019, 6, 105712 CrossRef CAS.
- W. J. Chuang, W. Y. Chiu and H. J. Tai, J. Mater. Chem., 2012, 22, 20311–20318 RSC.
- H. P. Cong, P. Wang and S. H. Yu, Small, 2014, 10, 448–453 CrossRef CAS.
- L. Bokobza, Polymer, 2007, 48, 4907–4920 CrossRef CAS.
- R. Du, J. X. Wu, L. Chen, H. Huang, X. T. Zhang and J. Zhang, Small, 2014, 10, 1387–1393 CrossRef CAS.
- V. Georgakilas, J. A. Perman, J. Tucek and R. Zboril, Chem. Rev., 2015, 115, 4744–4822 CrossRef CAS.
- K. Wang, L. Tian, T. H. Wang, Z. Q. Zhang, X. J. Gao, L. Wu, B. Fu and X. H. Liu, Compos. Interfaces, 2019, 26, 27–40 CrossRef CAS.
- Z. X. Deng, Y. Guo, X. Zhao, P. X. Ma and B. L. Guo, Chem. Mater., 2018, 30, 1729–1742 CrossRef CAS.
- J. Liu, Y. H. Jia, Q. L. Jiang, F. X. Jiang, C. C. Li, X. D. Wang, P. Liu, P. P. Liu, F. Hu, Y. K. Du and J. K. Xu, ACS Appl. Mater. Interfaces, 2018, 10, 44033–44040 CrossRef CAS.
- T. G. Polat, K. Ates, S. Bilgin, O. Duman, S. Ozen and S. Tunc, Colloids Surf., A, 2019, 580, 123751 CrossRef CAS.
- X. Yuan, H. L. Liu, H. Yu, W. J. Ding, Y. Li and J. K. Wang, J. Appl. Polym. Sci., 2020, 137, e49161 CrossRef.
- X. Tong, H. C. Zhao, T. Tang, Z. L. Feng and B. T. Huang, J. Polym. Sci. Pol. Chem., 2002, 40, 1706–1711 CrossRef CAS.
- Z. L. Li, M. Tang, W. Bai and R. K. Bai, Langmuir, 2017, 33, 6092–6101 CrossRef CAS.
- L. L. Pine and R. Gilbert, Polym. Degrad. Stab., 2002, 75, 337–345 CrossRef.
- Y. Y. Pan, Q. Wu, Y. Y. Weng, X. H. Zhang, Z. H. Yang, J. Q. Meng and O. K. C. Tsui, J. Mater. Chem. A, 2015, 3, 11111–11116 RSC.
|
This journal is © The Royal Society of Chemistry and the Centre National de la Recherche Scientifique 2021 |