DOI:
10.1039/D0NA00846J
(Paper)
Nanoscale Adv., 2021,
3, 463-470
Multifunctional NaYF4:Nd/NaDyF4 nanocrystals as a multimodal platform for NIR-II fluorescence and magnetic resonance imaging†
Received
11th October 2020
, Accepted 1st December 2020
First published on 9th December 2020
Abstract
Recently, multimodal imaging nanoprobes based on the complementary advantages of various imaging methods have attracted considerable attention due to their potential application in the biomedical field. As important bioimaging nanoprobes, lanthanide-doped nanocrystals (NCs) would be expected to improve the related biophotonic technology through integrated multimodal bioimaging. Herein, water-soluble and biocompatible NaYF4:Nd/NaDyF4 NCs were prepared by a solvothermal method combined with hydrophobic interaction with phospholipids as a capping agent. The NaYF4:Nd/NaDyF4 NCs exhibit excellent colloidal stability under physiological conditions. Compared with the bare NaYF4:Nd3+ NCs, the second near-infrared (NIR-II, 1000–1700 nm) fluorescence intensities of Nd3+ ions in the NaYF4:Nd/NaDyF4 core–shell NCs at the emissions of 1058 nm and 1332 nm are enhanced by 3.46- and 1.75-fold, respectively. Moreover, the r2 value of NaYF4:Nd/NaDyF4 NCs as T2-weighted contrast agents is calculated to be 44.0 mM−1 s−1. As a novel multimodal imaging nanoprobe, the NaYF4:Nd/NaDyF4 NCs can be employed for both NIR-II fluorescence and magnetic resonance imaging (MRI). The phospholipid-modified NaYF4:Nd/NaDyF4 NCs demonstrate in vitro and in vivo multimodal NIR-II fluorescence imaging and MRI of HeLa cells and tumors, respectively. This study provides an effective strategy for the development of novel multimodal probes for the medical application of nanomaterials.
Introduction
As a new biomedical imaging approach, nanotechnology has been widely applied in the early detection and diagnosis of diseases. In the past few decades, traditional medical imaging technologies, such as magnetic resonance imaging (MRI), computed tomography, positron emission tomography, and photoacoustics have played important roles in the diagnosis and treatment of diseases.1–4 Owing to the inevitable limitations of each monomodal imaging technology, however, it is difficult to meet the requirements of spatial resolution and sensitivity for modern medical imaging.5 Fortunately, the multimodal imaging approach combined with the respective merits of mono-modal imaging can provide multiple complementary imaging information in the diagnosis of diseases.6–8 So far, many strategies for constructing hybrid nanoparticles (NPs) have been developed by encapsulating or conjugating fluorescent dyes, magnetic nanocrystals (NCs), upconversion NPs, rare earth complexes and radioactive reagents.4,9–11 In our previous work, several nanocomposites or NCs have been developed for multimodal bioimaging nanoprobes, such as poly(DL-lactic-co-glycolic acid) coated NaYF4:Yb, Er@NaGdF4/doxorubicin nanocapsules, Fe3O4 nanoclusters, polyacrylic acid-NaMnF3@doxorubicin hybrid nanocomposites, ultrasmall Mn2+-doped NaNdF4 NCs, ZnPc/NaGdF4:Yb,Er nanoclusters and folic acid-chitosan-coated NaYF4:Yb/Er nanocrystals.12–17 However, to construct desired multimodal imaging nanoprobes with high sensitivity, high spatial resolution and deep tissue penetration is still a major challenge.
Recently, fluorescence imaging has attracted more and more attention in life science and medical fields due to its fast feedback, high sensitivity and high spatial–temporal resolution.15,18–21 In particular, fluorescence imaging in the second near infrared (NIR-II, 1000–1700 nm) window has been widely used in basic scientific research and clinical application due to its advantages of high sensitivity and high spatiotemporal resolution with the increase of tissue penetration depths. Compared with fluorescence imaging in the visible and the first near-infrared (NIR-I, 700–950 nm) regions, NIR-II fluorescence imaging significantly reduces the scattering loss, which makes it possible to observe the deep organisms of living animals with a higher spatial resolution.22 Because the NIR-II window is considered to be one of the optimal regions for optical bioimaging, it is an urgent task to develop fluorescence probes with NIR-II emission capability. Although the existing NIR-II fluorophores are relatively lacking, there currently exist four candidates: quantum dots,23–27 single-walled carbon nanotubes,28–30 organic dye molecules,31,32 and conjugated polymers.3 However, there are some major limitations to the above mentioned fluorophores.19 In contrast, lanthanide (Ln)-based NCs (e.g. Er3+, Tm3+, Ho3+, Pr3+ and Nd3+) as a new generation of NIR-II nanoprobes have many advantages, such as no photobleaching, long photoluminescence lifetimes, low long-term cytotoxicity and narrow emission band widths, which have gained more attention recently for bioimaging applications.19,33 Among the candidates, Nd3+-doped NCs arise as pioneers for NIR-II imaging due to their easy fabrication procedures and non-heating excitation bands in the biological transparency window.19 The Nd3+ ions in NCs with different host matrices are able to emit NIR-II fluorescence under the excitation of near-infrared light. Therefore, the Nd3+ doped NCs have received extensive attention in the past few years.34–39 Previous studies have shown that Nd3+ ions were mainly used as dopants, and the optimal doping molar ratio was controlled between 1% and 5%, as excessive concentrations of Nd3+ ions could cause a quenching effect and reduce the photoluminescence efficiency.33,35,36,40 In addition, surface quenching due to defects or interactions with solvent molecules also affects their optical efficiency. Therefore, to obtain bright fluorescence signals, passivation shells would be employed for fabricating core–shell nanostructures, which shows a beneficial effect and has been proved in many studies.35,36,39,41
As one of the main means of physics and chemistry, MRI is widely used in the field of clinical imaging diagnosis, which relies on longitudinal (T1) and transverse (T2) relaxation times of endogenous water protons of the tissues to produce endogenous contrast. In previous studies, MRI, as a biological imaging method, provides more relative position details than other imaging methods, and can complement the biological information provided by other imaging methods.42–46 Some rare earth elements (i.e., Gd3+ and Dy3+) as MRI contrast agents can be co-doped into upconversion NCs to achieve high tissue penetration depth utilizing multi-modal bioimaging.12,47,48 Among them, Dy3+ ions have the advantages of a short electron relaxation time (∼0.5 ps) and large magnetic moment (10.6 μB) and are considered to be an ideal contrast agent for T2-weighted MRI.49 Dy3+ doped upconversion NCs have been used as efficient T2-weighted contrast agents in ultrahigh field MRI to obtain contrast enhancement.48,50–52 Therefore, Dy3+ doped NCs are the ideal candidates for cancer imaging, which can lead to more accurate diagnosis. In order to be applied in biological environments, surface modification of NCs is necessary to enhance their water-solubility and biocompatibility.53
Significant research efforts have been made in the fabrication of Ln3+-doped multifunctional nanocomposites to bridge the strengths of resolution and depth between MRI and NIR-II optical imaging. Herein, we prepared phospholipid-modified NaYF4:Nd/NaDyF4 (Lipo-NaYF4:Nd/NaDyF4) NCs as multimodal bioimaging nanoprobes for in vitro and in vivo bioimaging of HeLa cells and tumors, respectively. Phospholipids possess a hydrophilic structure with low toxicity, and have been successfully used to modify various inorganic nanomaterials, such as quantum dots, iron, silica, and upconversion NCs.54,55 The luminescent core of NaYF4:Nd exhibited strong downconversion NIR-II luminescence under the excitation of 785 nm. With the growth of the protection NaDyF4 layer on the NaYF4:Nd core, the luminescence intensity at the emissions of 1058 and 1332 nm can be further enhanced by 3.46- and 1.75-fold, respectively, higher than that of bare NaYF4:Nd NCs. Moreover, a high r2 value of 44.0 mM−1 s−1 was calculated for the NaYF4:Nd/NaDyF4 NCs as T2-weighted contrast agents. Therefore, accurate imaging can be obtained by intensified NIR-II luminescence imaging associated with a shell coating and T2-weighted MRI imaging introduced by the Dy element. In addition, phospholipids have been employed as a surface ligand of NaYF4:Nd/NaDyF4 NCs, which not only provides good biocompatibility but also enhances tumor penetration of imaging nanoprobes. Most importantly, the current research demonstrates that NaYF4:Nd/NaDyF4 NCs are potential candidates as a multimodal bioimaging platform to obtain more accurate imaging information and sensitivity.
Results and discussion
The preparation process of Lipo-NaYF4:Nd/NaDyF4 NCs is schematically illustrated in Fig. 1a. First, uniform oleic acid (OA)-capped NaYF4:Nd NCs were prepared using a solvothermal method and could be well dispersed in cyclohexane. Subsequently, the NaYF4:Nd NCs were used as seed particles and coated with a layer of NaDyF4 as the shell to form OA-NaYF4:Nd/NaDyF4 core–shell NCs. In order to be applied in biological environments, the surface modification of the as-obtained NaYF4:Nd/NaDyF4 NCs is required to make them water-soluble and biocompatible. Finally, the OA-NaYF4:Nd@NaDyF4 NCs were modified with phospholipids as the capping ligand, which is considered to be safe and been widely used for encapsulating drugs. The transmission electron microscopy (TEM) images of the OA-NaYF4:Nd, OA-NaYF4:Nd/NaDyF4, and Lipo-NaYF4:Nd/NaDyF4 NCs are shown in Fig. 1. The OA-NaYF4:Nd NCs exhibit good uniformity with an average diameter of 12.6 nm (Fig. 1b), whereas the corresponding OA-NaYF4:Nd/NaDyF4 core–shell NCs have an average diameter of 15.7 nm (Fig. 1d). The thickness of the NaDyF4 shells in the NaYF4:Nd/NaDyF4 core–shell NCs is about 1.5 nm. After surface modification, the amphiphilic PEGylated Lipo-NaYF4:Nd/NaDyF4 NCs displayed excellent water dispersal without any visible aggregation and were monodisperse in size, as shown in the TEM image (Fig. 1f). Furthermore, the high resolution TEM (HRTEM) image shown in Fig. 1c demonstrates that the synthesized OA-NaYF4:Nd NCs possess clear lattice fringes, indicating high crystallinity of the obtained NaYF4:Nd NCs. After coating with NaDyF4 as the shell, the size of the obtained core–shell NCs increased slightly and the crystal lattice is clear, as shown in Fig. 1d and e. For subsequent biological application, the OA-NaYF4:Nd/NaDyF4 NCs were transferred from the organic phase to the aqueous phase using biomimetic phospholipids (Fig. 1a). After modification with the phospholipid, the size of the NCs was almost unchanged. The obtained Lipo-NaYF4:Nd/NaDyF4 core–shell NCs were still monodisperse in size (Fig. 1f and g). When the surface of the OA-NCs is modified with PEGylated phospholipids, the hydrophilic PEG on the surface of the NCs is exposed to water, which generates water dispersible NCs with a biomimetic and biocompatible surface.15 The van der Waals interactions between the OA ligands on the NCs' surface and the two hydrophobic tails of the phospholipids make the Lipo-NaYF4:Nd/NaDyF4 core–shell NCs excellent water dispersal systems. In particular, the Lipo-NaYF4:Nd/NaDyF4 NCs can remain stable in aqueous dispersion for up to six months and in 50% FBS dispersion for at least one month.
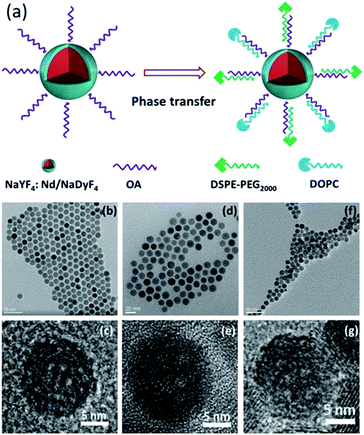 |
| Fig. 1 (a) Schematic illustration of Lipo-NaYF4:Nd/NaDyF4 NCs. Typical and high resolution TEM images of OA-NaYF4:Nd (b and c), OA-NaYF4:Nd/NaDyF4 (d and e) and Lipo-NaYF4:Nd/NaDyF4 (f and g) NCs, respectively. | |
The X-ray diffraction (XRD) patterns of the NaYF4:Nd NCs (Fig. 2) show that all peaks match well with the pure hexagonal NaYF4 phase (JCPDS no. 16-0334) and no impurity phase is observed. The strong and narrow peaks are evidence of the high crystallinity of the NCs, consistent with the results of TEM. It is worth noting that the lattice mismatch between hexagonal-phase NaLnF4 was considered to be one of the key factors for the formation of core–shell NCs.56,57 The patterns of the NaYF4:Nd/NaDyF4 NCs were slightly shifted relative to the standard hexagonal phase pattern of NaDyF4 (JCPDS no. 27-0687), which is also attributed to the lattice mismatch between NaYF4 and NaDyF4. Energy dispersive X-ray spectroscopy (EDS) further confirms the presence of Y, Nd, Na, Dy and F elements in the NaYF4:Nd/NaDyF4 core–shell NCs (Fig. S1 in the ESI†). The Dy element exists in the EDS results of the NaYF4:Nd/NaDyF4 core–shell NCs, indicating the successful coating of NaDyF4 on the NaYF4:Nd NCs.
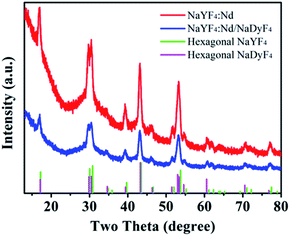 |
| Fig. 2 XRD patterns of the as-synthesized NaYF4:Nd (red) and NaYF4:Nd/NaDyF4 (blue) nanoparticles and corresponding standard curves (green, magenta). | |
The luminescence emission spectra of the obtained NCs were recorded and are shown in Fig. 3. Under excitation with a continuous-wave 785 nm laser, the emission spectra of the OA-NaYF4:Nd NCs and the OA-NaYF4:Nd/NaDyF4 NCs in cyclohexane, and the Lipo-NaYF4:Nd/NaDyF4 NCs in saline display two characteristic downconversion emission peaks at 1058 and 1332 nm (Fig. 3), which are attributed to the transitions of Nd3+ from the 4F3/2 state to the 4I11/2 and 4I13/2 states, respectively. After coating with the NaDyF4 shell layer, the NIR-II fluorescence intensities of Nd3+ ions in the OA-NaYF4:Nd/NaDyF4 NCs at the emission peaks of 1058 nm and 1332 nm are increased to be 3.46- and 1.75-fold higher than that of the bare OA-capped NaYF4:Nd NCs, respectively. In the process of crystal growth, lattice defects are inevitable. There are more defects on the surface of nanomaterials because of the large surface-volume ratio of the samples.58 These defects are usually the centers of fluorescence quenching.59 The outer passivation shell can effectively enhance the luminescence emission intensity of the NCs due to the partial elimination of surface defects in the NCs.60 Similar observations have been reported.39 Additionally, the Lipo-NaYF4:Nd/NaDyF4 NCs could be dispersed in saline solution after surface modification with phospholipids and the intensity of luminescence emission remains strong enough, as shown in Fig. 3. The emission intensity of the Lipo-NaYF4:Nd/NaDyF4 NCs was slightly decreased in the saline phase in comparison with the OA-NaYF4:Nd/NaDyF4 NCs in cyclohexane. These well-defined emission bands are very suitable for deep tissue fluorescence biological imaging, matching well with the NIR-II biological windows.
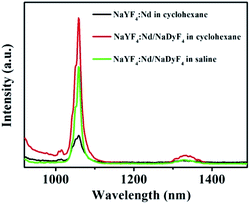 |
| Fig. 3 Luminescence emission spectra of OA-NaYF4:Nd NCs in cyclohexane (black), OA-NaYF4:Nd/NaDyF4 NCs in cyclohexane (red) and Lipo-NaYF4:Nd/NaDyF4 NCs in saline (green) solution under excitation of 785 nm. | |
Inspired by the excellent performance of Lipo-NaYF4:Nd/NaDyF4 NCs, the potential for multimodal imaging would be desired to explore their applications in the in vitro and in vivo bioimaging. The cytotoxicity of Lipo-NaYF4:Nd/NaDyF4 NCs as imaging contrast agents was evaluated against HeLa cells using the methyl thiazolyl tetrazolium (MTT) assay before biological applications. The cell viability of HeLa cells is demonstrated in Fig. 4 after incubation with Lipo-NaYF4:Nd/NaDyF4 NCs with a series of concentrations (0, 50, 100, 150, 200, 250, 300 and 400 μg mL−1) for 12 h at 37 °C. The cell viability still remains above 90% even after incubation with an extremely high concentration (400 μg mL−1) for 12 h. This result proves that the Lipo-NaYF4:Nd/NaDyF4 NCs have excellent biocompatibility within the appropriate concentration range, which is a very significant parameter for further research on biological applications.
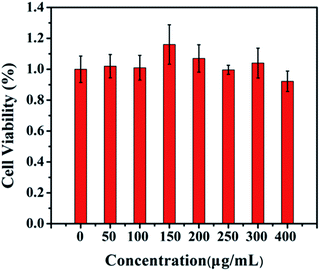 |
| Fig. 4 Cell viability of HeLa cells after incubation with Lipo-NaYF4:Nd/NaDyF4 NCs with different concentrations for 12 h at 37 °C. | |
To verify the NIR-II imaging in cells, the Lipo-NaYF4:Nd/NaDyF4 NCs with different concentrations (0, 1.5, 3.0, 6.0, 12.0, and 24.0 μg mL−1) were transfected into HeLa cells via electroporation. The strong NIR emission of HeLa cell pellets after labeling with Lipo-NaYF4:Nd/NaDyF4 NCs was observed using an InGaAs camera under 808 nm irradiation. As shown in Fig. S2 in the ESI,† the NIR-II emission intensity of the fluorescence images increases with the increased NC concentration, which is attributed to dose-dependent cellular internalization.15 This result shows that Lipo-NaYF4:Nd/NaDyF4 NCs can successfully penetrate the cell membrane and label effectively the HeLa cells.
To further confirm the internalization of Lipo-NaYF4:Nd/NaDyF4 NCs, the images of T2-weighted MRI were collected after the HeLa cells were labeled with different concentrations of Lipo-NaYF4:Nd/NaDyF4 NCs via electroporation. In vitro MRI was then performed on cell pellets packed in capillaries, which was considered a better mimic of in vivo tissues than cells diluted in culture media. MRI reveals more three-dimensional details of relative positions than other imaging methods including optical imaging.61 Due to the short electronic relaxation time and the high magnetic moment, the Dy-doped NCs are considered an appropriate contrast agent for T2-weighted MRI, especially for soft tissues.62 In order to evaluate the MRI capacity of the Lipo-NaYF4:Nd/NaDyF4 NCs, the transverse relativity (r2) was measured first. As shown in Fig. 5a, the T2-weighted MRI displays a strong dependence of signal intensity on the Dy3+ concentration. The r2 value of 44.0 mM−1 s−1 for Lipo-NaYF4:Nd/NaDyF4 NCs was calculated from the slope of the concentration-dependent relaxation rate (1/T2), which was much higher than that of the previous report (7.68 mM−1 s−1 for Dy NCs).47 In the previous report, the r2 value of superparamagnetic iron oxide NPs is about 44.79 mM−1 s−1, whereas commercial Resovist has an r2 value of 151 mM−1 s−1.63 The r2 value is dependent on the size of the nanocrystal clusters.64 In our case, we speculate that compared with the commercial contrast agent, the relatively lower r2 value may be attributed to the smaller size of the Lipo-NaYF4:Nd/NaDyF4 NCs. These results demonstrate the strong negative contrast effect and great potential utility of Lipo-NaYF4:Nd/NaDyF4 NCs for MRI. Therefore, a set of T2-weighted MRI experiments were carried out to investigate the ability of accumulation of Lipo-NaYF4:Nd/NaDyF4 NCs in the HeLa cells after electroporation. Fig. 5b shows in vitro T2-weighted MRI of HeLa cells labeled with Lipo-NaYF4:Nd/NaDyF4 NCs as a function of the added NC concentration. The Lipo-NaYF4:Nd/NaDyF4 solutions became darker in the T2-weighted MRI with increasing the Dy3+ concentration. The T2-weighted MRI demonstrated an obvious signal increase. This result shows that the Lipo-NaYF4:Nd/NaDyF4 NCs can be considered to be an excellent candidate acting as a T2-weighted contrast agent. The obtained Lipo-NaYF4:Nd/NaDyF4 NCs demonstrate not only high r2 transverse relaxation but also excellent NIR-II fluorescence emission, which prevent effectively a common fluorescence quenching that often occurs in the nanocomposite containing iron oxide as T2-weighted contrast agents. Therefore, multifunctional Lipo-NaYF4:Nd/NaDyF4 NCs as a multimodal platform would have potential application in NIR-II fluorescence and MR imaging.
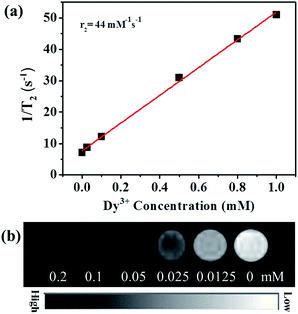 |
| Fig. 5 (a) T2 relaxivity plot of Lipo-NaYF4:Nd/NaDyF4 NCs dispersed in saline. (b) T2-weighted MRI of HeLa cells after being transfected with Lipo-NaYF4:Nd/NaDyF4 NCs using an 11.7 T MR system. | |
Owing to the excellent performances of the Lipo-NaYF4:Nd/NaDyF4 NCs in vitro, the in vivo dual-mode bioimaging was carried out after the Lipo-NaYF4:Nd and Lipo-NaYF4:Nd/NaDyF4 NCs (2.0 mg mL−1) dissolved in saline were injected respectively into the tumors grown on the back of the nude mouse. In order to further evaluate the potential application of Lipo-NaYF4:Nd/NaDyF4 NCs for in vivo imaging, the NIR-II fluorescence imaging of the whole mouse was performed under 808 nm diode laser irradiation (0.12 W cm−2) and the NIR-II fluorescence generated by Nd3+ was recorded using an InGaAs camera in the 900–1700 nm spectral range. The photograph and NIR-II fluorescence image of the whole body of the mouse are shown in Fig. 6a and b, respectively. The strong NIR-II fluorescence signals from the Lipo-NaYF4:Nd/NaDyF4 NCs can be clearly observed in the right tumor (yellow circle) on the back region of the cervical tumor bearing mouse in comparison with the left tumor (yellow circle), as displayed in Fig. 6b. Furthermore, the corresponding in vivo T2-weighted MRI was performed to evaluate the capability of the Lipo-NaYF4:Nd/NaDyF4 NCs as contrast agents. An obviously darker signal was observed in T2-weighted MRI of the right tumor in contrast with the left tumor, as shown in Fig. 6c, where the boundary between the normal soft tissue and the tumor is clearly visible. Thus, the tumor is easily distinguished. More importantly, both the NIR-II fluorescence signal and T2-weighted MRI contrast were enhanced simultaneously in the right tumor injected with the Lipo-NaYF4:Nd/NaDyF4 NCs, which can provide more comprehensive imaging information and lead to higher diagnostic accuracy due to the resolution of MRI location at the sub-millimeter level. Accordingly, self-confirmed multimodal imaging based on MR and NIR-II fluorescence imaging of Lipo-NaYF4:Nd/NaDyF4 NCs would be desired for further accurate next generation diagnosis of tumors.
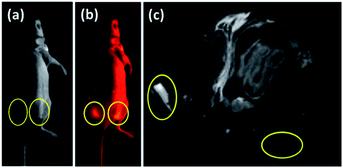 |
| Fig. 6 (a) Photograph of a nude mouse implanted with HeLa cells. The positions of the two tumor regions are marked by yellow circles. The in vivo NIR-II fluorescence (b) and T2-weighted MR imaging (c) of tumor regions after being directly injected with Lipo-NaYF4:Nd (left) and Lipo-NaYF4:Nd/NaDyF4 (right) NCs, respectively. The fluorescence and negative contrast originating from Lipo-NaYF4:Nd/NaDyF4 NCs is clearly observed at the injection site (right circle), while the tumor (left circle) acts as a reference with the injected Lipo-NaYF4:Nd NCs. | |
Experimental section
Chemicals
Yttrium chloride hexahydrate (YCl3·6H2O, 99.99%), neodymium chloride hexahydrate (NdCl3·6H2O, 99.99%) and dysprosium oxide (Dy2O3, 99.99%) were purchased from Jinan Camolai Trading Co., China. OA (90%), 1-octadecene (ODE, 90%) and trifluoroacetic acid (TFA, 99%) were purchased from Aldrich. 1,2-Distearoyl-sn-glycero-3-phosphoethanolamine-N-[methoxy (polyethyleneglycol)-2000] (ammonium salt) (DSPE-PEG2000) and 1,2-dioleoyl-sn-glycero-3-phosphocholine (DOPC) were purchased from Avanti Polar Lipids, Inc. All the chemicals related with this work were of analytical grade and were used without any further purification.
Synthesis of Nd3+-doped NaYF4 NCs
Hexagonal-phase NaYF4: 5% Nd NCs were synthesized following a solvothermal method in our previous work with slight modification.11,12,14,65 In a typical experiment, 18 mg NdCl3·6H2O and 288 mg YCl3·6H2O were added into a 100 mL three-necked flask containing 6 mL OA and 15 mL ODE and then the solution was maintained at 160 °C for 1 h under an argon atmosphere. After being cooled down to room temperature, 10 mL methanol solution of NaOH (100 mg) and NH4F (148 mg) were added and the mixture was stirred at room temperature for 1 h, followed by evaporating water and methanol at 120 °C. After degassing, the solution was heated to 300 °C and kept for 1 h, then cooled down to room temperature and the products were obtained by washing with ethanol several times, and finally re-dispersed in cyclohexane for future use.
Preparation of dysprosium trifluoroacetic acid precursors
The dysprosium trifluoroacetic acid (TFA) precursors were prepared from the corresponding oxides and TFA. Specifically, 0.013 mmol Dy2O3 was mixed with TFA (8 mL) and distilled water (10 mL) in a 50 mL three-neck round-bottom flask. The mixture was kept under magnetic stirring at 80 °C overnight until a transparent solution was formed. After that, the excess TFA and water were slowly evaporated by lowering the temperature to 60 °C. The final solid products were collected and put into a brown bottle and then stored in a desiccator.
Synthesis of OA-capped NaYF4:Nd/NaDyF4 core–shell NCs
A mixture of 0.5 mmol of Dy(CF3COO)3 and 0.5 mmol of NaCF3COO was put into a 50 mL flask containing OA (6 mL) and ODE (15 mL). After stirring at room temperature for 10 min, 4 mL of cyclohexane containing 0.05 mmol of NaYF4:Nd NCs was added to the mixture. Then, the solution mixture was slowly heated to 100 °C and kept for 30 min until the solution became clear. Then, under the protection of an argon atmosphere, water and air in the reaction system were removed. After that, the reaction system was heated to 280 °C and maintained for 1 h. The final OA-NaYF4:Nd/NaDyF4 core–shell NCs were collected by centrifugation at 11
000 rpm and washed with ethanol three times.
Surface modification of NaYF4:Nd/NaDyF4 NCs with phospholipids
Lipo-NaYF4:Nd/NaDyF4 NCs were synthesized according to a published method with minor modifications.15,66,67 DSPE-PEG2000 (40 mg) and DOPC (5 mg) phospholipids were added into 2 mL chloroform. The OA-capped NaYF4:Nd/NaDyF4 NCs in chloroform (0.5 mL, 10 mg mL−1) was mixed with the above chloroform solution (2 mL) in a round-bottom flask (10 mL). The mixed solution was sonicated for 30 min. To evaporate the solvent, the mixed solution was maintained at 60 °C for 60 min under vacuum in a rotary evaporator and a lipid film formed on the inside wall of the flask. Subsequently, the lipid film was hydrated with 1.5 mL of physiological saline. After vigorous sonication, the obtained NaYF4:Nd/NaDyF4 NCs became soluble. The solution was transferred to a microtube and centrifuged gently. The pellet was discarded to remove possible large aggregates. Excess lipids were removed from the Lipo-NaYF4:Nd/NaDyF4 NCs by ultracentrifugation and washing. The obtained Lipo-NaYF4:Nd/NaDyF4 NCs were finally suspended in saline water and stored at 4 °C in a fridge for further experiments. The preparation of Lipo-NaYF4:Nd NCs was carried out by a similar procedure.
Cell culture and MTT assay
HeLa cells were cultured in Dulbecco's Modified Eagle Medium (DMEM) supplemented with 10% fetal bovine serum (FBS) and 1% penicillin/streptomycin at 37 °C in a humidified 5% CO2 atmosphere. The cytotoxicity of Lipo-NaYF4:Nd/NaDyF4 NCs was measured using MTT assay. Cells were seeded into a 96-well culture plate with a density of 104 per well and incubated for 24 h under the same culture conditions as used previously. After that, the medium was washed with phosphate-buffered saline (PBS) and replaced with DMEM solution of Lipo-NaYF4:Nd/NaDyF4 NCs with different concentrations (0, 50, 100, 150, 200, 250, 300, and 400 μg mL−1). The cells were then incubated for 12 h followed by calculating the cell viability with a typical MTT assay.
In vitro NIR-II photoluminescence imaging of Lipo-NaYF4:Nd/NaDyF4 NCs
In our experiment, the electroporation method was used to transfect the Lipo-NaYF4:Nd/NaDyF4 NCs into the cells instead of cultivation for several hours. Specifically, HeLa cells were detached using trypsin and harvested by 1000 rpm centrifugation for 4 min. HeLa cells were resuspended with transfer buffer with different Nd3+ concentrations of Lipo-NaYF4:Nd/NaDyF4 NCs (0, 1.5, 3.0, 6.0, 12.0, and 24.0 μg mL−1). Subsequently, the HeLa cells were loaded into 96-well culture plates. Then, the mixture was treated with electrical pulses provided by the electroporation device (Etta Biotech X-Porator H1), centrifuged and washed with DMEM (only supplemented with 1% penicillin/streptomycin) several times to remove the free Lipo-NaYF4:Nd/NaDyF4 NCs. The NIR-II fluorescence imaging of HeLa cells was performed by excitation using an 808 nm diode laser.
Relaxivity and T2-weighted MRI of Lipo-NaYF4:Nd/NaDyF4 NCs in vitro
Different concentrations of Lipo-NaYF4:Nd/NaDyF4 NCs (10 μL, 0, 0.025, 0.1, 0.5, 0.8, and 1.0 mM) were distributed in capillary tubes. The transversal relaxation times (T2) were measured using an 11.7 T Bruker-Biospin MRI scanner (60 MHz, 21 °C). The relaxation rates (1/T2) were then plotted corresponding to the Dy3+ concentrations, and the relaxivities (r2) were calculated from the slope of the graphs. A series of Lipo-NaYF4:Nd/NaDyF4 NCs in saline solutions (0, 0.0125, 0.025, 0.05, 0.1, and 0.2 mM of the Dy3+ concentration) for relaxivities have also been employed for in vitro cell MRI.
MRI and NIR-II fluorescence imaging in vivo
Nude mice (4 week aged, 20 g) were purchased from SLRC Laboratory Animal Co., Ltd. (Shanghai, China) and kept in the facility with free access to food and water. All animals received humane care in compliance with the Guide for the Care and Use of Laboratory Animals by the National Institute of Health. The study protocol was approved by the Laboratory Animal Ethics Committee. To perform in vivo imaging studies, the nude mice were injected with 5 million HeLa cells for induction of tumors. After 14 days of tumor growth, the Lipo-NaYF4:Nd and Lipo-NaYF4:Nd/NaDyF4 NCs in physiological saline (20 μL, 2 mg mL−1) were injected directly into the tumors and imaged using a T2-weighted relaxation enhancement sequence with TR = 3000 ms, TE = 38 ms, slice thickness = 0.5 mm, and field of view (FOV) = 40 mm and an NIR fluorescence imaging system equipped with an InGaAs/SWIR camera (Photonic Science, UK and an 808 nm long pass filter) and an 808 nm diode laser.
Characterization
The TEM images and EDS spectra were obtained by using an FEI Tecnai G2 F20 S-Twin microscope operating at 200 kV. The power XRD patterns were recorded on a Bruker D8 Advance X-ray diffractometer (Cu Kα, operated at 40 kV, λ = 0.15406 nm) over the 2θ range of 10–80° with a scanning rate of 4° per minute. The NIR fluorescence spectra were recorded using a Model NS1 NanoSpectralyzer at room temperature using an excitation laser source of 785 nm. The collected range was from 900 to 1700 nm. MRI of phantoms was performed on a Bruker AVANCE 500WB spectrometer (Bruker NMR, Germany), with an 89 mm vertical-bore magnet of 11.7 T using a 15-mm-i.d. birdcage coil. T2 relaxation times of different Dy concentrations were measured using a multi-slice multi-echo (MSME) sequence with 20 echoes. The experimental parameters were TR = 3000 ms, TE = 40 ms, FOV = 12 mm × 12 mm, matrix = 96 × 96, slice thickness = 0.8 mm, and an average number = 2. T2 relaxation rates were characterized by using the reciprocal of T2 relaxation times. Transverse relaxivity (r2) in the unit of (mM)−1 s−1 was calculated using curve-fitting of the T2 relaxation rates versus the Dy concentration (mM). The MSME method was used and TR/TE values were set as 500 ms/5.2 ms and 7500 ms/40 ms for T2-weighted MRI. For all in vitro experiments, the experimental parameters were FOV = 12 × 12 mm, matrix = 96 × 96, and slice thickness = 0.8 mm. Inductively coupled plasma atomic emission spectroscopy (ICP-AES) (Agilent 5100) was employed to analyze the element concentrations in the Lipo-NaYF4:Nd/NaDyF4 NCs.
Conclusions
In summary, Lipo-NaYF4:Nd/NaDyF4 NCs have been developed as imaging contrast agents for multimodal self-confirmed NIR-II fluorescence and MR imaging of cancer cells in vitro and tumors in vivo. Owing to the partial elimination of surface defects of the NaYF4:Nd NCs coated with the NaDyF4 layer, the NIR-II fluorescence intensities of Nd3+ ions in core–shell NCs at 1058 nm and 1332 nm are 3.46- and 1.75-fold higher than that of the bare NaYF4:Nd3+ NCs, respectively. The formed multifunctional Lipo-NaYF4:Nd/NaDyF4 NCs are water-dispersible and stable, and exhibit a low cytotoxicity at concentrations up to 400 μg mL−1. Additionally, a high r2 relaxivity of 44.0 mM−1 s−1 was calculated. More importantly, the Lipo-NaYF4:Nd/NaDyF4 NCs provide a new strategy as imaging contrast agents for simultaneously enhanced NIR-II fluorescence and T2-weighted MR imaging, which can provide more comprehensive imaging information to improve diagnostic accuracy of tumors and make them more competitive in next generation bioimaging fields.
Author contributions
Both Junwei Zhao and Xin Wang contributed to analysis of the data and writing the manuscript. Huishan Hu carried out the synthesis of materials and the characterization experiments of the as-synthesized samples. Wenquan Liu contributed to analysis of the data. Xin Wang contributed to the conception and design of the experiment. All authors reviewed the manuscript.
Conflicts of interest
There are no conflicts to declare.
Acknowledgements
The work was supported financially by the National Natural Science Foundation of China (No. 11774384, 11174324 and 11404122), the Youth Innovation Promotion Association of Chinese Academy of Sciences (No. 2011235), the Opening Foundation of Henan Key Laboratory of Special Protective Materials (Grant No. SZKFJJ201903) and the First-Class Discipline Cultivation Project of Henan University (2020YLZDYJ10).
Notes and references
- P. Mi, D. Kokuryo, H. Cabral, H. Wu, Y. Terada, T. Saga, I. Aoki, N. Nishiyama and K. Kataoka, Nat. Nanotechnol., 2016, 11, 724–730 CrossRef CAS PubMed.
- M. A. Sowers, J. R. McCombs, Y. Wang, J. T. Paletta, S. W. Morton, E. C. Dreaden, M. D. Boska, M. F. Ottaviani, P. T. Hammond, A. Rajca and J. A. Johnson, Nat. Commun., 2014, 5, 5460 CrossRef PubMed.
- G. Hong, Y. Zou, A. L. Antaris, S. Diao, D. Wu, K. Cheng, X. Zhang, C. Chen, B. Liu, Y. He, J. Z. Wu, J. Yuan, B. Zhang, Z. Tao, C. Fukunaga and H. Dai, Nat. Commun., 2014, 5, 4206 CrossRef CAS PubMed.
- A.-C. F. Jean-Luc Bridot, S. Laurent, C. Rivière, C. Billotey, B. Hiba, M. Janier, V. Josserand, J.-L. Coll, L. Vander Elst, R. Muller, S. Roux, P. Perriat and O. Tillement, J. Am. Chem. Soc., 2007, 129, 5076–5084 CrossRef PubMed.
- G. Song, X. Zheng, Y. Wang, X. Xia, S. Chu and J. Rao, ACS Nano, 2019, 13, 7750–7758 CrossRef CAS PubMed.
- T. E. Yankeelov, R. G. Abramson and C. C. Quarles, Nat. Rev. Clin. Oncol., 2014, 11, 670–680 CrossRef PubMed.
- L. Wu, A. Mendoza-Garcia, Q. Li and S. Sun, Chem. Rev., 2016, 116, 10473–10512 CrossRef CAS.
- J. E. Lemaster, F. Chen, T. Kim, A. Hariri and J. V. Jokerst, ACS Appl. Nano Mater., 2018, 1, 1321–1331 CrossRef CAS.
- L. Jing, K. Ding, S. V. Kershaw, I. M. Kempson, A. L. Rogach and M. Gao, Adv. Mater., 2014, 26, 6367–6386 CrossRef CAS PubMed.
- S. Yan, X. Zeng, Y. Tang, B. F. Liu, Y. Wang and X. Liu, Adv. Mater., 2019, 31, 1905825 CrossRef CAS PubMed.
- X. Wu, Q. Zhang, X. Wang, H. Yang and Y. Zhu, Eur. J. Inorg. Chem., 2011, 2011, 2158–2163 CrossRef.
- J. Zhao, H. Yang, J. Li, Y. Wang and X. Wang, Sci. Rep., 2017, 7, 18014 CrossRef PubMed.
- J. Zhao, X. Li, X. Wang and X. Wang, Nanoscale Res. Lett., 2019, 14, 200 CrossRef PubMed.
- J. Zhao, Z. Zhang and X. Wang, J. Alloys Compd., 2020, 820, 153142 CrossRef CAS.
- X. Wang, H. Hu, H. Zhang, C. Li, B. An and J. Dai, Nano Res., 2018, 11, 1069–1081 CrossRef CAS.
- X. Wang, Q. Zhang, J. Zhao and J. Dai, J. Mater. Chem. B, 2013, 1, 4637–4643 RSC.
- Q. Chen, X. Wang, F. Chen, Q. Zhang, B. Dong, H. Yang, G. Liu and Y. Zhu, J. Mater. Chem., 2011, 21, 7661–7667 RSC.
- G. Hong, A. L. Antaris and H. Dai, Nat. Biomed. Eng., 2017, 1, 0010 CrossRef CAS.
- Y. Fan and F. Zhang, Adv. Opt. Mater., 2019, 7, 1801417 CrossRef.
- Y. Zhong, Z. Ma, F. Wang, X. Wang, Y. Yang, Y. Liu, X. Zhao, J. Li, H. Du, M. Zhang, Q. Cui, S. Zhu, Q. Sun, H. Wan, Y. Tian, Q. Liu, W. Wang, K. C. Garcia and H. Dai, Nat. Biotechnol., 2019, 37, 1322–1331 CrossRef CAS PubMed.
- H. Li, X. Wang, X. Li, S. Zeng and G. Chen, Chem. Mater., 2020, 32, 3365–3375 CrossRef CAS.
- Y. Li, G. Bai, S. Zeng and J. Hao, ACS Appl. Mater. Interfaces, 2019, 11, 4737–4744 CrossRef CAS PubMed.
- A. Benayas, F. Ren, E. Carrasco, V. Marzal Beneyto, B. del Rosal, B. Gonfa, A. Juarranz, F. Sanz-Rodriguez, D. Jaque, J. Garcc-a-Soic, D. Ma and F. Vetrone, Adv. Funct. Mater., 2015, 25, 6650–6659 CrossRef CAS.
- H. Pan, P. Zhang, D. Gao, Y. Zhang, P. Li, L. Liu, C. Wang, H. Wang, Y. Ma and L. Cai, ACS Nano, 2014, 8, 5468–5477 CrossRef CAS PubMed.
- G. Hong, J. T. Robinson, Y. Zhang, S. Diao, A. L. Antaris, Q. Wang and H. Dai, Angew. Chem., Int. Ed., 2012, 51, 9818–9821 CrossRef CAS PubMed.
- Y. Zhang, G. Hong, Y. Zhang, G. Chen, F. Li, H. Dai and Q. Wang, ACS Nano, 2012, 6, 3695–3702 CrossRef CAS PubMed.
- R. Hu, W. C. Law, G. Lin, L. Ye, J. Liu, J. Liu, J. L. Reynolds and K. T. Yong, Theranostics, 2012, 2, 723–733 CrossRef CAS PubMed.
- G. Hong, S. Diao, J. Chang, A. L. Antaris, C. Chen, B. Zhang, S. Zhao, D. N. Atochin, P. L. Huang, K. I. Andreasson, C. J. Kuo and H. Dai, Nat. Photonics, 2014, 8, 723–730 CrossRef CAS PubMed.
- S. Diao, G. Hong, A. L. Antaris, J. L. Blackburn, K. Cheng, Z. Cheng and H. Dai, Nano Res., 2015, 8, 3027–3034 CrossRef CAS.
- G. Hong, S. Diao, A. L. Antaris and H. Dai, Chem. Rev., 2015, 115, 10816–10906 CrossRef CAS PubMed.
- A. L. Antaris, H. Chen, K. Cheng, Y. Sun, G. Hong, C. Qu, S. Diao, Z. Deng, X. Hu, B. Zhang, X. Zhang, O. K. Yaghi, Z. R. Alamparambil, X. Hong, Z. Cheng and H. Dai, Nat. Mater., 2016, 15, 235–242 CrossRef CAS PubMed.
- B. Li, L. Lu, M. Zhao, Z. Lei and F. Zhang, Angew. Chem., Int. Ed., 2018, 57, 7483–7487 CrossRef CAS PubMed.
- U. Rocha, K. U. Kumar, C. Jacinto, I. Villa, F. Sanz-Rodrc-guez, M.-a. del Carmen Iglesias de la Cruz, A. Juarranz, E. Carrasco, F. C. J. M. van Veggel, E. Bovero, J. G.-a. Soic and D. Jaque, Small, 2014, 10, 1141–1154 CrossRef CAS PubMed.
- B. del Rosal, A. Perez, M. g. Misiak, A. Bednarkiewicz, A. Vanetsev, Y. Orlovskii, D. Jovanovic, M. Dramicanin, U. Rocha, K. Kumar, C. Jacinto, E. Navarro, E. Martc-n Rodrc-guez, M. Pedroni, A. Speghini, G. Hirata, I. Martin and D. Jaque, J. Appl. Phys., 2015, 118, 143104 CrossRef.
- G. Chen, T. Y. Ohulchanskyy, S. Liu, W.-C. Law, F. Wu, M. T. Swihart, H. C gren and P. N. Prasad, ACS Nano, 2012, 6, 2969–2977 CrossRef CAS PubMed.
- A. Bednarkiewicz, D. Wawrzynczyk, M. Nyk and W. Strek, Opt. Mater., 2011, 33, 1481–1486 CrossRef CAS.
- D. H. Ortgies, M. Tan, E. C. Ximendes, B. del Rosal, J. Hu, L. Xu, X. Wang, E. MartC-n Rodrc-guez, C. Jacinto, N. Fernandez, G. Chen and D. Jaque, ACS Nano, 2018, 12, 4362–4368 CrossRef CAS PubMed.
- R. W. Mengyao Zhao, B. Li, Y. Fan, Y. Wu, X. Zhu and F. Zhang, Angew. Chem., Int. Ed., 2018, 58, 2050–2054 CrossRef PubMed.
- F. Ren, L. Ding, H. Liu, Q. Huang, H. Zhang, L. Zhang, J. Zeng, Q. Sun, Z. Li and M. Gao, Biomaterials, 2018, 354, 30–43 CrossRef PubMed.
- I. Villa, A. Vedda, I. X. Cantarelli, M. Pedroni, F. Piccinelli, M. Bettinelli, A. Speghini, M. Quintanilla, F. Vetrone, U. Rocha, C. Jacinto, E. Carrasco, F. S. Rodrc-guez, C. n. Juarranz, B. del Rosal, D. H. Ortgies, P. H. Gonzalez, J. G.-a. Soic and D. J. Garcc-a, Nano Res., 2015, 8, 649–665 CrossRef CAS.
- X.-F. Yu, L.-D. Chen, M. Li, M.-Y. Xie, L. Zhou, Y. Li and Q.-Q. Wang, Adv. Mater., 2008, 20, 4118–4123 CrossRef CAS.
- Y. Chen, H. Chen, S. Zhang, F. Chen, S. Sun, Q. He, M. Ma, X. Wang, H. Wu, L. Zhang, L. Zhang and J. Shi, Biomaterials, 2012, 33, 2388–2398 CrossRef CAS PubMed.
- H. Yang, Y. Zhuang, H. Hu, X. Du, C. Zhang, X. Shi, H. Wu and S. Yang, Adv. Funct. Mater., 2010, 20, 1733–1741 CrossRef CAS.
- L. Cheng, K. Yang, Y. Li, J. Chen, C. Wang, M. Shao, S.-T. Lee and Z. Liu, Angew. Chem., Int. Ed., 2011, 50, 7385–7390 CrossRef CAS.
- H. Yang, C. Zhang, X. Shi, H. Hu, X. Du, Y. Fang, Y. Ma, H. Wu and S. Yang, Biomaterials, 2010, 31, 3667–3673 CrossRef CAS PubMed.
- Y. Luo, S. Du, W. Zhang, Z. Liao, F. Zuo and S. Yang, RSC Adv., 2017, 7, 37929–37937 RSC.
- J. Zhou, Z. Lu, G. Shan, S. Wang and Y. Liao, Biomaterials, 2014, 35, 368–377 CrossRef CAS PubMed.
- W. Yuan, D. Yang, Q. Su, X. Zhu, T. Cao, Y. Sun, Y. Dai, W. Feng and F. Li, Adv. Funct. Mater., 2016, 26, 8631–8642 CrossRef CAS.
- S. Viswanathan, Z. Kovacs, K. N. Green, S. J. Ratnakar and A. D. Sherry, Chem. Rev., 2010, 110, 2960–3018 CrossRef CAS PubMed.
- J. Zhou, Q. Liu, W. Feng, Y. Sun and F. Li, Chem. Rev., 2015, 115, 395–465 CrossRef CAS PubMed.
- W. Fan, W. Bu and J. Shi, Adv. Mater., 2016, 28, 3987–4011 CrossRef CAS PubMed.
- J. Jin, Z. Xu, Y. Zhang, Y.-J. Gu, M. H.-W. Lam and W.-T. Wong, Adv. Healthcare Mater., 2013, 2, 1501–1512 CrossRef CAS PubMed.
- G. Chen, I. Roy, C. Yang and P. N. Prasad, Chem. Rev., 2016, 116, 2826–2885 CrossRef CAS PubMed.
- J. Nam, N. Won, J. Bang, H. Jin, J. Park, S. Jung, S. Jung, Y. Park and S. Kim, Adv. Drug Delivery Rev., 2013, 65, 622–648 CrossRef CAS PubMed.
- S. Shanmugam, R. Baskaran, P. Balakrishnan, P. Thapa, C. S. Yong and B. K. Yoo, Eur. J. Pharm. Biopharm., 2011, 79, 250–257 CrossRef CAS PubMed.
- D. Liu, X. Xu, Y. Du, X. Qin, Y. Zhang, C. Ma, S. Wen, W. Ren, E. M. Goldys, J. A. Piper, S. Dou, X. Liu and D. Jin, Nat. Commun., 2016, 7, 10254 CrossRef CAS PubMed.
- B. Xu, X. Zhang, W. Huang, Y. Yang, Y. Ma, Z. Gu, T. Zhai and Y. Zhao, J. Mater. Chem. B, 2016, 4, 2776–2784 RSC.
- L. J. Tian, Y. J. Sun, Y. Yu, X. G. Kong and H. Zhang, Chem. Phys. Lett., 2008, 452, 188–192 CrossRef CAS.
- Y. Sun, Y. Chen, L. Tian, Y. Yu, X. Kong, J. Zhao and H. Zhang, Nanotechnology, 2007, 18, 275609 CrossRef.
- Y. Wang, L. Tu, J. Zhao, Y. Sun, X. Kong and H. Zhang, J. Mater. Chem. C, 2009, 113, 7164–7169 CAS.
- S. Carron, Q. Y. Li, L. Vander Elst, R. N. Muller, T. N. Parac-Vogt and J. A. Capobianco, Dalton Trans., 2015, 44, 11331–11339 RSC.
- G. K. Das, N. J. J. Johnson, J. Cramen, B. Blasiak, P. Latta, B. Tomanek and F. C. J. M. van Veggel, J. Phys. Chem. Lett., 2012, 3, 524–529 CrossRef CAS.
- S. Josef, P. Katerina, H. Marketa, J. Ivan, M. Massimiliano, M. David, K. Lucia, S. Jan, P. Helena and G. Eva, Int. J. Nanomed., 2014, 9, 5355–5372 Search PubMed.
- E. Poselt, H. Kloust, U. Tromsdorf, M. Janschel, C. Hahn, C. Masslo and H. Weller, ACS Nano, 2012, 6, 1619–1624 CrossRef CAS PubMed.
- Q. Zhang, X. Wang and Y. Zhu, J. Mater. Chem., 2011, 21, 12132 RSC.
- L. L. Li, R. Zhang, L. Yin, K. Zheng, W. Qin, P. R. Selvin and Y. Lu, Angew. Chem., Int. Ed., 2012, 51, 6121–6125 CrossRef CAS PubMed.
- C. Yao, P. Wang, L. Zhou, R. Wang, X. Li, D. Zhao and F. Zhang, Anal. Chem., 2014, 86, 9749–9757 CrossRef CAS.
Footnotes |
† Electronic supplementary information (ESI) available. See DOI: 10.1039/d0na00846j |
‡ These authors contributed equally. |
|
This journal is © The Royal Society of Chemistry 2021 |