DOI:
10.1039/D1NA00283J
(Paper)
Nanoscale Adv., 2021,
3, 4834-4842
Cryo-milled nano-DAP for enhanced growth of monocot and dicot plants†
Received
17th April 2021
, Accepted 19th June 2021
First published on 21st June 2021
Abstract
Phosphorus (P) is a limiting macronutrient that regulates plant growth and development based on the bioavailability of its inorganic form, i.e., orthophosphate (Pi). P plays a critical role in cell development, and it is a key component of ATP, DNA, lipids, and cell signaling machinery. Without the exogenous application of P fertilizers, the yield of crops will not meet the ever-growing demand in today's world. However, due to the non-renewable nature of natural P reserves and simultaneous rapid human population growth, food crops must be ultimately produced more than ever by using a lower P fertilizer input. Hence, the strategy of preparing nano-fertilizers was conceptualized and demonstrated with great success. For example, nano-diammonium phosphate (n-DAP) performed far better than the commercial granular DAP (c-DAP). However, nano-fertilizers, including n-DAP, cannot be produced on a large scale using the available processing methods. Herein, a novel processing strategy, namely cryo-milling, is demonstrated to prepare n-DAP on a kg-scale without altering DAP's bonding structure. Cryo-milling involves milling at liquid N2 temperatures and therefore helps in brittle fracture of coarser DAP particles into n-DAP particles. Cryo-milled n-DAP, with particle size ∼5000 times smaller but specific surface area ∼14
000 times greater than that of c-DAP, enhanced the growth of monocot (wheat) and dicot (tomato) plants due to improved bioavailability of Pi even for a far lower input than c-DAP. Phenotypic observations such as higher leaf biomass, longer shoots, shorter roots, and less anthocyanin pigmentation manifested the extraordinary efficacy of cryo-milled n-DAP for 75% lower input than c-DAP.
Introduction
Exogenous fertilizers, especially limiting nutrients such as phosphorus (P), have been identified as one of the critical measures to sustain food security globally.1 Plants secure their nutrition by absorbing the water-soluble inorganic phosphorus (Pi), the bioavailable form of P, through their roots. Pi's role in plant metabolism encompasses enzyme catalysis, signal transduction, energy transfer, macromolecular biosynthesis, etc. In contrast to some other mineral nutrients, Pi is highly immobile in the soil and often limits the agricultural output.2,3 Therefore, any deficiency in Pi's bioavailability forces superfluous consumption of P fertilizers,4 which are mainly produced from non-renewable rock phosphate reserves. Moreover, the production of P fertilizers not only results in enormous quantities of radioactive phosphogypsum but also cadmium (Cd) contaminated final products.5–7 Therefore, if the current production and use of P fertilizers are continued, the major caveats would be (i) rapid depletion of non-renewable global rock phosphate reserves,8 (ii) irreparable soil health which is detrimental for sustainable agriculture, and (iii) extreme environmental pollution.
Limited P availability is detrimental to plant health, and unfortunately, P is deficient in ∼55% of the global cropland soils.9 Moreover, the major drawback associated with commercial P fertilizers is their poor bio-availability.3 A large amount (up to 80%) of the total agricultural P input is wasted due to its fixation in the soil in non-bioavailable inorganic and organic forms and runoff, making only a fraction of it available for absorption by plant roots for nutrition purposes.10 Therefore, modern and effective approaches are required regarding mineral fertilization in cropping systems.11–13 The poor bioavailability of solid P fertilizers is mainly attributed to the large size (and therefore shallow specific surface area) of the constituent particles. Consequently, the concept of nano-fertilizers (NFs) was conceived and applied because the reduced particle size in NFs enormously increases the specific surface area of the constituent particles and thereby enhances the fertilizer's absorption efficiency by roots even for a far lower fertilizer input. In this context, excellent results on both micro-14–16 and macro-nutrients17,18 (as NFs) have been published. NF incited growth-promoting effects in various food crops.19,20 It is observed from the published results that NFs are typically made by using wet chemical methods, which are limited to lab-scale production and involve harmful chemicals. In contrast, mechanical milling, a physical process, could be touted as an efficient and alternative method of preparing NFs from fertilizers containing coarse particles. Here lies the motivation behind this work, which is not only to demonstrate a novel, easy, effective, and environmentally benign physical method, namely cryo-milling, to prepare nano-diammonium phosphate (n-DAP) from commercial DAP (c-DAP) but also to elucidate the enhanced efficacy of n-DAP on the growth of monocot (wheat) and dicot (tomato) plants even for a far lower input than c-DAP.
Experimental section
Processing and basic characterization of n-DAP
A Union Process (Model 1S-USA) cryo-mill was used to prepare n-DAP from c-DAP (Gromor, Coromandel Fertilizers, India), with an average particle size of ∼2 mm (Fig. 1). 1 kg c-DAP was processed in the milling jar with the aid of stainless steel (SS) balls (5 kg in weight). After filling the milling jar with c-DAP and SS balls, the jar was fixed in its designated slot on the mill. Then the jar was pre-cooled using liquid N2 and the milling was carried out at a temperature of 86 kelvin for 3 h at a milling speed of 300 rpm. The obtained n-DAP was characterized with respect to its morphology, surface composition, particle size distribution, and specific surface area using a Field Emission Scanning Electron Microscope (FESEM) (Ultra55 Carl Zeiss) operated at 5 kV and Fourier Transform Infrared Spectroscopy (FTIR) (Bruker Tensor) using broad IR radiation in reflection geometry, the Dynamic Light Scattering (DLS) technique (Nano sizer nano-s, Malvern), and Brunauer–Emmett–Teller (BET) method using N2 adsorption (Gemini model (11-2370) surface analyzer, Micromeritics).
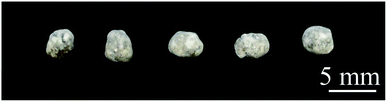 |
| Fig. 1 Photograph of commercial diammonium phosphate (c-DAP) granules. Bar depicts 5 mm. | |
Plant growth experimentation
Seeds of Triticum aestivum (wheat) of Solanum lycopersicum cv. Arka Vikas (tomato) were germinated on doubled distilled (dd) water moistened blotting paper under skotomorphogenic conditions. Further details of germination and media composition are given in ESI Fig. S1 and Table S1.† On the 4th day of seed germination, seedlings with similar-sized radicles were transferred to each pot and then allowed to grow under controlled conditions. Standard protocols were followed in supplementing both solid and liquid growth media. During all the above experiments, the positions of pots were continuously changed to avoid any position effect.
In the ‘supplementation by mixing’ method, graded quantities [(10% to 100% of the field recommended dosage, 100% = 68.255 mg DAP in this study)]7 of P fertilizers (c-DAP and n-DAP), as given in Table S2,† were directly mixed with the solid media. In the ‘pot bottom’ method, different doses of P fertilizers (as given in Table S2†) were first dissolved in 250 mL of manually prepared ½× P free modified Murashige and Skoog (MS) media in separate reagent bottles. In the ‘foliar spray’ method, different doses of the fertilizers (Table S2†) were first dissolved in 20 mL of dd water and then sprayed every 3rd day, starting from the day of seedling transfer, during the plant growth experimentation. In these methods, either 50 mL solution of manually prepared ½× P free media or solution with graded doses (in the pot bottom method) was supplied from the pot base every 3rd day for two weeks for both wheat and tomato seedlings. Positive (68.255 mg of KH2PO4) and negative control (no P input, i.e., 0 P) pots were also prepared for comparison purposes.
In hydroponics experiments, 1 L manually prepared P free ½× modified MS media was transferred to each pot. Then different amounts (25 μM to 200 μM, Table S3†) of P-fertilizer were mixed in the respectively labelled pots. A self-made Styrofoam floater containing fifteen holes ∼1.5 cm in diameter lined below with a plastic wire mesh of ∼2 mm wide holes was placed tightly over the media in each pot. In each hole, a germinated wheat seedling was placed with the roots dipped in the liquid growth media below and allowed to grow. Cleaned clay balls were placed in the holes to prevent algal growth in the media. The setup was aerated for 10 seconds twice a day for proper redistribution of the nutrients. On every 3rd day, the pots were replenished with manually prepared P free ½× modified MS media to maintain the 1 L mark during the experimentation.
To generate statistics and to check reproducibility, all experiments were conducted in duplicate with 15 seedlings per pot and repeated at least twice. During the experimentation, both wheat and tomato seedlings were exposed daily to 16 h of light and 8 h of darkness at 24 °C. Luminance in the plant culture room was maintained at 150–200 μmol m−2 s−1 along with 60–65% relative humidity. Growing seedlings were closely observed for the plant growth rate and the onset of any phenotypic changes.
Morphological assays
To estimate the physical parameters of plants, seedlings were scanned along with a reference of 1 cm using a laser printer. The scanned images were then analyzed for the shoot or/and root lengths using ImageJ software.21 Similarly, the surface area of the first true leaf of tomato seedlings and the surface area of the shoot of wheat seedlings were determined. To determine the fresh weight, each shoot and the respective root of freshly harvested seedlings were separately weighed.
Biochemical and physiological assays
The estimation of total soluble Pi,22–24 anthocyanin25,26 and total carbohydrate27–29 for both shoots and/or roots, separately, was performed in accordance with the standardized published protocols for each assay. In tomato, 500 mg fresh tissue from phenotypically distinguished yet same-age-old seedlings for each test type was subjected to total soluble Pi content assay. Similarly, fresh leaves and/or roots of wheat seedlings with a total weight of 500 mg were utilized for the total soluble Pi assay. Anthocyanin estimation for both tomato and wheat was performed from shoot samples with 250 mg of fresh tissue for each treatment. Studies on elevated sugar content in plants under low Pi have been reported.30 Under such conditions of Pi deprivation, an increase in phloem loading of sugar from the shoot to the root occurs in Arabidopsis.31 Thus, for both wheat and tomato seedlings, the total carbohydrate content assay was performed using 100 mg of the fresh shoot and root tissues. Each assay was performed at least in triplicate and replicated twice. The photosynthetic efficiency was measured after subjecting seedlings to 20 min of dark adaptation using a JUNIOR-PAM fluorometer (Walz, Germany). F0 (initial) and Fm (maximal) fluorescence was measured from fully developed leaves of at least ten seedlings that were grown for 14 days. The resulting Fv/Fm value was determined by WinControl 3.26 software.
Statistical analysis
The recorded data in all experiments were subjected to a two-way ANOVA test (Sidak's multiple comparisons) using GraphPad Prism version 8.0 and converted into statistically significant bar graphs. All experiments were repeated at least twice, unless otherwise specified.
Results and discussion
Different characteristics of n-DAP
Cryo-milling involves high energy milling of powders at liquid N2 temperature, which embrittles the powder particles and thereby facilitates easy brittle fracture of coarse c-DAP particles into n-DAP particles, plausibly without altering the bonding structure in DAP. Using this method, we successfully prepared 1 kg of n-DAP in a single milling process step. In comparison to the larger size of conventional DAP (Fig. 1), the FESEM image of n-DAP (i.e., after cryo-milling) clearly shows that the particle size has considerably decreased (Fig. 2). However, from the FESEM image, no particular shape could be attributed to the n-DAP particles. Also, the n-DAP particles appear to have agglomerated, and therefore to quantify the reduced particle size, DLS was carried out. DLS data showed that the average particle size of n-DAP is ∼378 nm, which is ∼5000 times smaller than that of c-DAP particles (Fig. 1 and S2†). Furthermore, a BET specific surface area of 24.62 m2 g−1 (which is ∼14
000 times greater than that of c-DAP) was measured for n-DAP manifesting the reduction in particle size after cryo-milling. The reduced particle size and increased specific surface area make n-DAP physically more available than c-DAP to any interacting surrounding media.
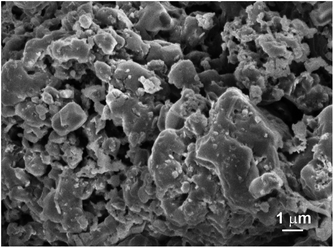 |
| Fig. 2 Nano-diammonium phosphate (n-DAP) as seen using a Field Emission Scanning Electron Microscope (FESEM). Bar represents 1 μm. | |
FTIR spectra of c-DAP and n-DAP were also compared to examine the chemical stability of n-DAP (Table 1).32,33 From the spectra, the characteristic infra-red (IR) bands of PO43−, OH, NH4+ and P–NH are easily discernible (Fig. S3†). More importantly, there is little change in the IR band positions after cryo-milling, indicating that composition-wise there is negligible change in the case of n-DAP.
Table 1 Comparison of the different infra-red (IR) band positions in c-DAP and n-DAP
IR band |
c-DAP (cm−1) |
n-DAP (cm−1) |
PO43− |
522.8 |
525.1 |
551 |
552 |
948.2 |
950.1 |
1044.1 |
1047.9 |
OH |
1193.4 |
1193.8 |
894.5 |
897 |
P–NH |
3174.9 |
3181.5 |
NH4+ |
2999.5 |
3000.6 |
1451 |
1453 |
1714 |
1713 |
Plant growth characteristics
Tomato seedling growth in solid media (supplemented by mixing).
The shoots of n-DAP treated seedlings were found to be significantly longer than their respective c-DAP counterparts at each concentration, with a maximum increase of 34.57% recorded at 25% (quarter-strength) dosage of supplementation (Fig. 3A and B). The supplementation of n-DAP at half-strength (50%) or higher dosage produced seedlings with at least 51.48% heavier shoots than the full-strength c-DAP supplemented seedlings (Fig. 3C). We, however, could not salvage the intact roots from the perlite mix; hence a root morphological comparison could not be performed. We also noticed that the first true leaf on n-DAP treated seedlings had larger surface area over their respective c-DAP counterparts. Even quarter-strength n-DAP treated seedlings had 13.11% more surface area than the full-strength c-DAP treated seedlings (Fig. S4A†). Furthermore, we observed that n-DAP supplemented seedlings, starting from the quarter-strength dosage, had ∼9% higher Fv/Fm values over the full-strength c-DAP controls (Fig. 3D). The shoot samples of 25% n-DAP grown seedlings even had 12.28% higher total soluble Pi content than the 100% c-DAP treated seedlings. Similarly, the Pi content of 50% n-DAP seedlings had a tremendous 93.38% increase over their c-DAP counterparts (Fig. 3E). Similarly, both quarter-strength and half-strength n-DAP supplementation resulted in 23.47% and 27.63% higher root total soluble Pi over their respective c-DAP supplemented counterparts (Fig. 3F). We next tested the inverse effect of Pi content and anthocyanin accumulation in shoot tissues.34 The n-DAP supplemented seedlings accumulated at least 28.28% lower anthocyanin than their respective c-DAP treated counterparts (Fig. 3G). Furthermore, the shoot total carbohydrate content in n-DAP treated seedlings was significantly lower than their respective c-DAP controls (Fig. S4B†). However, such difference in total carbohydrate content of the roots for the two sets of seedlings remained statistically insignificant (Fig. S4C†).
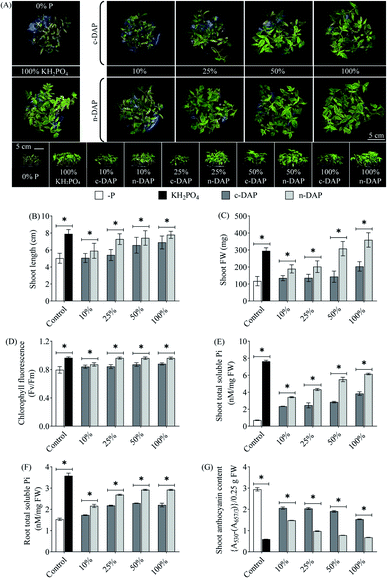 |
| Fig. 3 (A) Phenotypes of 15 day-old tomato seedlings grown in sand and perlite mixture with the supplementation of n-DAP and c-DAP at a dosage regime of 10%, 25%, 50%, and 100% recommended dosage of P. Changes in (B) shoot length, (C) shoot fresh weight, (D) chlorophyll fluorescence (14 DAT), (E) shoot total soluble Pi content, (F) root total soluble Pi content, and (G) shoot anthocyanin content of c-DAP and n-DAP supplemented tomato seedlings with respect to the controls are represented in graphs. Error bars indicate standard deviation [(n ≥ 30 for B and C), (n ≥ 10 for D), (n ≥ 15 for E–G)] and bar colours indicate the type of P used for the treatment. | |
These observations collectively indicated that augmentation of n-DAP even at quarter-strength (25%) field recommended dosage resulted in equivalent effectiveness, if not better, when compared to the tomato seedlings supplemented with the full-strength (100%) c-DAP. It is known that early soil P fertilization, especially when done in the proximity of seeds, helps to overcome the low soil P availability and promotes early seedling growth.35 The more uniform distribution of n-DAP over the granular c-DAP in the solid growth mixture plausibly resulted in an increased physical availability of Pi per unit area for growing tomato roots. Such uniform availability of n-DAP in the media led to enhanced Pi uptake by roots from the rhizosphere, which in turn helped these seedlings grow faster by accumulating more Pi than their c-DAP counterparts in the present study.17,36
Tomato seedling growth in solid media (exogenous supplementation).
To determine and optimize the best method of n-DAP application, we further tested two other supplementation methods, namely the ‘pot bottom supplementation’ method and ‘foliar spray’ method. For this we conducted the assays only at quarter-strength, and full-strength field recommended doses. This is because equivalent or better performance was observed when tomato seedlings were grown by mixing with at least 25% n-DAP dosage as compared to those supplemented with 100% dose of c-DAP (Fig. 3). In the ‘pot bottom supplementation’ method, we observed near stagnancy in the length among the n-DAP and c-DAP supplemented seedlings (Fig. 4A and B). The n-DAP treatment resulted in marginally heavier fresh shoots yet lacked statistical significance (Fig. 4C). Unlike the previously observed results from the ‘supplementation by mixing’ method (Fig. 3), in both foliar spray and pot bottom supplementation methods, the total soluble Pi contents in the n-DAP supplemented shoots barely outperformed their c-DAP counterparts at the quarter-strength dosage. In these two additional methods, the shoot Pi levels remained significantly lower (Fig. 4D) than the levels observed in the tomato seedlings grown with the ‘supplementation by mixing’ method (Fig. 3E). Nonetheless, in comparison to the ‘foliar spray’ method, the ‘pot bottom supplementation’ method had higher total soluble Pi content of shoot (Fig. 4D). The anthocyanin contents of n-DAP shoots under both conditions were lower than those of their c-DAP counterparts. Nonetheless, among the two methods, the ‘pot bottom’ supply treatment shoots resulted in lower anthocyanin content over the ‘foliar spray’ treated shoots (Fig. 4E).
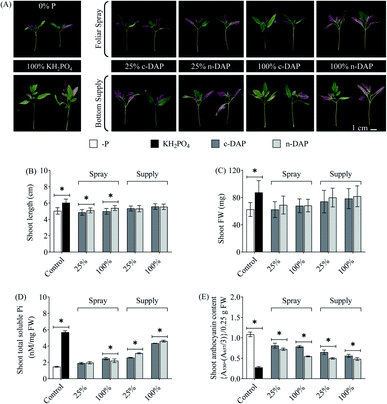 |
| Fig. 4 (A) Phenotypes of two-week-old tomato seedlings grown in a sand and perlite mixture with the supplementation of n-DAP and c-DAP at a dosage regime of 25% and 100% recommended dosage of P via foliar spray and pot bottom supply. Changes in (B) shoot length, (C) shoot fresh weight, (D) shoot total soluble Pi content, and (E) shoot anthocyanin content of c-DAP and n-DAP supplemented tomato seedlings on 14 DAT with respect to the controls are represented in graphs. Error bars indicate standard deviation (n ≥ 20) and bar colours indicate the type of P used for the treatment. | |
Altogether, the plant morphological changes of n-DAP against their c-DAP counterparts remained insignificant in these additionally tested methods. One of the reasons for this observation could be the overall lower Pi uptake when seedlings were grown using these two methods in comparison to the enhanced Pi acquisition efficiency observed in their counterparts grown with the ‘supplementation by mixing’ method. However, based on the tested biochemical markers, n-DAP supplemented seedlings (pot bottom supplementation method) scored over their c-DAP counterparts. Such differences might be explained by the plausible time interval between fertilizer supplementation and actual Pi availability to plants. The ‘pot bottom supplementation’ method requires a prolonged period for the solubilized n-DAP/c-DAP to reach the plant roots through the solid growth media compared to P being mixed with the growth media, as in the first tested (supplementation by mixing) method. Furthermore, the rate and efficiency of the source to sink transport of Pi by foliar spray would be lower than that supplied to the root. This may be because leaf cells reportedly express Pht2;1 for leaf Pi loading, which is a low-affinity Pi transporter.37 In contrast, high-affinity Pi transporters, the primary facilitator of Pi uptake in plants, are preferably localised in the roots.38 This might explain the relatively higher shoot total soluble Pi content of ‘pot bottom supplemented’ seedlings over ‘foliar sprayed’ seedlings. Among the three tested supplementation approaches, the first method (by mixing) clearly scored over the remaining two procedures for the application of n-DAP. The better performance of tomato seedlings in this method could be directly linked to the uniform availability of Pi in the close proximity of roots. Such an easy access to the unlimited Pi would result in enhanced Pi uptake efficiency, as evidenced by higher total soluble Pi accumulation in the tested tissues, leading to better growth and higher performance of those seedlings.
Wheat seedling growth in solid media (supplemented by mixing).
Next, we investigated the efficacy of n-DAP over c-DAP in a monocotyledonous species, wheat. Similar to tomato, wheat seedlings supplemented with n-DAP, except for the quarter-strength dosage, for 14 days, resulted in longer shoots than their c-DAP counterparts (Fig. 5B). The fresh weight of n-DAP wheat shoots marginally increased over their c-DAP counterparts but lacked statistical significance (Fig. S5A†). Similarly, the n-DAP supplementation produced seedlings with significantly shorter roots when compared to their respective c-DAP supplemented counterparts (Fig. 5C). Due to the relatively larger difference observed in the root length, as opposed to the shoot length, between n-DAP and c-DAP treated seedlings, we observed more root weight differences, than that of shoot weight, in wheat seedlings. n-DAP supplemented seedlings had reduced root weight (by at least 12.59%) over their c-DAP counterparts (Fig. 5D). For the chlorophyll fluorescence, near static Fv/Fm values were obtained in all wheat seedlings supplemented with either c-DAP or n-DAP at all graded concentrations (Fig. S5B†). Nevertheless, n-DAP supplementation produced shoots with a maximum of 21.68% (at half-strength) more surface area than the respective c-DAP counterparts (Fig. S5C†).
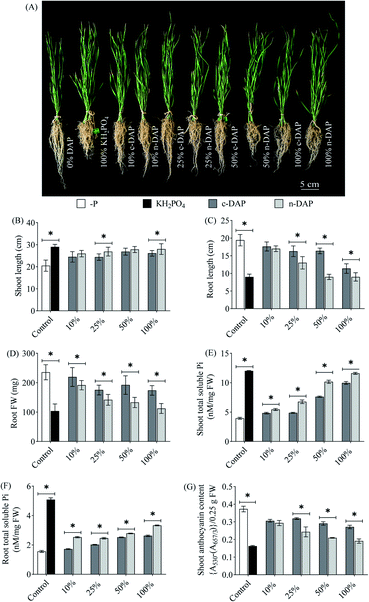 |
| Fig. 5 (A) Phenotypes of two-week-old wheat seedlings grown in a sand and perlite mixture with the supplementation of n-DAP and c-DAP at a dosage regime of 10%, 25%, 50% and 100% recommended dosage of P. Changes in the (B) shoot length, (C) root length, (D) root fresh weight, (E) shoot total soluble Pi content, (F) root total soluble Pi content, and (G) shoot anthocyanin content upon supplementation of c-DAP and n-DAP to growing tomato seedlings on 14 DAT with respect to the controls are given in graphs. Error bars indicate standard deviation [(n ≥ 30 for B–D), (n ≥ 15 for E–G)] and bar colours indicate the type of P used for the treatment. | |
The subsequent biochemical assays showed that n-DAP grown seedlings accumulated higher total soluble Pi content in shoots than their c-DAP counterparts. The shoots of 50% n-DAP treated seedlings had equivalent Pi content to those supplemented with 100% c-DAP (Fig. 5E). Similarly, n-DAP supplemented seedlings had at least 10.5% higher root Pi content over their c-DAP counterparts (Fig. 5E and F). Apart from the lowest tested concentration (i.e., 10%), n-DAP treated seedlings also exhibited a significant decrease in shoot anthocyanin content, at least 10.33% lower in both quarter-strength and half-strength n-DAP seedlings over the full-strength c-DAP control seedlings (Fig. 5G). This indicates plausible better fitness of the n-DAP supplemented seedlings as they are better prepared to combat any oxidative stress as compared to their c-DAP controls. In both shoot and root samples, we further recorded statistically significant reduction in the total carbohydrate content in n-DAP treated seedlings over their c-DAP counterparts, albeit only at the full-strength doses (Fig. S5D and E†).39
The observations made in Fig. 5, mainly the biochemical aspects, indicated the superiority of n-DAP over c-DAP. The half-strength n-DAP grown wheat seedlings performed on par with the full-strength c-DAP supplied seedlings. The variation in the degree of responses such as increase in the size of seedlings and Pi accumulation between wheat and tomato seedlings might be explained by the differences in the size of mature wheat and tomato seeds. Additionally, it can be attributed to their different growth dynamics. It is known that seed phosphorus content affects plant growth.40 Mature wheat seeds possess prominent endosperm that stores a large quantity of inorganic phosphate as phytate.41 Relying on this endospermic stored Pi might help the seedlings to grow with minimal influence from external Pi sources during the juvenile stages. This might explain the relatively smaller differences in wheat seedlings' shoot morphology when graded doses of n-DAP and c-DAP were supplemented. Tomato produces smaller seeds which have minimal endospermic tissue contributing to much less nutrients, thereby plausibly forcing the growing seedlings to rely more on external nutrients for the growth and development. Therefore, such anatomical differences potentially resulted in a more sensitive phenotypic response to the availability of external Pi per unit area in the rhizosphere of tomato seedlings while the wheat seedlings became relatively less susceptible to such changes. The presence of a profuse rooting system of wheat seedlings, as opposed to that of tomato seedlings, can be another trait for such a difference in the results obtained with the two species when subjected to similar growth conditions.
Wheat seedling growth in liquid media (hydroponics).
Due to the lack of a relatively more robust growth response by wheat seedlings in solid growth media with n-DAP, we further tested their growth under a hydroponics system (Fig. 6A). Morphologically, the wheat seedlings did not show any significant difference in their shoot length over their c-DAP supplemented counterparts apart from the full-strength dose, at which a significant increase of 13.73% shoot length was recorded in n-DAP grown seedlings (Fig. S6A†). Only at the full-strength dosage did n-DAP seedlings produce significantly heavier fresh shoots, by 46.42%, than their c-DAP counterparts (Fig. 6B). Like the shoot, the root lengths of n-DAP treated seedlings, except at full-strength dosage, were nearly equivalent to those of their respective c-DAP counterparts (Fig. S6B†). Although the root fresh weight of n-DAP grown seedlings gradually decreased (beginning at 50 μM strength) with respect to their c-DAP treated counterparts, such incremental changes remained statistically insignificant (Fig. 6C). Likewise, only the full-strength n-DAP supplied seedlings showed a significant increase in their shoot surface area with 38.81% over the full-strength c-DAP supplemented seedlings (Fig. S6C†). The chlorophyll fluorescence (Fv/Fm) also did not vary much and exhibited negligible variation among the n-DAP and c-DAP treated seedlings (Fig. S6D†).
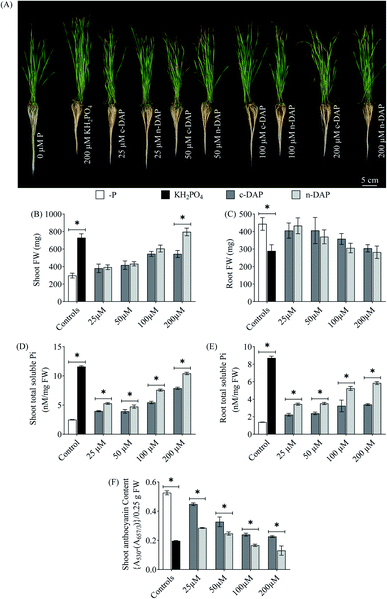 |
| Fig. 6 (A) Phenotypes of two-week-old wheat seedlings grown via hydroponics with the supplementation of n-DAP and c-DAP at a dosage regime of 25 μM, 50 μM, 100 μM and 200 μM. Changes in the (B) shoot fresh weight, (C) root fresh weight, (D) shoot total soluble Pi content, (E) root total soluble Pi content, and (F) shoot anthocyanin content are graphically represented. Error bars indicate standard deviation [(n ≥ 30 for B and C), (n ≥ 15 for D–F)] and bar colours indicate the type of P used for the treatment. | |
In contrast to the morpho-physiological characteristics, the n-DAP treated seedlings outperformed their c-DAP treated counterparts biochemically. It was observed that the shoot of half-strength n-DAP supplemented seedlings not only surpassed its c-DAP counterpart by 39.05% in total soluble Pi content but almost matched that of the full-strength c-DAP treated seedlings. Furthermore, the shoot Pi content of seedlings grown with 200 μM n-DAP surpassed that of the c-DAP counterpart by 32.38% (Fig. 6D). Similarly, seedlings supplemented with even the lowest dose of n-DAP (25 μM) had 54.31% more total soluble Pi content in roots over their c-DAP counterparts (Fig. 6E). Overall, the results demonstrated a significant increment in the nutrient uptake efficiency of n-DAP over the c-DAP in multiple tested methods. A significant decrease of at least 24.46% anthocyanin content was observed in n-DAP seedlings than their c-DAP counterparts (Fig. 6F). Supplementation of n-DAP caused the total carbohydrate content to decline from 200 μM to 25 μM in both shoot and root samples than those supplemented with c-DAP (Fig. S6E and F†).
Observations from the wheat morphological data and chlorophyll fluorescence indicted uncertainty in correlating the increased efficiency of n-DAP over c-DAP. We propose that the increment in the shoot length and fresh weight of the wheat seedlings grown in hydroponics over the ones grown in solid growth media might plausibly be due to the enhanced abundance of Pi and other nutrients in the liquid media per unit surface area per root. The roots of hydroponically grown seedlings have unhindered access to the supplemented P-fertilizers as the growth medium was liquid, while the roots of wheat seedlings grown in the solid growth media had to navigate through the solid media to tap and access the P-fertilizers.
Conclusions
We have successfully developed an industrially viable dry method to generate chemically stable and ∼5000 times smaller n-DAP particles using the cryo-milling process. By testing multiple methods, we even recommend ‘supplementation by mixing’ of n-DAP with soil as the most preferred mode of nano-P fertilizer application. Subsequently, through a series of morphological, physiological, and biochemical assays, we provide evidence on the superior agronomic use efficiency of n-DAP over c-DAP. Improved biomass, pronounced Pi content, and reduced anthocyanin at the quarter-strength (in tomato) and half-strength (in wheat) n-DAP supplemented seedlings support the improved agronomic use efficiency of the developed nano-P fertilizer. Such a surge in plant total soluble Pi content is often associated with better Pi uptake efficiency, in this case primarily from the supplemented n-DAP that outsmarts it granular counterpart for this trait (Fig. 7). Besides the potential better agronomy of n-DAP over c-DAP, the use of n-DAP in reduced quantities while meeting the plants' optimum P nutrient requirement is preferred for better soil health and agricultural sustainability.
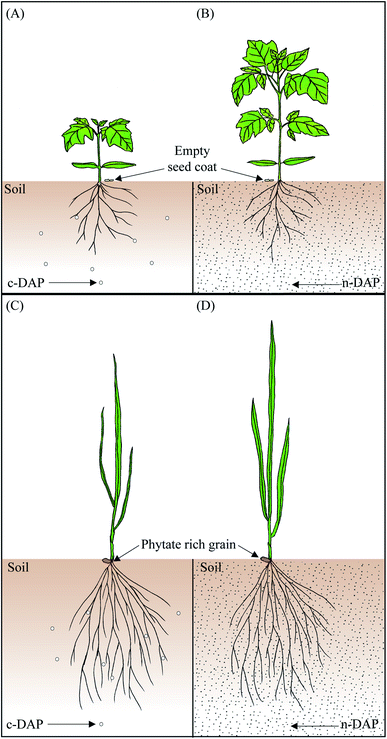 |
| Fig. 7 A plausible model depicting the superiority of n-DAP over c-DAP upon supplementation to growing seedlings in solid growth media. (A and B) c-DAP supplemented to tomato seedlings remain relatively inaccessible to the taproot system. Due to the increased surface area of n-DAP upon supplementation, the P fertilizer accessibility per unit area of the root surface significantly increases, thus, leading to enhanced growth. (C and D) In wheat seedlings, the robust fibrous rooting system can tap more of the sparsely distributed c-DAP than the roots of tomato seedlings. Additionally, mature wheat grains store a large amount of minerals, including a high quantity of Pi in the form of phytate. Since the seed Pi content affects seedling growth, the presence of a phytate-rich grain along with a robust fibrous root system contributes to smaller morphological differences between the c-DAP and n-DAP supplemented wheat seedlings. | |
Author contributions
Rahul Kumar and Vadali Venkata Satya Siva Srikanth conceived the idea of this study. Sreedhara Sudhakara Sarma and Tata Narsinga Rao were involved in material production. The material was characterized by Sreedhara Sudhakara Sarma and Harita Pant. Naorem Ronald Reagan Singh conducted the plant-based material supplementation experiments and analyzed the data. Rahul Kumar and Naorem Ronald Reagan Singh wrote the original manuscript.
Conflicts of interest
There are no conflicts to declare.
Acknowledgements
The authors acknowledge the funding bodies of the lab Department of Science and Technology, Govt. of India (DST; grant no. INT/BLG/P-06/2019), Science and Engineering Research Board (SERB, grant no. CRG_2018_001033), and IoE-UoH by MHRD [grant no. (F11/9/2019-U3(A)) and RC1-20-018]. The support from the funding agencies, namely University Grants Commission (under Special Assistance Programme, UGC-SAP-DRS-II), and DST (under Funds for Infrastructure in Science and Technology, FIST Level II) for the common facilities in the Department of Plant Sciences, University of Hyderabad, is duly acknowledged. Furthermore, Junior Research Fellowship availed to NRRS from CSIR during the tenure of this study is duly acknowledged.
References
- R. K. Varshney, K. C. Bansal, P. K. Aggarwal, S. K. Datta and P. Q. Craufurd, Trends Plant Sci., 2011, 16, 363–371 CrossRef CAS PubMed.
- S. Pourranjbari Saghaiesh, M. K. Souri and M. Moghaddam, J. Plant Nutr., 2019, 42, 178–185 CrossRef CAS.
- M. K. Souri and M. Hatamian, J. Plant Nutr., 2019, 42, 67–78 CrossRef CAS.
- J. M. Mogollón, A. H. W. Beusen, H. J. M. van Grinsven, H. Westhoek and A. F. Bouwman, Global Environmental Change, 2018, 50, 149–163 CrossRef.
-
B. J. Alloway and E. Steinnes, in Cadmium in Soils and Plants, ed. M. J. McLaughlin and B. R. Singh, Springer, Netherlands, 1st edn, 1999, ch. 5, pp. 97–123 Search PubMed.
-
K. Kovler, in Toxicity of Building Materials, ed. F. Pacheco-Torgal, S. Jalali and A. Fucic, Woodhead Publishing, 2012, pp. 196–240 Search PubMed.
- H. Tayibi, M. Choura, F. A. López, F. J. Alguacil and A. López-Delgado, J. Environ. Manage., 2009, 90, 2377–2386 CrossRef CAS PubMed.
- J. Cooper, R. Lombardi, D. Boardman and C. Carliell-Marquet, Resour., Conserv. Recycl., 2011, 57, 78–86 CrossRef.
- G. K. MacDonald, E. M. Bennett, P. A. Potter and N. Ramankutty, Proc. Natl. Acad. Sci. U. S. A., 2011, 108, 3086–3091 CrossRef CAS PubMed.
-
D. Curtin
J. K. Syers and A. E. Johnston, Efficiency of Soil and Fertilizer Phosphorus Use: Reconciling Changing Concepts of Soil Phosphorus Behaviour with Agronomic Information, ed. J. K. Syers, A. E. Johnston and D. Curtin, Food and Agricultural Organization of the United Nations, Rome, 2008, vol. 45, p. 2009 Search PubMed.
- M. Ebrahimi, M. K. Souri, A. Mousavi and N. Sahebani, Chem. Biol. Technol. Agric., 2021, 8, 19 CrossRef CAS.
- F. Zargar Shooshtari, M. K. Souri, M. R. Hasandokht and S. K. Jari, Chem. Biol. Technol. Agric., 2020, 7, 19 CrossRef CAS.
- M. K. Souri and M. Bakhtiarizade, Sci. Hortic., 2019, 243, 472–476 CrossRef CAS.
- I. A. Medina-Velo, A. C. Barrios, N. Zuverza-Mena, J. A. Hernandez-Viezcas, C. H. Chang, Z. Ji, J. I. Zink, J. R. Peralta-Videa and J. L. Gardea-Torresdey, J. Hazard. Mater., 2017, 332, 214–222 CrossRef CAS PubMed.
- P. Deshpande, A. Dapkekar, M. D. Oak, K. M. Paknikar and J. M. Rajwade, Carbohydr. Polym., 2017, 165, 394–401 CrossRef CAS PubMed.
- A. Mukherjee, S. Pokhrel, S. Bandyopadhyay, L. Mädler, J. R. Peralta-Videa and J. L. Gardea-Torresdey, Chem. Eng. J., 2014, 258, 394–401 CrossRef CAS.
- N. Kottegoda, C. Sandaruwan, G. Priyadarshana, A. Siriwardhana, U. A. Rathnayake, D. M. Berugoda Arachchige, A. R. Kumarasinghe, D. Dahanayake, V. Karunaratne and G. A. J. Amaratunga, ACS Nano, 2017, 11, 1214–1221 CrossRef CAS PubMed.
- R. Liu and R. Lal, Sci. Rep., 2014, 4, 5686 CrossRef CAS PubMed.
-
M. H. Siddiqui, M. H. Al-Whaibi and F. Mohammad, in Nanotechnology and Plant Sciences: Nanoparticles and Their Impact on Plants, 2015, pp. 1–303 Search PubMed.
- L. Zheng, F. Hong, S. Lu and C. Liu, Biol. Trace Elem. Res., 2005, 104, 83–92 CrossRef CAS PubMed.
- C. A. Schneider, W. S. Rasband and K. W. Eliceiri, Nat. Methods, 2012, 9, 671–675 CrossRef CAS PubMed.
- B. N. Ames, Methods Enzymol., 1966, 8, 115–118 CAS.
- A. Jain, M. D. Poling, A. S. Karthikeyan, J. J. Blakeslee, W. A. Peer, B. Titapiwatanakun, A. S. Murphy and K. G. Raghothama, Plant Physiol., 2007, 144, 232–247 CrossRef CAS PubMed.
- R. Srivastava, Akash, A. P. Parida, P. K. Chauhan and R. Kumar, Int. J. Biol. Macromol., 2020, 165, 2253–2266 CrossRef CAS PubMed.
- D. A. Sims and J. A. Gamon, Remote Sens. Environ., 2002, 81, 337–354 CrossRef.
- Akash, A. P. Parida, A. Srivastava, S. Mathur, A. K. Sharma and R. Kumar, Plant Physiol. Biochem., 2021, 162, 349–362 CrossRef CAS PubMed.
- P. Nos, HiPer® Carbohydrates Estimation Teaching Kit (Quantitative); product code: HTBC003; number of experiments that can be performed: 10; duration of experiment, DNSA method: 1 hour; the kit is stable for 12 months from the date of manufacture, Storage Instr..
- S. S. Swaroopanand, S. Mahavidyalya, H. Bhilai, N. Agrawal, D. K. Minj and K. Rani, IOSR J. Environ. Sci., Toxicol. Food Technol., 2015, 1, 24–27 Search PubMed.
- V. M. Jain, G. N. Karibasappa, A. S. Dodamani and G. V. Mali, J. Educ. Health Promot., 2017, 6, 90 CrossRef PubMed.
- C. Hermans, J. P. Hammond, P. J. White and N. Verbruggen, Trends Plant Sci., 2006, 11, 610–617 CrossRef CAS PubMed.
- J. P. Hammond and P. J. White, J. Exp. Bot., 2008, 59, 93–109 CrossRef CAS PubMed.
- V. Ramakrishnan and G. Aruldhas, Infrared Phys., 1988, 28, 77–81 CrossRef CAS.
- M. Gargouri, C. Chtara, P. Charrock, A. Nzihou and H. El Feki, Ind. Eng. Chem. Res., 2011, 50, 6580–6584 CrossRef CAS.
- C. Jiang, X. Gao, L. Liao, N. P. Harberd and X. Fu, Plant Physiol., 2007, 145, 1460–1470 CrossRef CAS PubMed.
-
P. de Willigen and M. Van Noordwijk, PhD thesis, Agricultural University Wageningen, Netherlands, 1987, 220.
- P. S. Bindraban, C. O. Dimkpa and R. Pandey, Biol. Fertil. Soils, 2020, 56, 299–317 CrossRef CAS.
- P. Daram, S. Brunner, C. Rausch, C. Steiner, N. Amrhein and M. Bucher, Plant Cell, 1999, 11, 2153–2166 CrossRef CAS PubMed.
- L. Nussaume, S. Kanno, H. Javot, E. Marin, N. Pochon, A. Ayadi, T. M. Nakanishi and M. C. Thibaud, Front. Plant Sci., 2011, 2, 1–12 Search PubMed.
- J. P. Hammond and P. J. White, Plant Physiol., 2011, 156, 1033–1040 CrossRef CAS PubMed.
- Y.-G. Zhu and S. E. Smith, Plant Soil, 2001, 231, 105–112 CrossRef CAS.
- Z. Šramková, E. Gregová and E. Šturdík, Acta Chim. Slovaca, 2009, 2, 115–138 Search PubMed.
Footnote |
† Electronic supplementary information (ESI) available: Details of seed germination (Fig. S1), media composition (Table S1), experimental setup (Tables S2 and S3), DLS and FTIR spectra of n-DAP particles (Fig. S2 and S3) and supporting figures of the assays conducted (Fig. S4–S6). See DOI: 10.1039/d1na00283j |
|
This journal is © The Royal Society of Chemistry 2021 |