DOI:
10.1039/D0NJ03988H
(Paper)
New J. Chem., 2021,
45, 110-122
Radical sensitivity and selectivity in the electrochemical sensing of cadmium ions in water by polyaniline-derived nitrogen-doped graphene quantum dots†
Received
8th August 2020
, Accepted 13th November 2020
First published on 9th December 2020
Abstract
In the present work, the sensing capability of nitrogen-doped graphene quantum dots (N-GQDs) was explored for the first time toward hazardous heavy metal ions and they were found to be able to selectively detect cadmium ions (Cd(II)). Cd(II) is one of the hazardous heavy metal ions on the World Health Organization's list of 10 chemicals of major public health concern. The wide use of Cd(II) in industrial applications and its acute and chronic effects on living beings make the sensing of Cd(II) critical. The aromatic N-GQDs, synthesized here through a hydrothermal route using the single precursor polyaniline exhibited a 25- and 6-fold increase in the current response compared to that of a glassy carbon electrode (GCE) and GQD/GCE, respectively. It showed an excellent and exemplary selectivity toward Cd(II), with almost a nil current response for other interfering metal ions. The LOD value of 1.0 × 10−5 ppb, i.e., in parts per trillion, by N-GQDs is the lowest value ever reported, which was 3–4 orders of magnitude lower than the previously reported values. The LOD was extended to the ultra-high sensitivity level of 1 × 10−7 ppb, i.e., in parts per quadrillion, using a pre-reduction step. The LDR (0.001–112
000 ppb) and LOD values satisfactorily met the sensitivity requirement for detecting Cd(II) within the safe limit specified by the U.S. Environmental Protection Agency (EPA) of ≤5 ppb. The sensing performance of N-GQD/GCE was tested against real environmental samples spiked with Cd(II), such as groundwater, seawater, and wastewater, and was found to perform efficiently.
1. Introduction
Toxic heavy metal ions are a class of contaminants that require rigorous attention because of their inimical toxicity and widespread use.1–5 Because of this, cadmium (Cd), mercury, lead, and arsenic, etc. appear in the World Health Organization's (WHO) list of 10 chemicals of major public health concern. The wide use of Cd(II) in industrial applications, including in Ni–Cd rechargeable batteries, electroplating, cigarette smoke, industrial, and agricultural fields,6,7 and the acute and chronic effects of Cd(II) on health, such as reproductive failure, damage to the central nervous system, heart diseases, emphysema, hypertension, renal damage, DNA damage, and cancer, and on the environment emphasize the need for the strict monitoring of Cd(II) in water sources.8 The maximum permissible limit of Cd(II) in drinking water is ≤5 ppb as per the U.S. Environmental Protection Agency (EPA).8 Therefore, there is a critical need to develop and implement quantitative sensing methods to monitor these components in wastewater and drinking water. Currently, the detection and monitoring of the concentration of these heavy metals, including Cd(II), in environmental samples is done by analytical techniques with instruments that tend to be bulky, expensive, and complex to operate7,9 such as atomic absorption spectrometry,10,11 inductively coupled plasma emission or mass spectrometry,12,13 X-ray fluorescence spectrometry,14 and neutron activation analysis.15 Electrochemical (EC) sensing is an appealing method because of its high inbuilt sensitivity, low-cost, trouble-free operation, rapid analysis capability, and portability,2,16,17 but requires a highly selective and sensitive electrode material because of the complexity of the environmental samples and the ultra-low concentrations (≤5 ppb) of Cd(II) that need to be detected. Previous reports on the selective EC sensing of Cd(II) have utilized different materials, including natural polyphenol extracted from pomegranates and acorns with limit of detection (LOD) values of 0.01 and 0.1 ppb, respectively;18 SnO2 quantum dots with a detection limit of 500 ppb;7 a metal–organic framework (MOF) and polyaniline (PANI)-based material reported by Wang et al., with a 0.3 ppb LOD in the EC sensing of Cd(II);19 a coconut shell powder-modified carbon paste electrode, which showed an LOD of 105 ppb;20 a benzenesulfonyl hydrazide-based electrode with a detection limit of 0.015 ppb;21 and a kaolin platinum electrode (K/Pt) with an LOD of 0.604 ppb.22 Though a few of the reported materials18,19,21 have sensitivity in the applicable concentration range, an ideal electrode material must have good selectivity in addition to a simple synthesis procedure, low cost of production, and be environmentally benign along with possessing the desired sensitivity.
Here in this work, we utilized a non-toxic, environmentally friendly material, namely N-doped graphene quantum dots (N-GQDs), for the EC sensing of Cd(II). The N-GQDs were synthesized through a simple hydrothermal route from PANI using an earlier procedure from our group.23 To the best of our knowledge, there has been no report of EC sensing studies on the use of N-GQDs toward heavy metal ions and so we believe this is the first report on this subject. GQDs are among a new class of emerging carbon-based nanomaterials24,25 with low cytotoxicity and biocompatibility26 and are anticipated to be good potential candidates in the fields of biosensors and bio-imaging devices. Graphene-based nanomaterials, more precisely graphene quantum dots (GQD), have attracted much attention from researchers, mostly due to their exceptional characteristics from the synergistic effects of both graphene and carbon dots. Thus the zero-dimensional (0D) GQDs possess a higher surface area, reliable electrical properties, excellent photoluminescent features, low cytotoxicity, and biocompatibility. Consequently, they are anticipated to be appropriate candidates for biosensors, bio-imaging devices, photovoltaic devices, fuel cells, and light-emitting diodes.27–29 Surface functionalization and heteroatom doping can alter their attributes by generating more active sites, which further enhances the features and facilitates a greater suitability of these materials for these applications.30–33 Here, N-GQDs with an efficient nitrogen (N) doping of ∼10% and an aromatic structure have better electronic characteristics than that of GQDs because of the lower band gap, which enhances the availability of electrons and the conductivity of the material. Further, the doping increases the active sites in the N-GQDs and further enhances the conductivity and electrocatalytic activity, while the crystalline (graphitic) structure also intensifies the conductivity, and together these are expected to facilitate better performance in EC sensing. Interestingly, the N-GQDs-modified GCE (N-GQD/GCE) in this work exhibited a very high sensitivity toward Cd(II), with a limit of detection (LOD) of 0.00001 ppb, i.e., in the ppt range, without a pre-reduction step, while that with the pre-reduction step exhibited an LOD 100 times lower, i.e., 0.0000001 ppb (in the ppq level). The LOD obtained from the slope of the LDR (without the pre-reduction step) was 0.0008 ppb (S/N = 3), i.e., in the ppt range, which is significantly lower than the safe US EPA limit (≤5 ppb). Both the experimental as well as the calculated LOD values are the lowest reported to date for Cd(II) sensing. The N-GODs exhibited a higher selectivity for Cd(II), with no interference from a range of metal ions, including Hg(II), Ag(I), Pb(II), Fe(II), Co(II), Ni(II), Zn(II), Sn(II), Cu(II), and Cr(VI), in the potential range of −1.2 to −0.4 V. The sensing performance was tested with real samples spiked with Cd(II), such as groundwater, seawater, and wastewater, and it was found to be effective.
2. Experimental section
2.1. Reagents and chemicals
Aniline, ammonium peroxydisulfate (APS), chloroform, hydrochloric acid (HCl), and sodium hydroxide (NaOH) were purchased from Merck, India Ltd. HgCl2, AgNO3, CdCl2, NiSO4, ZnNO3, CoNO3, PbNO3, SnCl2, FeSO4, K2Cr2O7, and CuSO4 were purchased from Spectrochem Pvt. Ltd. Distilled water was used in all the procedures unless specified otherwise.
2.2. Characterizations
Field-emission scanning electron microscopy (FE-SEM) was performed on a Zeiss EVO 18 scanning electron microscope with an acceleration voltage of 15 kV. High-resolution transmission electron microscopy (HR-TEM) analyses were done using a JOEL JEM 2100 electron microscope with an accelerating voltage of 200 kV. The universal attenuated total reflection (UATR) mode of Fourier-transform infrared (FTIR) spectroscopy was used for recording the IR spectra using a PerkinElmer Spectrum100 FTIR spectrophotometer. Raman spectroscopy measurements were carried out on a Renishaw inVia Raman microscope with an excitation laser wavelength of 532 nm. The absorption spectra were recorded using a CARY 100 Bio UV-visible spectrophotometer. The PL spectra were recorded on an Horiba FluoroMax-4 system with excitation and emission slits of 1 and 2 nm, respectively. X-ray photoelectron spectroscopy analyses were carried out using an XPS, PHI 5000 Versa Probe II system (ULVAC-PHI Inc., USA), equipped with a micro-focused (200 μm, 15 kV) monochromatic Al-Kα X-ray source (hν = 1486.6 eV). X-ray diffraction (XRD) analyses were carried out on a Bruker D8 discovery small angle X-ray diffractometer, with Cu Kα radiation (λ = 1.54 Å) used as an excitation source.
2.3. Synthesis of the N-GQDs
The procedure for the synthesis of the N-GQDs by our group is reported in ref. 23 in detail. PANI was used as the precursor, and by a simple hydrothermal route, N-GQDs with an aromatic and crystalline nature were obtained.
The GQDs were synthesized from citric acid (1 mM) as the precursor and using a simple hydrothermal route at 180 °C for 4 h.
2.4. Preparation of the electrodes for EC sensing
Prior to the GCE surface modification with the samples, the electrodes were mechanically polished with a wetted micro cloth containing alumina powder, and then cleaned carefully in distilled water by ultrasonication for 2 min. After each analysis, the GCEs were polished as illustrated above and cleaned by ultrasonication in distilled water, followed by acetone. The N-GQDs sample was drop-cast over the cleaned GCE with care and then allowed to dry for 24 h at room temperature (RT) to achieve the modified electrodes. The coating thickness was optimized by trial and error by varying the drop-casting volumes from 20–100 μM.
Further, for proof of the N-GQD–Cd interactions, various characterizations were carried out. The procedure for the preparation of the sample for the characterizations is as described. The N-GQDs were mixed with CdCl2 solution and the product formed was washed well and dried.
The real environment samples spiked with Cd(II) were prepared using one groundwater sample, two seawater samples from two different regions, as well as one wastewater sample. First, blank readings corresponding to each of the samples were carried out so that the current response, if any, was subtracted from each result to obtain the values (corrected for the blank). The corresponding recovery values as well as the RSD were calculated.
2.5. Electrochemical methods
The EC behaviors of various metal ions (Hg(II), Ag(I), Cd(II), Ni(II), Zn(II), Co(II), and Pb(II)) on the surface of the N-GQD/GCE were investigated using cyclic voltammetry (CV), differential pulse voltammetry (DPV), and chronoamperometry at RT. All the EC measurements were carried out on a potentiostat/galvanostat PG 302N, AUT 83909 system (Metrohm, Autolab, Netherlands) with a three-electrode system using the modified GCE (3 mm diameter) as the working electrode, platinum wire as the counter electrode, and Ag(s)/AgCl(s)/Cl−1 (aq.) (saturated KCl) as the reference electrode. First, 20 μL of N-GQDs solution, after 2 times dilution, was drop-cast on to a GCE to obtain the working electrodes, and 0.1 M PBS was used as the electrolyte for all the studies.
The pre-reduction studies were carried out by applying the reduction potential of −0.95 V prior to the oxidation sweep. Then the oxidation and reduction half cycles were carried out.
3. Results and discussions
3.1. Synthesis and characterization of the N-GQDs
The N-GQDs were synthesized using an earlier method reported by our group. The significance of this synthesis approach is the aromatic nature of the precursor PANI and the presence of in situ N, which facilitates the formation of N-doped GQDs without the need for additional doping agents to yield aromatic and crystalline N-GQDs. The structural benefits of N-GQDs as an electrode material were due to their improved conducting properties and as they can act as a good reducing agent. A schematic of the synthesis of the N-GQDs is given in Fig. 1A. The obtained N-GQDs were characterized using various techniques.
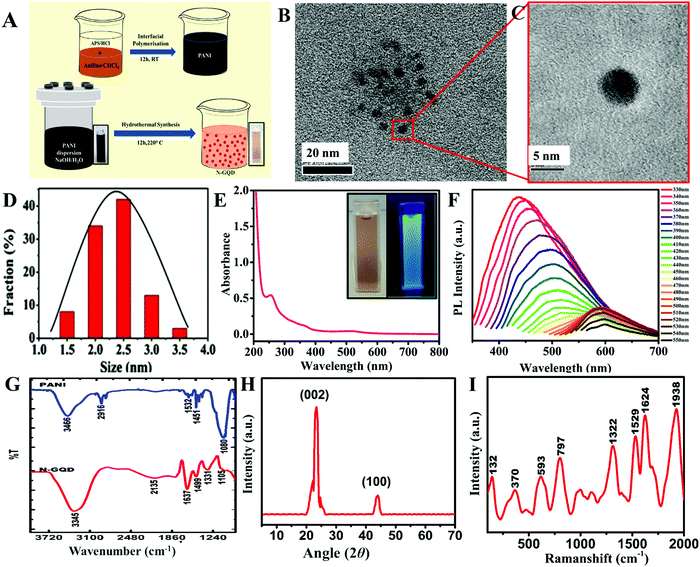 |
| Fig. 1 (A) Schematic of the synthesis of the N-GQDs along with digital images of the aqueous dispersion/solution of PANI and the N-GQDs; (B) and (C) HR-TEM images of the synthesized N-GQD; (D) size distribution of the synthesized N-GQD as obtained from HR-TEM; (E) UV-vis spectrum of the N-GQDs (inset: (E) digital images of the PL of the N-GQDs under normal light and under UV (365 nm) irradiation); (F) dependence of the PL spectrum of the N-GQDs on the excitation wavelength from 300 to 560 nm; (G) FTIR spectra of the N-GQDs and PANI; (H) XRD diffractogram and (I) Raman spectrum of the N-GQDs. | |
HR-TEM images of the as-synthesized N-GQDs confirmed that they had a fairly uniform and narrow size distribution (Fig. 1B and C), ranging from 2 to 3 nm (Fig. 1D). The formation of N-GQDs was further confirmed through their absorption and emission characteristics, which were in good agreement with the previous reports.35–37 The peak at ∼260 nm in the UV-vis spectrum of the N-GQDs (Fig. 1E) could be ascribed to the π–π* transition of the aromatic sp2 domains.38 The typical absorption peaks of graphene derivatives are in the range of 200–300 nm.39 The effective N-doping in the N-GQDs could be verified by the peak around 510 nm due to the n–π* transition. The photoluminescence characterization revealed that the N-GQDs exhibited an excitation-dependent emission (Fig. 1F). The quantum confinement effect (the size effect) is one of the most widely accepted mechanisms for the excitation-dependent emission that is common in carbon-based nanomaterials sized ≤5 nm, and occurs because the smaller size limits the formation of conjugated aromatic domains (isolated sp2 clusters) that are responsible for excitation-independent emission. The size of the N-GQDs obtained from the HR-TEM images were ≤4 nm, and were in the range of 1.5–3.5 nm (Fig. S1, ESI†), which supports the observed phenomenon of excitation-dependent emission. The combination of the non-formation of isolated sp2 clusters and the small size led to a bigger HOMO to LUMO energy gap and the size distribution caused broadened emission wavelengths in the range of 430–650 nm,66 resulting in the observed excitation-dependent emission. The strongest peak around 450 nm corresponded to the excitation at 340 nm, with a Stokes shift of 110 nm, and was responsible for the blue emission observed. The rapid decrease in the emission intensity with the increase in the excitation wavelength is a characteristic emission behavior of N-GQDs (luminescent carbon nanoparticles), and was in good agreement with the previous reports.37,40,41,56,58 The bluish emission confirmed the oxygen-rich functionalities in the N-GQDs.23 These results confirmed the formation and the structure of the N-GQDs.
The FTIR spectra of the N-GQDs and PANI (Fig. 1G) further confirmed the formation of the N-GQDs. The peaks at 3466 and 2916 cm−1 in the spectrum of PANI corresponded to N–H and C–H stretching, respectively. The peak at 1532 cm−1 corresponded to C–C stretching of the benzenoid ring. The C
C stretching was noted at 1451 cm−1 and the C–N stretching was at 1080 cm−1. In the N-GQDs, a broad band at 3345 cm−1 could be assigned to the combined effect of N–H stretching vibrations along with the –O–H stretching and the aromatic –C–H stretching. The peak around 2135 cm−1 indicated the presence of C
N functionality. The peak around 1637 cm−1 suggested the presence of an amide functionality. The peak around 1331 cm−1 corresponded to the C–N stretching vibrations. The peak around 1499 cm−1 is indicative of sp2 bonded C–N. Thus the results further confirmed the formation of N-rich N-GQDs. The XRD pattern of N-GQDs consisted of sharp peaks corresponding to the crystalline nature of the N-GQDs. The 2θ = 23.3° and 43° corresponded to the (002) and (100) graphitic plane reflections (Fig. 1H). The Raman spectra of the N-GQDs consisted of two peaks at 1322 and 1529 cm−1 that were assigned to the D and G bands that are well known for GQDs. The peaks at 1003 and 1108 cm−1 were attributed to the C–C and C
C functional group vibrations, while the peaks at 1624 and 1938 cm−1 corresponded to C–O/C
O vibrations (Fig. 1I).57,59
3.2. EC sensing studies of N-GQDs toward heavy metal ions
The preliminary EC sensing property of N-GQD/GCE was studied using the toxic heavy metal ions Cd(II), Hg(II), Ag(I), Pb(II), Fe(II), Co(II), Ni(II), Zn(II), Sn(II), Cu(II), and Cr(VI). It was observed that the N-GQD/GCE exhibited a current response for Cd(II) alone and no peak was observed for any of the other metal ions. Therefore, further the sensing of Cd(II) by N-GQDs was studied in detail. The CVs obtained by varying the concentrations of N-GQDs from 20 to 100 μL are given in Fig. S2 (ESI†). It can be seen that an optimum result was obtained for the concentration of 20 μL of N-GQDs, with a better CV curve with a comparatively lower redox peak separation. Further increases in the concentration of N-GQD (40 to 100 μL) resulted in a higher separation between the redox peaks, and the reduction peak shifted to a higher potential value. Hence, a 20 μL concentration of N-GQDs was utilized for the modification of the GCE for the further studies. The CV profiles for the EC sensing of 1 mM Cd(II) on the bare GCE, N-GQD/GCE, and GQD/GCE (Fig. 2A) showed that the current responsea on N-GQD/GCE were ∼25, 7, and 6 times higher than those on the bare GCE, PANI/GCE, and GQD/GCE, respectively, which shows the superior EC sensing of Cd(II) by the N-GQDs. The enhancement in the current response was possibly due to the synergistic effect of the increased conductivity of the N-GQDs due to the graphene structure (sp2 hexagonal skeleton) and the hetero N-atom doping, which enhanced the conductivity and the electrocatalytic property. The oxidation peak in the anodic sweep suggested that Cd(II) was in the reduced form Cd(0) and this was attributed to the reducing capability of N-GQDs to give Cd(0). The synthesized N-GQDs contain an aromatic basal plane enriched with N atoms, in the form of graphitic-N, pyrrolic-N, and pyridinic-N, and in addition, they have side functionalities, such as –NH2, –OH, –COOH, and –CONH2. Most of the nitrogen functionalities can act as reducing agents since they contain a lone pair of electrons that can be donated to Cd(II). On the other hand, the C–Cd linkages formed can be resonance stabilized by the donated lone pair on N. The positive charges created by the donation of electrons are highly resonance stabilized by the electron-releasing groups in the aromatic skeleton of the N-GQDs. Thus the electron-rich environment of N-GQDs can act as a good reducing environment for Cd(II), thus spontaneously reducing Cd(II) to Cd(0) on the N-GQDs. Further, by varying the concentration of Cd(II) to higher concentrations (2 to 10 mM), the current response became saturated (Fig. S3, ESI†). This observation backs the spontaneous reduction of Cd(II) on the N-GQDs. These observations were in good agreement with the results obtained from FTIR, Raman, and XPS studies. This is the reason why an oxidation peak was observed in the anodic sweep without a pre-concentration step.
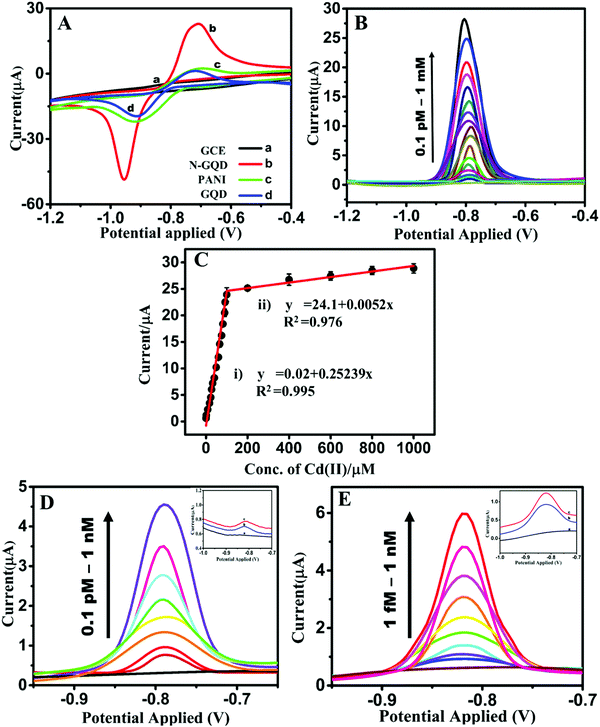 |
| Fig. 2 (A) CV for 1 mM Cd(II) in 0.1 M PBS on (a) bare GCE, (b) N-GQD/GCE, (c) PANI/GCE, and (d) GQD/GCE at the scan rate of 50 mV s−1. (B) DPV responses of Cd(II) on N-GQD/GCE from 10−13 to 10−3 M. (C) LDRs obtained for the sensing of Cd(II) on the N-GQD/GCE for the concentration range of 10−11 to 10−3 M (0.001 to 112 000 ppb). (D and E) DPV responses of Cd(II) on N-GQD/GCE for the concentration range of 10−13 to 10−9 M; and that with pre-reduction from 10−15 to 10−9 M, respectively; (insets D and E: DPV response without pre-reduction and with pre-reduction in 0.1 M PBS: (a) absence of Cd(II) and (b and c) in the presence of 0.1 and 1 pM Cd(II), respectively; and the DPV response of the N-GQD/GCE in 0.1 M PBS (a) in the absence and (b and c) in the presence of 1 and 10 fM Cd(II), respectively). | |
The effect of the solution pH on the electrochemical sensing of Cd(II) on the N-GQDs was studied using both CV and DPV in the range of 2–12. Fig. S4 (ESI†) shows that there was a gradual increase in the peak current with a decrease in the acidity (pH 2–6) and became maximum at neutral pH (7), which again gradually decreased with the increase in the basicity of the pH (8–12). This can be explained with respect to the protonation–deprotonation properties of Cd(II). From pH 2–6, N in the N-GQDs acquires a higher Lewis base nature, thus the interaction between Cd(II) and the N in N-GQDs is affected since Cd(II) has a soft acid character and so it interacts with soft bases more. The Cd(II) has the tendency to form cadmium hydroxy species [(Cd(OH)+)], which has a tendency to preferentially adsorb over the Cd(II) ion.34 At higher pH values, the formation of (Cd(OH)+) species is more feasible. Hence at higher pH, the effective concentration of Cd(II) in the electrolyte became less, and thus lower current responses were obtained.
3.3. Sensitivity, LDR, and LOD
In order to investigate the efficiency of the N-GQDs as an electrode for the quantitative sensing of Cd(II), the current response with the increasing concentration was examined by varying the concentration of Cd(II) from 10−13 to 10−3 M. The result (Fig. 2B) showed that the current responses increased with the increase in the concentration of Cd(II). The LDR obtained for Cd(II) sensing is depicted in Fig. 2C. The concentration ranges 10−11 to 10−3 M (0.00001 to 1000 μM) fitted into two linear ranges. The concentration range of 10−11 to 10−4 M (0.00001 to 100 μM) fell into the first linear range with a correlation coefficient (R2) of 0.995, while the second linear range corresponded to the concentration range 2 × 10−4 to 10−3 M (200 to 1000 μM) with an R2 of 0.976. The corresponding linear regression equations can be expressed as the oxidation peak current [Ipa (μA)] = 0.2524x (μM) + 0.0201 (R2 = 0.9950) and Ipa (μA) = 0.0052x (μM) + 24.1013 (R2 = 0.9760), respectively. The sensitivity values (calculations in the ESI†) corresponding to these LDR values were 3.570 and 0.074 μA μM−1 cm−2, respectively. The calculated LOD (calculation in the ESI†) from the lowest concentration range of the LDR was 7.12 × 10−12 M, i.e., 0.0008 ppb (S/N = 3); whereas the experimental LOD obtained for Cd(II) on the N-GQDs was 10−13 M, i.e., 0.00001 ppb (inset of Fig. 2D). The values obtained were without the pre-reduction step and further, and so the LOD was measured after pre-reduction and the N-GQDs exhibited an LOD of 10−15 M, which corresponded to 0.0000001 ppb, i.e., 0.01 ppq (inset of Fig. 2E). Further, in order to validate the LOD and the corresponding current response, the concentration was gradually increased from 10−13 to 10−9 M and 10−15 to 10−9 M for the studies without pre-reduction and with pre-reduction, respectively, and the result (Fig. 2D and E) evidenced the reproducibility of the current response. The corresponding increase in the current response with pre-reduction was ∼20%.
Table 1 compares the analytical performances of the N-GQD/GCE with the various reported EC Cd(II) sensors based on the LOD values and the LDRs. The Cd(II) sensing studies were carried out with either stripping voltammetric techniques42,59–61,63 or without a pre-concentration step.7,19,43–45,62 SnO2 QDs were reported to have an LOD of 500 ppb, with a corresponding linear concentration range in the 10
000–45
000 ppb concentration range. The fabricated sensor exhibited a sensitivity of 77.5 × 102 nA ppm−1 cm−2.7 A phytic acid-functionalized polypyrrole/graphene oxide-based sensor exhibited an LOD of 2.1 ppb with a linear range of 5–150 ppb.43 A metal–organic framework Cd(II) sensor was reported with linear detection in the concentration range of 0.5–600 ppb and an LOD of 0.3 ppb,19 while a MWCNT/poly (pyrocatechol violet)-modified electrode with an LOD of 0.2 ppb exhibited a linear range of 1–300 ppb,44 and a glutathione-functionalized magnetic nanocomposite showed an LOD of 0.17 ppb with an LDR of 0.5–100 ppb.45 Thus it is clear that the LOD value of 0.00001 ppb by our N-GQDs was the lowest and was 3–4 magnitudes lower than the previously reported values. The LDR ranges obtained covered a wide range starting from nM to mM (0.00001 to 1000 μM, i.e., 0.001–112
000 ppb). The fabricated sensor exhibited a good sensitivity of 3.570 μA μM−1 cm−2. Table 1 shows that the performance of the N-GQD/GCE was better in terms of the sensing property and detection limit compared to that of the previous Cd(II) sensor reports, with the lowest LOD and a better LDR.
Table 1 Comparison of the LOD of N-GQD/GCE with that given in the literature reports of various EC Cd(II) sensors
Materials/electrodes |
With/without pre-reduction |
LOD (ppb) |
LDR (ppb) |
Ref. |
NA – not available. |
Glutathione-functionalized magnetic nanocomposite (GSH@Fe3O4) |
With pre reduction |
0.172 |
0.5–100 |
45
|
Without pre reduction |
NA |
— |
N-Doped carbon quantum dots–graphene oxide hybrid (NCQDs–GO) |
With pre reduction |
7.45 |
5.62–5620 |
42
|
Without pre reduction |
NA |
— |
SnO2 quantum dots |
With pre reduction |
NA |
— |
7
|
Without pre reduction |
500 |
10 000–45 000 |
MOF |
With pre reduction |
0.3 |
0.5–600 |
19
|
Without pre reduction |
NA |
|
Reduced graphene oxide (rGO) and carbon nanotube (CNT) composite |
With pre reduction |
0.6 |
20–200 |
46
|
Without pre reduction |
NA |
— |
MWCNT/poly (pyrocatechol violet) (PCV) |
With pre reduction |
0.2 |
1–300 |
44
|
Without pre reduction |
NA |
— |
Phytic acid-functionalized polypyrrole/graphene oxide (PA/PPy/GO) |
With pre reduction |
NA |
— |
43
|
Without pre reduction |
2.1 |
5–150 |
Chemically modified glassy carbon spheres |
With pre reduction |
22.48 |
112.4–1124 |
63
|
Without pre reduction |
N |
|
Thiomorpholine-functionalized nanoporous mesopore |
With pre reduction |
67.4 |
112.4–1 124 000 |
60
|
Without pre reduction |
NA |
|
Poly(4-vinylpyridine-co-aniline) (poly(4VP-co-Ani))-based solid-state ion sensor |
With pre reduction |
NA |
|
62
|
Without pre reduction |
89.2 |
112.4–11 240 000 |
Amino-calixarene-derivatized graphitic carbon electrode |
With pre reduction |
0.0003 |
0.0011–0.0134 |
59
|
Without pre reduction |
NA |
|
N-GQD |
With pre reduction |
0.0000001 |
|
Our result |
Without pre reduction |
0.000011 |
0.001–112 000 |
The simplicity of the material and the excellent sensing and selectivity of the N-GQDs make them a potential material for practical applications.
3.4. Evidence for Cd(II) and N-GQDs interaction
In order to understand the interaction between N-GQDs and Cd(II), the N-GQDs were mixed with CdCl2 solution and the product formed was washed and dried. The N-GQD–Cd was analyzed by FTIR, Raman spectroscopy, XRD, and XPS. The FTIR spectra of the N-GQD–Cd and N-GQDs are shown in Fig. 3A. The FTIR spectrum of N-GQD–Cd had an additional peak from that of the N-GQDs, specifically at around 1741 cm−1, which was attributed to the metal–carbon stretching frequency. Cd(II) may bond more toward the heteroatom-linked C bonds due to its comparatively electron-rich nature. There was also an observed shift of wavenumbers toward the lower regions.20,48 The peak shift toward the lower wavenumber side implied that the mass of that bond increased. That observation supports the interaction of Cd(II) on N-GQDs and confirmed the bonding of atoms with Cd. The peak corresponding to the –C
O stretching frequency in the N-GQDs at 1637 cm−1 was shifted to 1567 cm−1 in N-GQD–Cd, possibly due to the Cd linkage to the carbonyl carbon. The C–C stretching vibrations of pyridine were noted by the band at 1499 cm−1 in the N-GQDs and were shifted to 1399 cm−1 in N-GQD-Cd due to the bonding between the pyridinic C and Cd. The reduction in the intensity of the C
N peak at 2135 cm−1 in the FTIR spectrum of N-GQD–Cd suggested the interaction of Cd(II) with the N-GQDs through these linkages. However, the peaks corresponding to the N-GQDs functionalities were retained in the N-GQD–Cd spectrum, indicating the stability of the N-GQDs. There were similar observations in the Raman spectrum of N-GQD–Cd (Fig. 3B) as well, with two additional peaks corresponding to Cd–C and N–Cd linkages observed at ∼1820 and 900 cm−1 compared to that of the N-GQDs, in addition to the peak corresponding to the N-GQDs. The XRD pattern (Fig. 3C) of N-GQD–Cd retained the peaks of N-GQDs at around 2θ = 23° and 43°, corresponding to the (002) and (100) graphitic planes with d-spacings of 3.85 and 2.09 Å, respectively.49,50 In addition, N-GQD–Cd showed peaks at around 2θ = 10° and 15°, corresponding to the d-spacings of 8.75 and 5.89 Å, respectively, which could be assigned to the (110) and (202) planes of the N-GQD–Cd complex.51 These observations were in good agreement with the SAED analysis data (Fig. S5, ESI†). While comparing the XRD diffractograms of the N-GQDs and N-GQD–Cd, it is clear that the intensity and position of the graphitic peaks remained the same for the N-GQDs and N-GQD–Cd, suggesting the structure and crystallinity of N-GQDs were unaltered after the addition of Cd(II). Thus, these results indicated the interaction between N-GQDs and Cd(II) through N and also the hetero functional C in the aromatic basal plane. The XPS survey spectrum further confirmed the above observations. The survey scan spectrum of the N-GQDs (Fig. 3D) revealed the C 1s peak at ∼284.5 eV, O 1s peak at ∼530.5 eV, and N 1s peak at ∼400.5 eV. Along with these peaks in the survey scan spectrum of N-GQD–Cd in Fig. 3E, a pronounced Cd 3d peak at ∼411.8 eV was observed.52,53 The high-resolution XPS spectra of N 1s in the N-GQDs indicated the presence of pyridinic (∼398.7 eV), pyrrolic (∼400.2 eV), graphitic (∼400.7 eV), and oxidized pyridinic (∼402.3 eV) N species (Fig. 3G), while that of the C 1s spectrum of the N-GQDs revealed the presence of C–C/C
C (284.7 eV), C–O (286.4 eV), C
O (287.9 eV) O–C
O (290 eV), C–N (∼285.8 eV), and C
N (287 eV) (Fig. 3F) functional groups.23 Whereas in the high-resolution XPS spectra of C 1s as well as the N 1s of N-GQD–Cd (Fig. 3I and J), the peaks corresponding to the C–N, C
N, C–O, O–C
O, pyridinic-N, and pyrrolic-N could not be observed and there was a huge reduction in the intensity of the peaks obtained, which was possibly due to the formation of new bonds between C and N with Cd. The linkage between the C functionalities (C–O, C
O, etc.) with Cd reduced the intensity of these functionalities, and instead new C–Cd peaks were formed. Similar observations were made in the case of N-functionalities and the N–Cd species were formed, which was clear from the high-resolution XPS spectrum of Cd. These observations agreed with the FTIR and Raman observations. The variations in the peaks’ characteristics suggested the interaction among the Cd, C, and N-functionalities. The doped N thus had a profound capability for Cd binding/sensing as evidenced by the huge differences in the peak characteristics. From the high-resolution C 1s and N 1s XPS spectra of N-GQDs and N-GQD–Cd, a decrease in intensity of the C-hetero (N and O)-based functionalities or groups was evident; whereas the peak corresponding to the basic C
C skeleton remained unchanged. These result indicated the higher interaction of Cd toward the C linked with N or O atoms or directly to the heteroatoms. Thus, the heteroatom doping has a profound effect in the selective sensing of Cd by N-GQDs, whereby the lower response of GQDs to that of N-GQDs supported this observation. The high-resolution Cd 3d spectrum displayed two characteristic peaks of Cd 3d5/2 and Cd 3d3/2, centered at 404.8 and 411.9 eV, respectively, which indicate the Cd(II) states54 (Fig. 3H). The obtained peak positions showed a shift toward lower binding energies (BE) of 404.8 and 411.9 eV from those of 405.1 and 412 eV corresponding to the 3d5/2 and Cd 3d3/2 of the Cd(II) state. The lowering of BE is an indication of a lowering of the oxidation state.1,55 This could be attributed to the spontaneous reduction of Cd(II) to Cd(0) on the surface of the N-GQDs, which in turn confirmed the spontaneous reduction of Cd(II) by the N-GQDs. The enhanced reducing property of the N-GQDs was due to the effective N-doping. Thus, the XPS and FTIR results indicated that the N-doping plays an important role in the sensing properties of the N-GQDs and this was supported by the fact that the N-GQDs showed enhanced sensitivity toward Cd(II) to that of the undoped GQDs. The higher selectivity of N-GQDs toward Cd(II) may possibly have been due to the affinity between Cd(II) and N. The softness/hardness of metal ions is seen as a useful parameter to explain the affinity between metal ions and ligands. It is generally agreed that soft metal acids have higher affinities for soft bases (ligands). Though N-functionalities are considered relatively hard bases, N-containing aromatic heterocyclic as well as N-attached aromatics rings are termed as soft bases due to the resonance of electrons in the aromatic ring. Hence N-based functionalities in the N-GQDs, namely pyridinic, pyrrolic, etc., are soft in nature.62 It was shown that the N-GQDs were enriched with N-based heteroatom functionalities, such as pyridinic and pyrrolic.23 Thus, these pyridinic and pyrrolic groups can have a better interaction with the soft acid Cd(II) through C and N linkages compared to with Pb(II), Co(II), Cu(II), Zn(II), Cr(VI), etc., which have moderate to high hard acid characteristics.64,65 Thus this higher affinity of N-containing heterocyclic aromatic systems toward Cd(II) compared to other (studied) metal ions may be the reason for the selectivity of the N-GQDs toward Cd(II). Thus, the selectivity of N-GQDs toward Cd(II) and not Pb(II) and other similar metal ions should be assigned to the structure, the aromatic nature, and the functionalities of the N-GQDs.
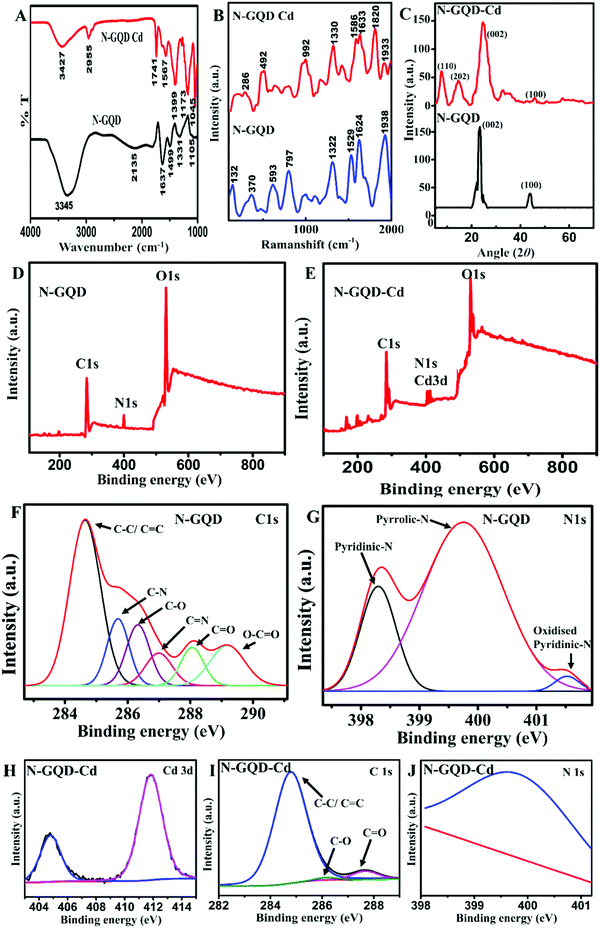 |
| Fig. 3 (A–C) FTIR, Raman, and XRD diffractograms of N-GQDs and N-GQD–Cd, respectively. (D and E) XPS (survey) spectra of N-GQDs and N-GQD–Cd, respectively; (F and G) High-resolution XPS spectra of the C 1s and N 1s of N-GQD. (H–J) The Cd 3d, C 1s, and N 1s high-resolution XPS spectra of N-GQD–Cd. | |
A schematic of the sensing of Cd(II) on N-GQD/GCE and the enhancement in the current response is shown in Scheme 1. As mentioned earlier, the N-GQD/GCE did not require a separate pre-reduction step prior to achieving the oxidation peak of Cd(0) to Cd(II) in the N-GQD/GCE, as the non-bonding electrons mainly from the N-atom (doped) and the aromatic π electrons could reduce Cd(II) to Cd(0) on the N-GQDs. Thus on the surface of N-GQD/GCE, Cd(II) will undergo reduction to Cd(0), which upon the application of an EC potential results in the corresponding redox peaks, as illustrated in the scheme.
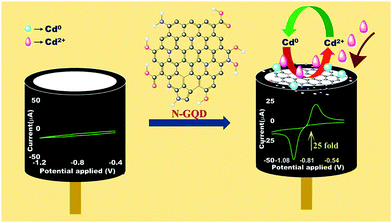 |
| Scheme 1 Schematic diagram showing the modification of the GCE with the N-GQDs and the resulting enhanced sensing of Cd(II) by CV. | |
3.5. Scan rate study
The CV profiles at different scan rates without and with pre-reduction are shown in Fig. 4A and B, respectively. A linear fit of the current response vs. the scan rate corresponded to an adsorption-controlled process, whereas that for the current response vs. the square root of the scan rate corresponded to a diffusion-controlled process.1,47 The studies were carried out by varying the scan rate values from 10 to 1000 mV s−1 both with and without pre-reduction. With pre-reduction, the R2 values were 0.8668 and 0.9368 for the current responses vs. the scan rate, for the anodic and cathodic peak currents, respectively; and the R2 values were 0.9205 and 0.9665 for the current response vs. the square root of the scan rate, for the anodic and cathodic peak currents, respectively (Fig. 4D and F respectively). These results suggest that the system was governed by a diffusion-controlled process as it gave a better fit, with R2 = 0.9205 and 0.9665 for the pre-reduction studies. The shifting of the CV peaks with increasing the Cd(II) concentration was a clear indicator of a diffusion-controlled process (Fig. S6A, ESI†), which was in agreement with the above observation. The corresponding graphs for the studies without and with pre-reduction are presented in Fig. 4.
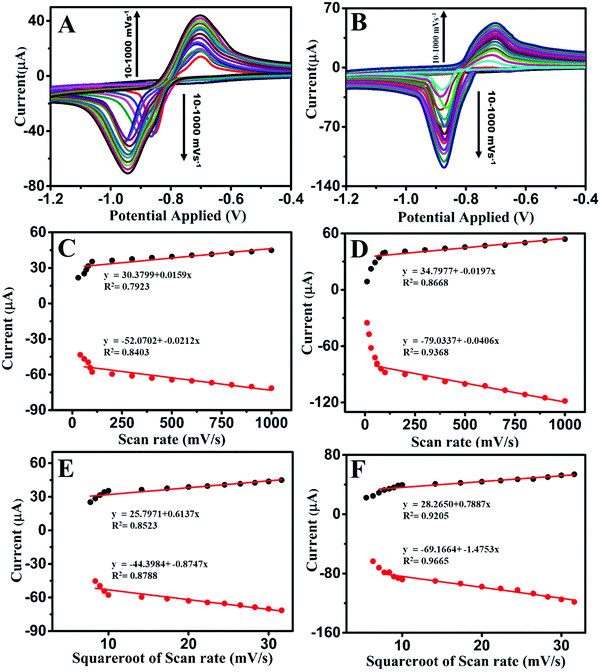 |
| Fig. 4 (A) and (B) CV profiles of the N-GQD/GCE in 0.1 M PBS containing 1 mM Cd(II) at various scan rates (10–1000 mV s−1) without and with pre-reduction respectively. (C) and (D) Calibration plots of the cathodic and anodic peak current vs. the scan rate for without and with pre-reduction respectively. (E) and (F) Calibration plots of the cathodic and anodic peak current vs. the square root of the scan rate for without and with pre-reduction respectively. | |
3.6. Mechanism of the sensing of Cd(II) by the N-GQDs
The enhanced sensitivity of N-GQDs toward Cd(II) could be assigned to the better electrocatalytic activity and electronic conductivity of N-GQD/GCE. As mentioned earlier, the heteroatom doping from the precursor incorporated N atoms into the hexagonal sp2 graphitic aromatic rings without disrupting the aromatic structure. The incorporation of aromatic pyridinic- and pyrrolic-N functionalities in the sp2 hexagonal plane resulted in an increased reactive surface area than that of the GQDs. The pyridinic- and pyrrolic-N functionalities along with the edge hydrophilic group provide an efficient electron cloud that enhances the electronic properties of the N-GQDs as compared to that of GQDs and PANI, which was evident from the multi-fold increase in the current response by the N-GQDs. The N-doping incorporated N into the aromatic lattices without disturbing its honeycomb structure and giving a strong aromatic basal plane enriched with charge carriers. The π-electrons from the graphene structure and the non-bonded electrons from the heteroatom N provide an electron-rich environment for the system. Thus due to the enrichment of electrons in the system as a whole, the HOMO–LUMO band gap in N-GQDs was decreased. As the relationship between the band gap and conductivity are inversely proportional, the conductivity of N-GQDs increased. Thus the higher sensitivity of N-GQDs could be assigned to the better electron-transfer ability and conductivity, which in turn facilitates the electrocatalytic activity of the N-GQDs. The electron-transfer abilities of the N-GQD/GCE, GQD/GCE, and bare GCE were studied by electrochemical impedance spectroscopy (EIS) and the results are given in Fig. S7 (ESI†). From the EIS plots, it can be seen that the diameter of the semicircles were in the order of: bare GCE (66 kΩ) > GQD/GCE (20 kΩ) > N-GQD/GCE (3.81 kΩ), which suggest that the charge-transfer resistance (Rct) was lowest for N-GQD/GCE compared to for GCE and GQD/GCE. The N-GQD/GCE had the lowest Rct compared to that of GQD/GCE and to that of bare GCE. Thus these results indicated the better charge-transfer property of N-GQDs, which was assigned to the comparatively better electron-transfer ability of the N-GQD/GCE.
The selectivity offered by N-GQDs toward Cd(II) was most possibly due to the presence of the N heteroatom functionalities, such as pyridine and pyrrole, in the aromatic plane through doping. In most of the reported heavy metal-ion sensors, a pre-deposition step is conducted to deposit the metal ion in its reduced state on the modified electrode, which then undergoes oxidation when applying an oxidation potential in the CV. However, in this study, the modified electrode, N-GQD/GCE, gave both oxidation and reduction peaks for Cd(II) without any pre-deposition step (with an oxidation sweep being run first). This implies that Cd(II) got reduced spontaneously on the N-GQDs, which indicated that the N-GQDs can act as a reducing agent and can donate electrons to Cd(II) to form Cd(0). The morphological characterizations of N-GQDs and N-GQD-Cd were carried out through SEM analysis (Fig. 5A and B). The SEM elemental mapping results of the N-GQD–Cd and the N-GQDs are shown in Fig. 5C–F and Fig. S8 (ESI†), respectively. The SEM images clearly show the distribution of Cd on the surface of N-GQDs, which indicated the specific interaction of N-GQDs and Cd. This was possibly due to the spontaneous reduction of Cd(II) on the surface of N-GQDs through the N-functionalities. Further, the elemental mapping result (Fig. 5D) clearly showed the presence of Cd.
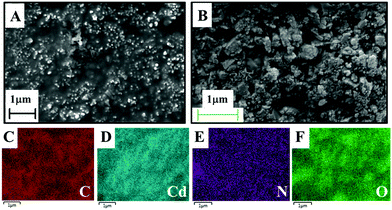 |
| Fig. 5 (A) and (B) SEM images of the synthesized N-GQDs and N-GQD–Cd respectively. (C)–(F) SEM elemental mapping results of N-GQD–Cd revealing the distribution of C, Cd, N, and O. | |
3.7. Selectivity and interference studies
The selectivity and sensitivity are two of the most significant parameters that act as the criteria for ensuring the practical applicability of a sensor. Since N-GQD/GCE exhibited remarkable sensitivity (3.570 μA μM−1 cm−2), exploring its selectivity toward Cd(II) was critical. The CV profiles of Cd(II) in mixtures containing other metal ions (Hg(II), Ag(I), Pb(II), Fe(II), Co(II), Ni(II), Zn(II), Sn(II), Cu(II), and Cr(VI)) at 1× concentrations of Cd(II) (Fig. 6A) showed that N-GQD/GCE was highly selective toward 1 mM Cd(II) in the chosen potential range of −1.2 to −0.4 V and the current response was similar to that obtained in the absence of the metal ions (∼23 ± 0.25 μA for 1 mM Cd(II)). This result is an indication of the ultra-selectivity of the N-GQDs toward Cd(II) in the potential window of −1.2 to −0.4 V and this is portrayed in the bar diagram (Fig. 6B). Further, the results from the interference studies carried out by chrono-amperometric technique at a fixed operational potential of −0.85 V (Fig. 6C) showed nil current responses for the other metal ions and TNP (1000× concentration to that of Cd(II)), where TNP was tested in an earlier work by our group, which showed a sensing property for N-GQDs toward TNP in the reduction scan.23 Inspired by this result, the interference study was repeated at lower concentration of Cd(II), i.e., 10−15 M, and with other species at 10−3 M concentration (1012×) compared to that of Cd(II). The results (Fig. 6D) showed the ability of N-GQDs to sense Cd(II) at ultra-low level concentration of Cd(II) without any interference from the other metal ions, which was shown by the current response only for Cd(II).
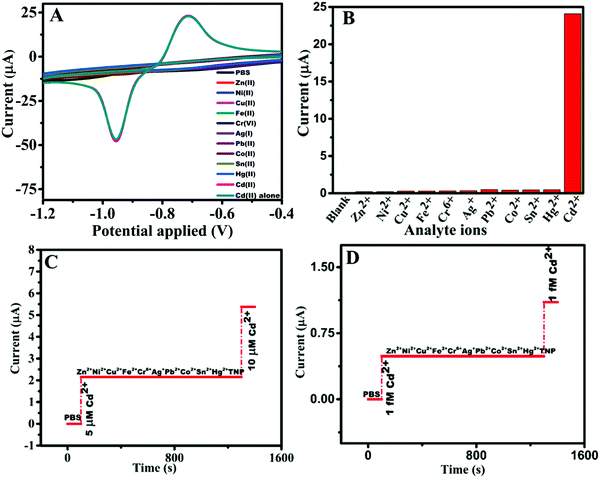 |
| Fig. 6 (A) and (B) Selectivity studies of N-GQD/GCE toward 1 mM Cd(II) in the presence of various analyte species with 1 mM concentration for each. (C) Interference studies of N-GQD/GCE for the detection of 5 and 10 μM Cd(II) in the presence of other metal ions of 1 mM concentration. (D) Interference studies of N-GQD/GCE for the detection of Cd(II) at 1 fM concentration in the presence of other metal ions of 1 mM concentration. | |
3.8. Reusability, reproducibility, and stability studies
In addition to the selectivity and sensitivity, the practical applicability of an electrode depends on its reproducibility, reusability, and stability. The reusability of the N-GQD/GCE electrode was scrutinized by comparing the peak currents for 1 mM Cd(II) on N-GQD/GCE (Fig. S9A, ESI†) before and after multiple washings and the results showed it had ∼96% retention after 3 times of washing. For the reproducibility studies, the current responses of five different electrodes toward 1 mM Cd(II) were examined. The relative standard deviation (RSD) of the peak currents of these individually prepared electrodes was found to be 2.26%, which showed its reproducibility (Fig. S9C, ESI†). The stability studies were conducted after one and three months’ time showed it retained ∼87% and ∼70% of its initial current response, respectively, as depicted in Fig. S9B (ESI†).
3.9. Environmental sample studies
Further, the studies were extended to real environment samples spiked with analytes such as groundwater, seawater from two locations, and wastewater. The recovery values of all the four samples were in the range 100.28–102.23%. The LOD (10−13 M) values obtained in these samples as the electrolyte (Fig. S10, ESI†) were the same as that obtained in PBS. The results thus showed the impressive potential of N-GQD/GCE for sensing Cd(II) in real water samples with no interference and indicated the selectivity of N-GQD/GCE for Cd(II). The recovery information with the real samples is summarized in Table 2. The DPV responses obtained from the real water samples’ analyses are given in Fig. S11 (ESI†).
Table 2 The recovery test results of Cd(II) in environmental samples
Sample no. |
Cd2+ spiked (μM) |
Environmental samples |
Cd2+ found (μM) |
Recovery (%) |
RSD (%) |
1 |
0.5 |
Groundwater |
0.5014 |
100.28 |
0.19 |
Seawater 1 |
0.5041 |
100.82 |
0.57 |
Seawater 2 |
0.5040 |
100.80 |
0.56 |
Wastewater |
0.5067 |
101.34 |
0.94 |
|
2 |
1 |
Groundwater |
1.0084 |
100.84 |
0. 59 |
Seawater 1 |
1.0111 |
101.11 |
0.78 |
Seawater 2 |
1.0140 |
101.40 |
0.98 |
Wastewater |
1.0217 |
102.17 |
1. 51 |
|
3 |
2 |
Groundwater |
2.0104 |
100.52 |
0.36 |
Seawater 1 |
2.0331 |
101. 65 |
1.16 |
Seawater 2 |
2.0360 |
101.80 |
1.26 |
Wastewater |
2.0447 |
102.23 |
1. 56 |
4. Conclusions
In the present work, we successfully demonstrated for the first time, the ultra-high sensitivity and selective EC sensing of Cd(II) by N-GQDs. Interestingly, they could detect Cd(II) up to 1 × 10−5 ppb, which could be drawn-out further to 1 × 10−7 ppb (ppq) by pre-reduction. The effective N-doping, which was attributed to the synthesis procedure, seemed to play a crucial role in the sensing performance of the N-GQDs and allowed spontaneously reducing Cd(II), mostly through the N-sites. Further, N-doping increased the electron availability of the N-GQDs, which in turn increased the ability of the N-GQDs to reduce Cd(II). It was observed that the N-GQD–Cd interactions mainly happened through the heteroatom sites. Thus, this work established a simple as well as environmentally friendly material for the sensing of Cd(II) with remarkable selectivity and sensitivity. A N-GQDs-modified electrode exhibited good reproducibility, reusability, and stability. Moreover the sensor was tested with real samples, such as groundwater, seawater, and wastewater, and showed good sensing performance. These results evidenced the applicability of the N-GQDs as a potential Cd(II) sensor material.
Conflicts of interest
There are no conflicts to declare.
Acknowledgements
The researcher gratefully acknowledges the financial support from the Indian Institute of Space Science and Technology (IIST), Trivandrum extended to the research work.
References
- R. Aswathi and K. Y. Sandhya, J. Mater. Chem. A, 2018, 6, 14602–14613 RSC.
- A. R. Thiruppathi, B. Sidhureddy and K. W. Chen, Electrochem. Commun., 2017, 76, 42–46 CrossRef CAS.
- Y. U. Ruilian, Y. Xing, Z. Yuanhui, H. U. Gongren and T. U. Xianglin, J. Environ. Sci., 2008, 20, 664–669 CrossRef.
- R. K. Sharma, M. Agrawal and F. Marshall, Bull. Environ. Contam. Toxicol., 2006, 77, 312–318 CrossRef CAS.
- F. Length, Int. J. Phys. Sci., 2007, 2, 112–118 Search PubMed.
- E. Chow, D. B. Hibbert and J. J. Gooding, Analyst, 2005, 130, 831–837 RSC.
- G. Bhanjana, N. Dilbaghi, R. Kumar, A. Umar and S. Kumar, Electrochim. Acta, 2015, 169, 97–102 CrossRef CAS.
- X. Chai, L. Zhang and Y. Tian, Anal. Chem., 2014, 86, 10668–10673 CrossRef CAS.
- J. Li, S. Guo, Y. Zhai and E. Wang, Electrochem. Commun., 2009, 11, 1085–1088 CrossRef CAS.
- A. Musa and J. Hamza, Niger. J. Pharm. Sci., 2009, 8, 95–101 Search PubMed.
- S. Saied, E. Zahir and A. Siddique, J. Chem. Soc. Pak., 2010, 32, 737–743 CAS.
- N. M. Hepp, W. R. Mindak and J. Cheng, J. Cosmet. Sci., 2009, 60, 405–414 CAS.
- F. L. M. Rasdi, N. K. Abu Bakar and S. Mohamad, Int. J. Mol. Sci., 2013, 14, 3078–3093 CrossRef CAS.
- A. Ene and A. G. L. Bosneaga, Rom. J. Phys., 2010, 55, 815–820 CAS.
- M. Heidarieh, SpringerPlus, 2013, 2, 1–5 CrossRef.
- D. Pan, Y. Wang, Z. Chen, T. Lou and W. Qin, Anal. Chem., 2009, 81, 5088–5094 CrossRef CAS.
- Z. Wang, H. Wang, Z. Zhang and G. Liu, Sens. Actuators, B, 2014, 199, 7–14 CrossRef CAS.
- A. Zazoua, S. Bouraoui, R. Halim, N. Khedimallah and N. Jaffrezic-Renault, Anal. Lett., 2018, 5, 359–370 CrossRef.
- Y. Wang, J. Mater. Chem. A, 2017, 5, 8385–8393 RSC.
- D. S. Rajawat, N. Kumar and S. P. Satsangee, J. Anal. Sci. Technol., 2014, 5, 1–8 Search PubMed.
- M. N. Arshad, J. Organomet. Chem., 2017, 827, 49–55 CrossRef CAS.
- M. A. E. Mhammedi, J. Hazard. Mater., 2009, 170, 590–594 CrossRef.
- A. Ramachandran, J. S. Arya Nair and S. Karunakaran Yesodha, ACS Sustainable Chem. Eng., 2019, 7, 6732–6743 CrossRef CAS.
- L. Chen, Talanta, 2017, 164, 100–109 CrossRef CAS.
- A. Karimzadeh, M. Hasanzadeh, N. Shadjou and M. Guardia, Trends Anal. Chem., 2018, 105, 484–491 CrossRef CAS.
- Y. Fu, G. Gao and J. Zhi, J. Mater. Chem. B, 2019, 7, 1494–1502 RSC.
- X. Li, M. Rui, J. Song, Z. Shen and H. Zeng, Adv. Funct. Mater., 2015, 25, 4929–4947 CrossRef CAS.
- S. Bak, D. Kim and H. Lee, Curr. Appl. Phys., 2016, 16(9), 1192–1201 CrossRef.
- M. Li, T. Chen, J. J. Gooding and J. Liu, ACS Sens., 2019, 4, 1732–1748 CrossRef CAS.
- O. Aky, F. Karda, M. Beytur, H. Yüksek, N. Atar and M. Lüt, J. Mol. Liq., 2017, 243, 677–681 CrossRef.
- N. Atar, J. Electrochem. Soc., 2017, 164(6), 223–229 CrossRef.
- M. L. Yola and N. Atar, Appl. Surf. Sci., 2018, 458, 648–655 CrossRef CAS.
- M. Kaur, M. Kaur and V. K. Sharma, Adv. Colloid Interface Sci., 2018, 259, 44–64 CrossRef CAS.
- G. Osmond, L. Management, G. Osmond, P. North and N. Zealand, Eur. J. Soil Sci., 1994, 45, 419–429 CrossRef.
- D. Pan, J. Zhang, Z. Li and M. Wu, Adv. Mater., 2010, 22, 734–738 CrossRef CAS.
- K. Habiba, Carbon, 2013, 64, 341–350 CrossRef CAS.
- M. Hassan, Nanoscale, 2014, 6, 11988–11994 RSC.
- D. Qu, Sci. Rep., 2014, 4, 1–11 Search PubMed.
- S. Zhu, Nano Res., 2015, 8, 355–381 CrossRef CAS.
- J. Shen, Y. Zhu, C. Chen, X. Yang and C. Li, Chem. Commun., 2011, 47, 2580–2582 RSC.
- T. Gao, ACS Appl. Mater. Interfaces, 2017, 9, 24846–24856 CrossRef CAS.
- L. Li, D. Liu, A. Shi and T. You, Sens. Actuators, B, 2018, 255, 1762–1770 CrossRef CAS.
- H. Dai, N. Wang, D. Wang, H. Ma and M. Lin, Chem. Eng. J., 2016, 299, 150–155 CrossRef CAS.
- M. A. Chamjangali, H. Kouhestani, F. Masdarolomoor and H. Daneshinejad, Sens. Actuators, B, 2015, 216, 384–393 CrossRef CAS.
- M. Baghayeri, A. Amiri, B. Maleki, Z. Alizadeh and O. Reiser, Sens. Actuators, B, 2018, 273, 1442–1450 CrossRef CAS.
- X. Xuan and J. Y. Park, Sens. Actuators, B, 2018, 255, 1220–1227 CrossRef CAS.
- A. Ramachandran, S. Panda and S. Karunakaran Yesodha, Sens. Actuators, B, 2018, 256, 488–497 CrossRef CAS.
- D. A. Köse and H. Necefoğlu, J. Therm. Anal. Calorim., 2008, 93, 509–514 CrossRef.
- N. Gavrilov, A. I. Pa, M. Mitri and J. Travas-sejdi, J. Power Sources, 2012, 220, 306–316 CrossRef CAS.
- Z. Zhou, W. G. Bouwman, H. Schut and C. Pappas, Carbon, 2014, 69, 17–24 CrossRef CAS.
- K. Thukral, CrystEngComm, 2014, 16, 2802–2809 RSC.
- P. P. Bag, X. Wang, P. Sahoo, J. Xiong and R. Cao, Catal. Sci. Technol., 2017, 7, 5113–5119 RSC.
- M. Huang, J. Mater. Chem. A, 2018, 6, 6015–6021 RSC.
- C. A. Prestidge and S. Grano, Scanning Microsc., 1998, 12, 553–583 Search PubMed.
- B. Zheng, Y. Chen, P. Li, Z. Wang, B. Cao and W. Zhang, Nanophotonics, 2016, 336, 1–9 Search PubMed.
- S. Fasbender, L. Zimmermann, R. Cadeddu, M. Lu, B. Moll, C. Janiak and R. Haas, Sci. Rep., 2019, 1–13 CAS.
- A. B. Ganganboina, A. D. Chowdhury and R. A. Doong, Microchim. Acta, 2018, 185, 1–11 CrossRef CAS.
- J. Wu, P. Wang, F. Wang and Y. Fang, Nanomaterials, 2018, 8, 864 CrossRef.
- P. S. Adarakatti and P. Malingappa, J. Solid State Electrochem., 2016, 20, 3349–3358 CrossRef CAS.
- M. R. Ganjali and M. Asgari, J. Solid State Electrochem., 2010, 14, 1359–1366 CrossRef CAS.
- S. Intarakamhang, W. Schuhmann and A. Schulte, J. Solid State Electrochem., 2013, 17, 1535–1542 CrossRef CAS.
- J. Lim, W. Ling and S. A. Ghani, J. Solid State Electrochem., 2013, 17, 681–690 CrossRef.
- G. K. Raghu, J. Solid State Electrochem., 2012, 16, 1953–1963 CrossRef CAS.
- L. Chen, G. Qu, X. Sun, S. Zhang, L. Wang, N. Sang, D. Yuguo, J. Liu and S. Liu, PLoS One, 2013, 8(3), 1–8 Search PubMed.
- K. B. Jacobson and J. E. Turner, Toxicology, 1980, 16, 1–3 CrossRef CAS.
- Z. Gan, H. Xu and Y. Hao, Nanoscale, 2016, 8, 7794–7807 RSC.
Footnote |
† Electronic supplementary information (ESI) available. See DOI: 10.1039/d0nj03988h |
|
This journal is © The Royal Society of Chemistry and the Centre National de la Recherche Scientifique 2021 |
Click here to see how this site uses Cookies. View our privacy policy here.