DOI:
10.1039/D1MA00539A
(Review Article)
Mater. Adv., 2021,
2, 6942-6983
Crystal facet and surface defect engineered low dimensional CeO2 (0D, 1D, 2D) based photocatalytic materials towards energy generation and pollution abatement
Received
21st June 2021
, Accepted 5th September 2021
First published on 13th September 2021
Abstract
Surface defect engineering and crystal facet engineering are two important strategies which effectively enhance the efficiency of a photocatalyst by modulating its physiochemical properties. To date, surface defect and morphology dependent photoactivity has been explored by using different nanostructured photocatalysts. Among them CeO2 based materials have recently received foremost interest, due to their easily tunable surface structure and low formation energy for surface defects. Therefore, in this review we have comprehensively summarized the recent achievements and progress in surface defect enriched low dimensional nanostructured CeO2 and CeO2 based heterostructure photocatalysts towards clean fuel generation reactions such as water splitting, CO2 reduction, N2 fixation and organic/inorganic environmental pollutant removal applications. The present review highlights the design of CeO2 with particular crystal facets and morphology and the positive impact of the crystal facets on the construction of different CeO2 based heterostructure materials (0D–2D, 0D–1D, 1D–2D, 2D–2D, 0D–3D, etc.) with other low dimensional photocatalysts. Next, the surface defects, crystal facets and heterojunctional interfaces responsible for improved photoactivity are briefly discussed with proper experimental evidence and the mechanism along with the theoretical concept is explained. Further, this review sheds light on the current challenges and future perspectives in this research area.
| Dr Sabiha Sultana received her bachelor's degree (1st Rank) from Utkal University, Bhubaneswar, in the year 2012 and MSc in Chemistry from Ravenshaw University, Cuttack, in the year 2014. Then, she joined as a PhD student under the supervision of Prof. Kulamani Parida at the Centre for Nano Science and Nano Technology, S‘O’A Deemed to be University, and recently completed her PhD degree. Her research area focuses on the development of nanostructured materials such as metal oxides, metal sulphides, and phosphides, and their application towards water splitting, N2 reduction, CO2 reduction, and pollution abatement. She has published 14 research articles in various international journals. |
| Dr Sriram Mansingh received his BSc (2nd Rank), MSc and MPhil (1st Rank) from Utkal University, India. Then, he completed his PhD degree at the Siksha ‘O’ Anusandhan (Deemed to be University) Bhubaneswar, India, under the guidance of Prof. Kulamani Parida. His current research mainly focuses on the development of nanostructured materials such as metal oxides, metal sulphides, and phosphides, and their application towards water splitting, N2 fixation and organic/inorganic pollutant detoxification. He has published 24 research articles in various international journals and one patent. |
| Prof. Kulamani Parida is a globally distinguished scientist in the area of materials science, catalysis and nanotechnology. He superannuated as a Chief Scientist and a Professor of Academy of Scientific and Innovative Research (AcSIR) from CSIR-IMMT, Bhubaneswar, in 2014. His adoration for Chemistry and passion towards research couldn't let Prof. Parida sit idle after retirement. With the same devotion and dedication, Prof. Parida (Director) started the ‘Centre for Nano Science and Nano Technology’ a research unit of Siksha ‘O’ Anusandhan (deemed to be university), Bhubaneswar. His research interests include the design and development of advanced materials encompassing a wide variety such as metal oxides/phosphates/sulfates, cationic and anionic clays, perovskites, MOFs, carbon based materials including graphene, g-C3N4, and naturally occurring materials such as manganese nodules, manganese nodule leached residues, and manganese oxides of natural origin for fine chemical, energy and environmental applications. He has published more than 500 research articles and 10 review articles in renowned international journals. He has 18 524 citations to his credit with an h-index of 72 and an i10-index of 326. He has 27 national and international patents to his credit and is the author of four book chapters. For more details: https://kmparidaimmt.weebly.com/. |
1. Introduction
Energy shortage and environmental pollution are pressing concerns that adversely impact the sustainable development of our society. Hence, the development of green, sustainable and energy-efficient methods for energy production and pollutant detoxification is of utmost concern. With regard to this, semiconductor photocatalysis has gained enormous popularity as it can convert solar energy into some useful energy feedstocks and also act as a boon for remediation of environmental pollutants.1–8 However, a major difficulty faced in this area is the low efficiency of the photocatalyst. The efficacy of a photocatalytic system generally depends upon three mechanistic features such as appropriate excitation, bulk diffusion and surface charge transfer.9–12 In order to achieve the higher efficiency of a photocatalyst, all these three properties have to be controlled in a single platform. With the development of modern technology and advanced characterization techniques, the terminologies surface defect engineering and crystal facet engineering have come to light very recently.13–15 It is found that either of these two approaches is a game changing entity for enhancing the reactivity and selectivity of a photocatalyst by modulating its physical and chemical properties.
Crystal facet engineering can efficiently modify the kinetic parameters responsible for the improved charge pair transfer and separation parameter and reaction rates of a photocatalytic reaction. In brief, morphologies with different exposed facets have different atomic rearrangement and co-ordination, and hence different morphologies exhibit different surface electronic structures (band gap, band edge potential, etc.), electrical conductivity, surface built-in electric field, reaction centers and reactant adsorption sites.16–19 By simply changing an exposed plane to another one, there is a drastic change in the photoactivity and selectivity of a photocatalyst for a photocatalytic reaction15, so there is an increased interest in morphology tuning and crystal facet engineering. While considering a multicomponent system, crystal facet engineering in nanostructured hybrids is quite complicated, as a heterostructural interface is evolved between the individual components. Those hybrids possess individual components with their own crystal planes of different orientation as well as a distinct interface with different band energy alignment. In most of the cases, proper band alignment at the interface between two individual components is found to be very crucial for interfacial charge transfer and separation. Hence the rational design of photocatalytic systems with active surface planes and suitable interfaces is very challenging.20–26 The Arienzo group prepared TiO2 with (001) and (101) exposed facets by using a morphology directing agent. Both theoretically and experimentally, they proved that the 001 surface is more active for photocatalytic oxidation reaction while the exposed 101 surface is a reductive surface, as it didn’t directly assist the oxidative process.22 In another example, Bomio et al. prepared a PbMoO4 photocatalyst with predominant 111, 100, 011 and 110 facets by using a template directing agent. They found that the 001 surface exhibited the lowest activity for RhB photodegradation as the surface acts as a recombination centre of photogenerated electrons and holes.23 Further, Zhu et al. prepared 010 and 011 facet exposed BiVO4. They found that the morphology and the distinct facets play a vital role in the anisotropic charge distribution at the photocatalyst surface. For the 011 facet the surface photovoltage signal was 70 times higher than that on the 010 facet; hence the former could be a better photocatalyst.24 Further, Naresh et al. explained the interaction of two exposed facets of two individual components in a heterostructure. They reported that 110 exposed Cu2O (rhombic dodecahedra) was a more active photocatalyst towards MO degradation than 111 exposed Cu2O (octahedral), while cubic 100 exposed facet Cu2O is photocatalytically inactive. But when these are made into heterostructures with 200 exposed ZnS, Cu2O(111)/ZnS(200) performed well in comparison to Cu2O(110)/ZnS(200). The reason was attributed to the unfavourable band alignment within Cu2O(110) and ZnS(200), showing the lowest charge separation efficiency.25 Hence photocatalytic activity is also strongly related to the exposed facet as well as their interfacial interaction.
Surface defects are another interesting feature which has a positive role in enhancing the photoactivity of a photocatalyst; hence defect engineering is catching more scientific significance for modifying the properties of a photocatalyst. In general, defects are created in a slightly reductive environment where the periodic arrangement is broken with little lattice distortion, and this can change intrinsic semiconductor properties such as orbital hybridization, conductivity, atom coordination number, and light absorption ability and can also change the electron density around the Fermi level.27–30 Basically there are two types of defects, i.e. bulk defects and surface defects, and among these two, surface defects enhance the charge separation efficiency of a photocatalyst while bulk defects act as a recombination center for photogenerated charge carriers.31,32 The surface defects are again classified into many divisions on the basis of dimension such as point defects, line defects, planar defects and volume defects. Surface anion vacancies (oxygen, sulphur and nitrogen) are regarded as the most explored type of vacancy which are employed in photocatalysis to modify the activity of the photocatalyst by enhancing its light absorption ability and charge transfer and separation efficiency and drive the surface reactions.33,34 Moreover, in a multicomponent nanohybrid, additional defects, i.e. interfacial defects, are found which enhance the photoactivity by accelerating the interfacial charge pair separation.35 However the controlled design of desirable vacancies in single and multicomponent photocatalysts without complete lattice distortion is very demanding. Hence, many studies have been concentrated on the design of a photocatalytic system with the combination of both surface defects and exposed crystal facets. For example, Li et al. prepared an oxygen vacancy enriched BiOBr(001) nanosheet and tested its effectivity towards the photocatalytic nitrogen reduction reaction. They found that the oxygen vacancy centres successfully activated the adsorbed nitrogen on BiOBr by π-back donation and significantly promoted the interfacial electron transfer from BiOBr to the activated nitrogen.36 In another report, Zhang et al. designed a defect enriched WO3 nanosheet and found that the as-prepared photocatalyst efficiently coupled sunlight into aerobic coupling of amines to the corresponding imines with excellent stability and enhanced the kinetic rate 6 times compared with defect deficient WO3. They deduced that defect engineering can serve as a versatile approach to refine the photocatalyst.37 Apart from the surface defects, interfacial defects in a heterojunction photocatalyst also play a vital role in photocatalysis. Zhang et al. successfully prepared a heterostructure between MoS2 QDs and ZnIn2S4 in a 2-D architecture with interfacial S-vacancies. The vacancies acted as electron traps and avoided the vertical transmission of electrons.38 Hence the heterostructure exhibited a high photocatalytic hydrogen evolution rate, which was higher than that of neat systems without vacancies.
Rare earth cerium oxide (CeO2) is considered as one of the most attractive metal oxides which can easily be tuned into different shapes with desired exposed facets. Further, another feature, i.e. surface defects, can easily be formed with very slight energy expense at the CeO2 surface. Due to these special features, it is often used as a catalyst in various catalytic applications such as solid oxide fuel cells (SOFCs), ultraviolet adsorbents, glass polishing materials, water gas shift reaction, PROX (CO oxidation), oxygen sensors, selective organic transformation, photocatalytic water oxidation and pollutant degradation.27,39–43 In addition to this, CeO2 has other special features such as cost-effectiveness, high stability, low toxicity and specific physical and chemical characteristics, such as high oxygen storage capacity (OSC), oxygen ion conductivity and fast shuttling of oxidation state (Ce3+ → Ce4+), which makes it an ideal candidate for photocatalysis.44–59 In brief, the rare earth CeO2 has a crystal structure of triclinic, tetragonal or cubic phase. However, mostly CeO2 crystallizes in the cubic fluorite structure with a space group of Fm
m (a = 0.541134 nm) over the temperature range extending from room temperature to its melting point.48 The fluorite structure consists of a face centred cubic unit cell of Ce4+ while eight oxygen anions occupy the tetrahedral interstitial sites. As a result, each Ce4+ is octahedrally coordinated by eight oxygen anions while each oxygen ion is tetrahedrally coordinated by four Ce4+ cations forming a CeO8 cluster as the basic unit. In cubic CeO2, mainly three exposed planes (111), (110) and (100) are observed, but due to thermodynamic restrictions, CeO2 predominantly exposes the (111) plane, as it is very stable and requires low surface energy. The (111) surface plane is terminated by three-fold co-ordinated oxygen atoms, while the polar (100) surface is terminated by two-fold co-ordinated oxygen atoms. The (110) surface is terminated by a CeO2 plane with three-fold co-ordinated oxygen and six-fold co-ordinated cerium atoms.29
Surface defects especially oxygen vacancies are termed as the most important feature of CeO2, which regulates all its properties. In a slightly reductive environment or at a low partial pressure of oxygen, surface defects (oxygen vacancies, interstitial metal defects) are generated on CeO2, as they require low formation energy. In brief, surface defects in CeO2 are generally created when the on plane oxygen is released from the lattice yielding a CeO7 type cluster with the concomitant oxygen vacancy formed in the crystal lattice. Further, these vacancies are accompanied by localization of the electrons left behind in the Ce 4f state leading to the formation of two Ce3+ ions. Hence, altogether oxygen vacancies and Ce3+ are termed as surface defects on CeO2 as shown in Fig. 1(a).50,51 The reaction of defect formation is as follows,51 where O×0 is the oxide ion in the lattice,
is the charged oxygen vacancy and e′ represents the electrons in the conduction band:
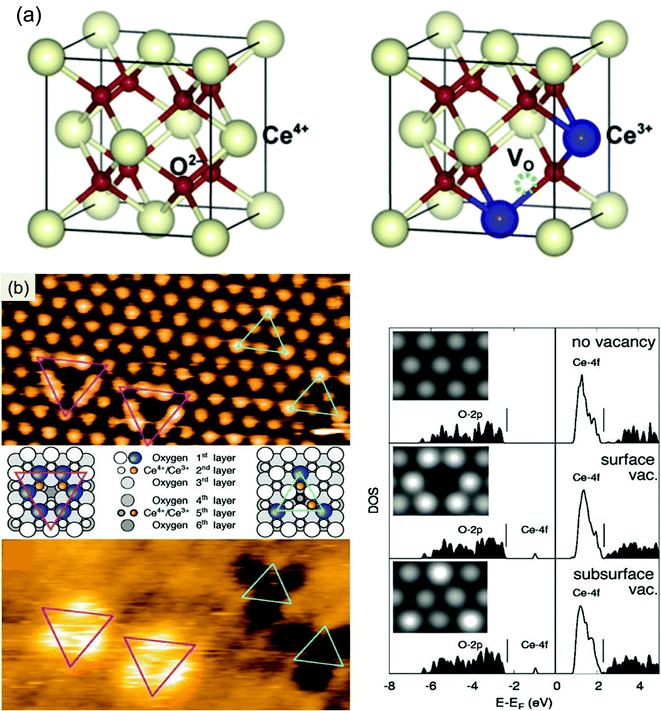 |
| Fig. 1 (a) Crystal structure of CeO2 with the formed oxygen vacancy. Reprinted from ref. 50 with permission from Elsevier. (b) Filled-state and empty-state STM images of single and subsurface vacancies and related structural models and calculated density of states (DOS) and simulated filled-state STM images. Reproduced from ref. 57 with permission from Science. | |
In addition to the doubly charged oxygen vacancy, singly charged and neutral vacancies are also present in the defective CeO2 lattice.54 Huang et al. reported a neutral oxygen vacancy
centre in CeO2 with very low formation energy in an oxygen-deficient environment. It acts as a deep donor with negative U (repulsive potential) behaviour leading to excellent photoactivity. In addition to this, easy shuttling of Ce3+↔4+ helps to generate an anion Frankel defect which enhances oxygen storage as well as release capability.52 Further, to know the structure and geometry of oxygen vacancies, Esch et al. thoroughly studied the defect formation and nature of defects from STM images and DFT calculations. They found that two types of oxygen vacancies are predominantly formed: the first type is the simple one oxygen vacancy which appears as a depression surrounded by three lobes, shown by the magenta triangle in Fig. 1(b), and the second type, which is called the subsurface vacancy, appears as triple protrusions centred on third layer oxygen sites (shown by the cyan triangle in Fig. 1(b)). The delocalization of the electron left behind determines which type of vacancy can be formed on CeO2. Hence, a permutation and combination of single and subsurface oxygen vacancies creates different dimeric and trimeric vacancy clusters with different orientation, which causes a major structural rearrangement in the crystal lattice of CeO2.57
Next is the optical property of CeO2. Theoretically the optical band gap of CeO2 is 6–8 eV because of the transition between O 2p and Ce 5d states.49 And, the experimental result reveals a bandgap of around 3 eV due to the charge transfer spectra of Ce(IV)–O(II), while the band gap may vary from 3.2 to 2.8–2.7 eV. The drastic change in the band gap and in the colour from yellow to blue-black arises only due to the presence of surface defects in CeO2.53 The change is due to the oxygen vacancy, which creates a Ce3+ state; thus the energy of the 4f orbitals shifts towards lower potential and hence shortens the bandgap. The density of state (DOS) of Ce 4f for Ce(III) is larger than that of Ce(IV) in the positive energy range, which indicates that Ce(III) can be more easily excited than Ce(IV) under light irradiation, which suggests that the oxygen vacancy enhances visible light absorption.55 In addition to the optical property, the oxygen vacancy of CeO2 also has a profound effect on the electrical as well as magnetic character. With the increase in oxygen vacancy concentration, the movement of the oxygen atom becomes easier in the crystal, as the vacancy easily migrates throughout the lattice via a vacancy hopping mechanism at an elevated temperature, and hence the crystal has a high electrical conductivity.56–58 This increased diffusion rate in the lattice is a probable cause of the increase in catalytic activity.58 Chen et al. reported that the intrinsic surface defects also have a direct relationship with the magnetic behaviour of CeO2. They found that an increase in the local oxygen vacancies and Ce3+ concentration directly increases the magnetism of CeO2 nanoparticles. However, after a certain concentration of Ce3+ the magnetic properties gradually decrease and vanish completely. The reduction of magnetic moments may be due to ion pair formation by oxygen vacancies which favours antiferromagnetism. Further, from the X-ray magnetic circular dichroism measurement and theoretical analysis, they found that the electrons in Ce bear magnetic moments and show magnetism via bound magnetic polarons which are constituted by oxygen vacancies and an oxygen mediated super-exchange framework (Ce3+–O–Ce3+).5 Therefore it is confirmed that oxygen defects and shuttling of oxidation states in CeO2 lead to some peculiar changes in optoelectronic, electrical as well as magnetic properties.
Another interesting feature of ceria is its morphology variation; ceria can be easily tailored to different morphologies such as nanorods, nanotubes, nanocubes, nanopolyhedra, and nanosheets with exposed active facets. Moreover, particle size and morphology have a prominent effect on the intrinsic surface defect formation in CeO2. From the literature survey, it was found that the smaller the particle size is, the higher the surface to volume ratio will be, and hence the more defects there will be. Additionally, designing a particular shape or exposure of a specific plane in CeO2 decides the formation and concentration of defects. Based on the DFT calculation, the (100) plane exhibits the lowest energy for vacancy formation, while the (111) crystal plane requires more energy. So based on this, nanostructures of (100) and (110) exposed facets contain more surface defects than (111) oriented surface structures.60–64 Wu's group evaluated the surface defects and and their relationship with the photoactivity of different exposed planes of three types of CeO2 nanostructures such as nanorods, nanocubes and nano-octahedra. Via UV-Raman spectroscopy and O2 adsorption analysis, they found that both oxidized and reduced CeO2 of nanorod morphology ((110) + (100)) has the most intrinsic defects followed by nanocubes (100) and nano-octahedra (111). Furthermore, the team observed that some defects are of one electron containing singly charged species while some are two electrons containing doubly charged.63 Recently by combining experimental and theoretical results, Oliveira and his group concluded that CeO2 consisting of the (111) exposed plane contains only one vacancy, due to the presence of the [CeO7·V0×] cluster, while (100) and (110) crystal planes exhibit two vacancies, because of the presence of the [CeO6·2V0×] cluster. In the samples consisting of (100) and (110) facets with two vacancies, there is a resonance between the vacancies which stabilizes the interaction between electrons and holes. Moreover, the one vacancy containing facet shows higher stability for the formed holes only, which reduces the electrical resistance to some extent. Hence the morphology containing (100) and (110) planes shows higher photoactivity and lower electrical resistance than the (111) plane containing morphology.54 In most cases, surface defects, exposed planes and morphology of CeO2 are the key factors that decide the enhancement in catalytic as well as photocatalytic activity.62–64 However, the vacancies can easily be healed in an oxidative environment (O-rich) and sometimes act as hole trapping centers which retards photo-efficiency. Importantly, designing a specific facet exposed system is very challenging. So fabricating CeO2 based nanomaterials with controlled surface defects and a well compatible merged facet interface is getting more attention and interest.
Therefore more emphasis has been given to the construction of hybrid/heterostructure based photocatalytic systems for potential application in the photocatalysis field. So far many such CeO2 based photocatalytic systems have been made and from the recent studies it was found that the construction of heterostructure photocatalysts of different morphologies, with other visible light semiconductors, is a feasible and effective method to improve the photogenerated electron–hole separation which ultimately improves the photocatalytic efficiency under visible light. In this approach, a wide bandgap metal-oxide semiconductor, i.e. CeO2, is often combined with a narrow bandgap semiconductor such as other metal oxides, sulphides, phosphides, and selenides to form a heterostructure oriented system.45–48 Further, the in situ generation of surface defects helps to minimize the hole–electron recombination and provides a strong interfacial surface for reactant binding and the corresponding dissociation, resulting in improved photoefficiency. Regarding this concept, several articles corresponding to the CeO2 based photocatalytic systems have been published in the field of solar fuel generation and pollutant removal, which we have discussed in the application section.
Nanostructured CeO2 has witnessed numerous possible scientific advancements and has always been the centre of attraction in photocatalysis, as summarized in Fig. 2. Currently, extensive studies have been made towards developing efficient CeO2 based photocatalytic systems by adopting different advanced strategies such as surface interface tuning and defect engineering. Hence, there are several reviews on CeO2 covering areas such as its properties, synthesis, nanostructure and catalytic application.27,39,45–48 However, a systematic review on the current progress and growth trend of exposed facet and defect mediated CeO2 based materials is highly needed to update the scientific society with regard to photocatalysis. So in this review, we have specifically discussed the strategic updates and the latest progress in exposed facet, morphology dependent CeO2 with defect engineering and then CeO2 based heterostructured materials towards various photocatalytic applications. In brief, the review elaborates the basic concepts and the design strategy of lower dimension (2D, 0D, 1D, 3D) CeO2 with particular crystal facets and broadly summarizes the synthetic strategy involving CeO2 based materials with a distinct morphological interface such as 0D–2D, 0D–1D, 1D–2D, 2D–2D, 0D–3D, etc. Further, it also narrates the detailed defect chemistry of such heterointerface systems in different photocatalytic applications such as water splitting (H2 and O2 evolution), CO2 reduction, N2 reduction, and pollutant removal (dyes, phenolic derivatives, antibiotics and heavy metals) along with elucidation of morphology dependent activity correlations. At last, we throw some light on the challenges encountered and provide future direction for improvement of CeO2 based materials to achieve benchmark efficiency.
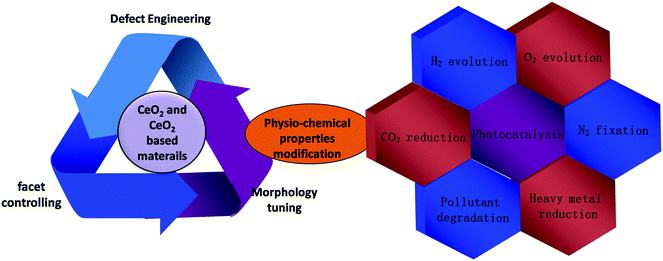 |
| Fig. 2 Complete schematic illustration of CeO2 based materials’ property tuning and applications. | |
2. Crystal facet engineering and defect designing
During the last two decades, several synthetic methods have been reported for the preparation of nanostructured CeO2. Generally the methods used for the synthesis of shape and size selective CeO2 with appropriate exposed active planes are precipitation, hydrothermal treatment, surfactant/capping agent-assisted solvothermal method, thermal decomposition, sol–gel combustion, template-directed synthesis, sonochemical method, microemulsion technique, electrochemical deposition and many more.65–91 More often two or more methods are combined to prepare facet orientated CeO2 as summarized in Fig. 3(a). Sun et al., Zhang et al. and Wang et al. have thoroughly explained the hidden mechanism and the science behind the adopted strategy toward designing different shape-selective nanostructured CeO2.39,45,46 Hence, in this section, we have briefly summarized some of the essential points for the synthesis of lower-dimensional facet oriented CeO2 and cited some latest examples of the corresponding synthesis.
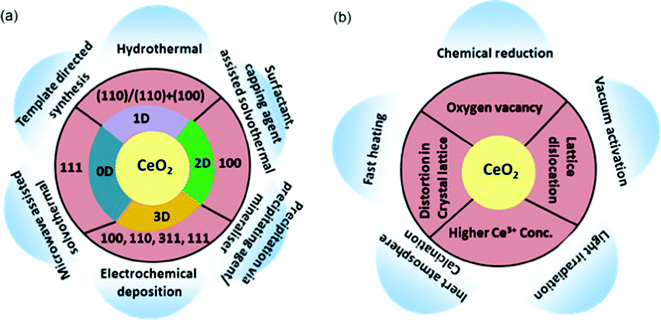 |
| Fig. 3 (a and b) Strategy for crystal facet designing and defect engineering. | |
The controlled nucleation and growth rate of CeO2 crystals determines the formation possibility of a particular facet, size and shape. Hence, appropriate additives such as precipitating agents/mineralisers [NaOH, urea, HMT, ammonia, etc.], template [soft, hard and self], surfactant/capping agent, organic ligands, polymers, etc., are often used to manage the exposed plane, shape and size of nanostructured CeO2. For example, the commonly used solutions to obtain facet oriented CeO2 are NaOH solution (cubes, rods, wire (110)), PO43− (octahedral, rod), and NH3·H2O (nanowires). In addition to these, the concentration of precipitants and the reaction temperature and time are also important for obtaining different exposed facets.65–96 Oliveria et al. prepared CeO2 of different morphology by simply varying the synthesis time via microwave-assisted hydrothermal synthesis. They found that when using cerium nitrate and NaOH, at first they obtained sheet like morphology, and after a certain time these sheets were elongated to form nanorods while after some time polyhedron type morphology was obtained followed by nanocubes.54 Further, it was reported that anions like Cl−, NO3−, SO42−, and PO43− mainly coming from the cerium precursor and the added precipitants have also influenced the morphology and the properties of CeO2. For example, Jiang et al. prepared CeO2 nanocubes and nanorods by using two different cerium precursors; for nanocubes, they used cerium chloride while for nanorods they used cerium nitrate. After the hydrothermal treatment at 110 °C for only 24 h, from both cerium precursors in NaOH solution, they got rod like morphology, while a further higher temperature treatment (180 °C) yielded nanocube type morphology with only the chloride containing precursor.15 In another report by Zhu et al., 110 exposed facet nanorods were prepared from cerium chloride and NaOH via hydrothermal treatment at 140 °C for 18 h, while 100 exposed nanocubes were prepared from the same concentration of NaOH with cerium nitrate precursor at 180 °C for 24 h.95 The controlled design of one kind of exposed facets requires more complicated reactions; hence in most of the cases nanostructured ceria is prepared by controlling the most active plane while other facets are also present but in a dormant form. Therefore a number of systematic studies on morphology oriented low dimensional nanostructured ceria with one (in some cases more than one) active facet have been carried out. From the conclusive research experiments it was found that by controlling the dimension of nanostructured CeO2 the desired active crystal facets can be controlled simultaneously. For example, by making 1-D nanorods or nanowires, 110 + 100 exposed facets are controlled wisely, while high surface to volume ratio 100 planes are controlled by making 2-D nanosheet and 3D-nanocube like structures.81,97,98 For example, Imagawa and Lin et al. prepared 100 exposed facet CeO2 nanoplates by using oleic acid and oleylamine, while Hao et al. prepared monodispersed 100 exposed ceria nanocubes by a simple hydrothermal method by using decanoic acid as an organic surfactant.99 In another report, a 200 exposed crystal facet CeO2 nanocube was prepared by Yang et al. using tert-butyl amine as the facet directing agent.100 Next, Liu et al. reported another CeO2 morphology, i.e. an octahedron with a predominant 111 exposed facet, via a hydrothermal method followed by high pressure.101 Hence, the synthesis of lower-dimensional CeO2 with controlled exposed facets requires conceptual designing and experimental evidence, which we have briefly discussed in the below segments.
In addition to crystal facet engineering, surface defect designing is another crucial strategy for remodifying the photocatalyst property. Doping is considered as an effective strategy to generate defects in CeO2; however, dopants form deep impurity levels within the forbidden band of semiconductors, where they act as recombination centres and impair photocatalytic activity.102 So in this review we have excluded the doping concepts and their impact on CeO2 based materials. Further, a number of synthetic methods such as chemical reduction, light irradiation, vacuum activation, inert atmosphere (N2, Ar, H2) calcination, etc. are often combined with the general nanostructured CeO2 preparation methods to achieve higher defect density at the surface as schematically presented in Fig. 3(b).28,103–106 Moreover, the different exposed facets exhibited different energy for vacancy formation. In general it is found that the 110 and 100 planes exhibited higher energy for surface vacancy formation while the 111 plane required a minimum energy. Among 110 and 100, 110 CeO2 has lower energy for vacancy formation than 100 CeO2. So via controlling the facets one can control the defects in CeO2. To further enhance the vacancy concentration, additional treatments as mentioned above are considered. For example Zhang et al. prepared oxygen vacancy enriched CeO2 nanorods by a one step hydrothermal method followed by in situ reduction treatment. Briefly, they used the cerium precursor and NaOH along with NaBH4 and treated the solution hydrothermally. NaBH4 here acts as a reducing agent and in situ generates oxygen vacancies during the growth of nanorods.103 And in some cases NaBH4 treatment was carried out after the complete preparation of CeO2 to increase the surface defect concentration.104 Next, Zhao et al. prepared defective CeO2 NRs by a simple hydrothermal method followed by calcination in different atmospheres (such as argon, a mixture of argon and hydrogen gas and air atmosphere) at 800 °C. Via systematic analysis through XPS, they found that in an Ar–H2 atmosphere maximum surface vacancies were produced, due to the reducing nature of hydrogen gas.69 Further, Aslam et al. synthesized nanosized CeO2 by using Triton X-100 as a surfactant via a solution combustion method followed by 500 °C calcination. They found that when the as-prepared CeO2 was additionally treated with direct sunlight, some CeO2−x was formed on the CeO2 surface. This defective CeO2 exhibited enhanced absorption in the visible region and its band gap reduced from 2.9 to 2.6 eV.105 Hezam et al. reported another Ov enriched CeO2via a sunlight assisted combustion method. They found that more defects are present in CeO2 prepared by the sunlight assisted combustion method compared to that by the conventional solution combustion method.106
3. Synthesis of nanostructured CeO2 and CeO2 based materials
3.1 Zero-dimensional CeO2
In recent years 0D quantum dots (QDs) have attracted great attention due to their size-derived properties which keep them one step ahead of their corresponding normal nanoparticles. The quantum confinement effect modulated extraordinary optoelectronic properties and prominent active sites make zero-dimensional materials promising candidates in a variety of applications including photocatalysis, electrocatalysis and biocatalysis.107 Different synthesis methods like non-hydrolytic sol–gel, hydrothermal, microwave-assisted solvothermal methods, etc.65–68 with suitable capping agents have been reported for the synthesis of CeO2 QDs. In this context, Xin et al. proposed a synthetic route to prepare monodisperse CeO2 QDs by heating a cerium precursor in triethylene glycol (TEG). In short, they obtained uniform monodisperse 5 nm QDs via a facile low cost, polyol condensation route which is based on the hydrolysis of the cerium alkoxide complex at an elevated temperature in the presence of an organic solvent (TEG) as shown in Fig. 4(a). The prepared CeO2 QDs show good redispersion ability and a high specific surface area.65 Further, Hassan and co-workers suggested two methodologies for the preparation of different size CeO2 QDs. In the typical hydrothermal process, they used a mixed solution of cerium precursor along with a particular molar concentration of bases (NaOH, NH4OH) and obtained 7 nm size QDs. While in the next strategy, they obtained 3 nm QDs via the non-hydrolytic sol–gel method by using the cerium precursor in polyamine and then adding the organic capping agent diphenyl ether to control the size of the nanoparticles.66 Moreover, in the above processes, more than one metal precursor was used in addition to the surfactant/capping agents which is quite essential for restricting the growth of QDs. In light of this, Lehne et al. used a single metal–organic precursor, i.e. a heteroleptic alkoxide complex of cerium, to obtain highly crystalline CeO2 QDs with a narrow size distribution via the microwave-assisted solvothermal synthesis in N-methyl pyrrolidine.67 Adding more to the text, for the biomedical application of quantum dots, their synthesis, storage and self-agglomeration were considered as serious parameters for their effective utilization. Hence incorporation of a suitable capping agent or organic moiety over the surface of QDs is an apparent solution. Arumugam's group synthesized 2.4 nm CeO2 QDs capped with the anionic group 2-amino-3-chloro-5-trifluoromethyl pyridine (ACTP). From XRD it was observed that capped as well as uncapped QDs exhibited a dominant (111) plane as shown in Fig. 4(b), and the QDs’ orientation was along the 110 direction. Further, they found another interesting fact, i.e. the synthesized QDs exhibited strong green emission at 295 nm (Fig. 4(c)), which confirms that the synthesized sample was in the quantum range with particle size ∼2.4 nm that is below its Bohr radius of 7–9 nm. Furthermore, it was found that due to the dielectric confinement effect the band gap energy values of QDs gradually decreased with the increasing amount of the capping agent, which is a good sign for photocatalysis.68
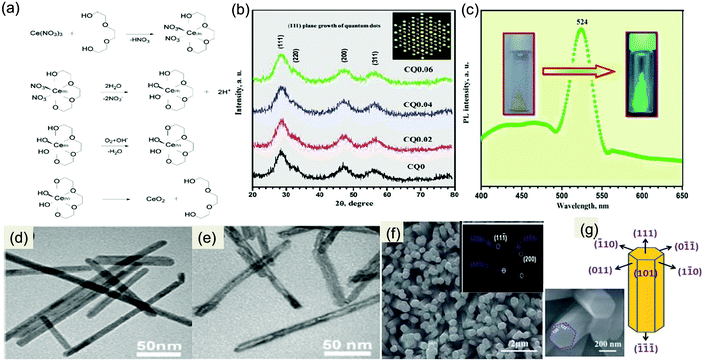 |
| Fig. 4 (a) Proposed mechanism for Ce chelated complex formation with the corresponding decomposition to quantum dots. Reprinted from ref. 65 with permission from Wiley. (b) and (c) XRD pattern of plane and ACTP capped CeO2 QDs; (inset) schematic (111) plane growth of QDs and PL spectrum of CeO2 QDs, exhibiting strong green emission at 524 nm. Reprinted with permission from ref. 68, copyright 2016 American Chemical Society. (d) and (e) TEM micrographs of CeO2 nanorods and nanotubes. Reprinted from ref. 70 with permission from Springer Nature. (f) SEM images and FFT pattern showing the single-crystalline nature. (g) Schematic diagram of facet oriented CeO2 NRs and its resemblance with the FESEM image (inset).76 Reproduced by permission of The Royal Society of Chemistry. | |
3.2 One-dimensional CeO2
One-dimensional (1D) nanostructures (nanowires, nanorods and nanotubes) have been intensively researched due to their fascinating physical and structural behaviour along with high chemical reactivity. Because of their high aspect ratio, they are beneficial for many potential technological applications.108 Different mediators such as mineralizers, organic solvents, and surfactants are used to kinetically control the 1D anisotropic crystal growth and other parameters like temperature, pH, concentration, etc. are also optimized for obtaining a thermodynamically favoured 1D structure.69–77,104 Zhao et al. prepared single-crystalline nanorods via the hydrothermal method (140 °C for 56 h) by using NH3·H2O as an alkaline agent without the use of any surfactant or capping agent followed by calcination.69 The study showed that the additional use of a capping agent/surfactant increases the probability of one-dimensional growth at lower temperature and consumes less time.54–60 Further, Cao et al. reported an interesting methodology (etching–dissolution–deposition) for the preparation of hollow nanotubes at low temperature. In this procedure, they first prepared CeO2 nanorods hydrothermally by using NaOH as a precipitating agent, and then they added a Ce salt precursor to the prepared CeO2 nanorod suspension under heating to obtain CeO2 nanotubes. They proposed that the surface Ce3+ underwent hydrolysis and further dissolution at the tips of the nanorods resulting in nanotube formation. Further, the redeposition and crystallization on the outer sides of the nanorods yielded CeO2 nanotubes of an average diameter of 11.8 nm, as shown in Fig. 4(d and e).70 In another work, Kuiry et al. prepared CeO2 nanotubes of diameter 40 nm and length 250 nm by using bis(2-ethylhexyl)sulfosuccinate sodium salt (AOT) as a surfactant in toluene–water solvent through the micro-emulsion technique.72 Besides, 1D CeO2 has been synthesized by using some hard templates such as AAO, ZnO, KxMnyOz, etc. as these substrates encourage 1D nucleation.73–75 For example, Wu's team first prepared K1.33Mn8O16 nanowires through the hydrothermal technique and then deposited CeO2 over them by a second hydrothermal treatment. The K1.33Mn8O16 nanowire template was instantaneously removed due to the release of an excess of HNO3 during the precipitation of CeO2 in the second hydrothermal treatment, leaving behind CeO2 nanotubes with a diameter of 90 ± 10 nm which fits with the diameter of K1.33Mn8O16 nanowires.75 Furthermore, the electrochemical deposition technique is now more popular for the growth of 1D nanostructures like nanorods and nanotubes over a conductive substrate.76,77 Lu et al. reported hexagonal CeO2 nanorods with prominent (110) exposed planes by a facile cathodic electrodeposition technique over a Ti substrate using Ce(NO3)3·6H2O, NH4Cl, and KCl chemicals at a current density of 0.5 mA cm−2. They found that the average diameter of the grown nanorods was about 200–400 nm and the length was up to 800 nm as shown in Fig. 4(f and g), while two sets of diffraction spots in the FFT pattern as shown in the inset of Fig. 4(f) confirmed the single crystalline nature with some defects.76 And Zhang et al. fabricated nanorod and nanowire CeO2 over a Cu substrate by using the same nitrate precursor at a current density of 0.44 mA cm−2 and 0.88 mA cm−2 respectively for 2 h at 70 °C. They found well-aligned CeO2 nanorods with an average diameter of about 200 nm while the diameter of nanowires was about 80 nm.77
3.3 Two-dimensional CeO2
Recently 2D materials have attracted a lot of interest because of their size-derived special properties such as high conductivity, high mobility, high mechanical strength and large spin diffusion length. Their excellent properties make them an outstanding material for many catalytic and energy applications.109 Metal oxide semiconductors, i.e. CeO2, with a cubic crystal structure have no intrinsic driving force for 2D anisotropic growth.78 So, the synthesis of 2D CeO2 is generally carried out by using a template/surfactant/mineraliser for controlling the crystal growth along two or more facets.78–83 Yu et al. synthesized CeO2 nanosheets via a two-step hydrothermal treatment. At first, the inorganic–organic Ce–EDA hybrid nanorods were prepared by using a hydrothermal reaction at 150 °C between 1,2-ethanediamine (EDA) and Ce(OAc)3. Then, the hybrid nanorods were again hydrothermally treated at 280 °C to form single-crystal-like CeOH(CO)3 nanosheets which were further calcined to obtain 2.4 nm thick ultrathin mesoporous CeO2 nanosheets as schematically represented in Fig. 5(a).79 Further, Yu and his research team described the synthesis of ultrathin ceria nanosheets by using a single surfactant/stabilizer (6-amino hexanoic acid) only. They found that the thickness of the nanosheet was approximately 2.2 nm and the lateral dimension was up to 4 mm as shown in Fig. 5(b and c). In the preparation procedure, at first small ceria nanocrystals stabilized by AHA were formed, and later these crystals were organized into ultrathin nanosheets through 2D self-assembly, followed by an in situ re-crystallization process.78 Huang et al. prepared 2D CeO2 nanosheets through electrochemical anodic deposition methods (0.2 mA cm−2 for 60 min at 70 °C) over a substrate by using cerous nitrate and NH4NO3.80 Likewise, Wang et al. reported ultrathin ceria nanoplates via a simple and robust solution-phase synthesis method where a soft surfactant (oleic acid or oleylamine) was used in the presence of a mineraliser (sodium diphosphate or sodium oleate) to prepare highly efficient ceria nanoplates. They concluded that thickness and morphology solely depend upon the reaction time. And the nanoplates possessed a high surface to volume ratio and the desirable high OSC(100) plane.81
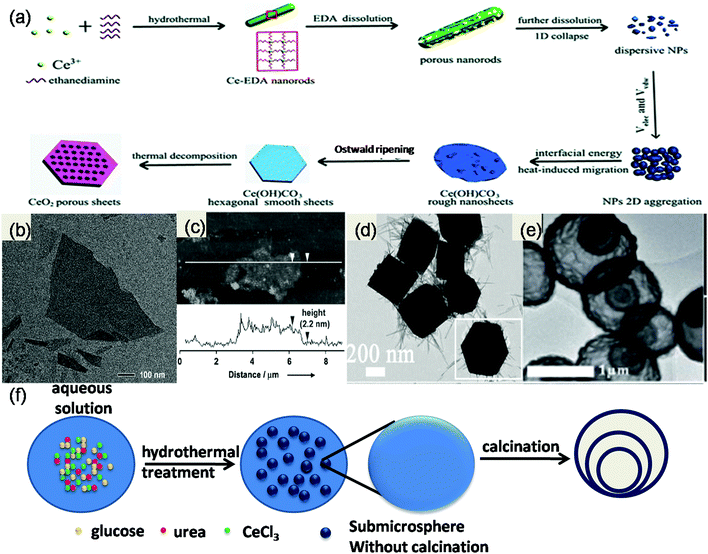 |
| Fig. 5 (a) Structure evolution mechanism of porous CeO2 sheets.79 Reproduced by permission of The Royal Society of Chemistry. (b) and (c) Ultrathin CeO2 nanosheets with AFM showing the single-crystalline nature. Reprinted from ref. 78 with permission from Wiley. (d) TEM micrographs of hexahedron prism anchored octahedral CeO2. Reprinted with permission from ref. 84, copyright 2015 American Chemical Society. (e) TEM micrographs of multishell CeO2 and (f) complete synthetic route for preparation of this.91 Reproduced by permission of The Royal Society of Chemistry. | |
3.4 Three dimensional CeO2
The three-dimensional nanoarchitecture with a large aspect ratio, high porosity, high surface area and high oxygen storage capacity has emerged as a promising candidate for different types of catalytic oxidation reactions.110 Generally, template or surfactant-assisted methods are applied to synthesize 3D (prism, octahedron, ordered mesoporous, sphere, hollow architecture, etc.) CeO2 materials.84–91 Li et al. synthesized hexahedron prism anchored octahedral 3D CeO2 by using phosphate ions as a mineraliser through a simple hydrothermal method, where the nanorods emerged out from the tinctured octahedra as shown in Fig. 5(d). The unique hierarchical 3D structure provides selected redox facets and spatial charge separation sites, which are quite effective in enhancing the photocatalytic activity.84 A pillow like CeO2 microstructure of 10 μm length and 4 μm width was prepared by Han and co-workers by a facile solvothermal method by using a cerium salt and ammonium nitrate as starting materials at pH 10. The obtained CeO2 pillows have a BET surface area of 191 m2 g−1 and a pore diameter ranging from 2 to 10 nm.85 Through a novel hydrothermal method followed by a controlled calcination method, Sun et al. prepared monodisperse flowerlike CeO2 microspheres containing a micro/nanostructure. Because of the simultaneous polymerization–precipitation process open three-dimensional (3D) porous and hollow structures were formed consisting of nanosheets as the petals with an average thickness of about 20 nm.86–88 Besides, mesoporous ceria attracts a lot of attention as a versatile catalyst and support specimen because of its high surface area and porosity, because of which it can increase the dispersion of active secondary components. Adding more to it, Ji et al. reported an ordered mesoporous (OM) CeO2 by using cubic Ia3d mesoporous MCM-48 silica where the obtained OM structure CeO2 achieved very high efficiency towards photocatalytic dye degradation.89 Further, hollow core–shell 3D structures are also investigated due to their peculiar advantageous properties. In this direction, Fang et al. communicated a variety of hollow 3D structures of CeO2 like cubic, polyhedral and spherical through the template-engaged (Cu2O) coordinating etching technique.90 However, template-free synthesis is more convenient and easy nowadays. Qi and team prepared multi-shelled CeO2 hollow microspheres (Fig. 5(e)) by a simple hydrothermal method followed by calcination without using any hard or soft template. Firstly, the reaction precursor glucose was subjected to a condensation and polymerization process under hydrothermal conditions resulting in carbon microspheres. Subsequently, Ce3+ ions got attached to the deprotonated functional groups inside the carbon microspheres in an alkaline environment. Finally, the as-formed carbon microspheres were used as sacrificial templates to obtain triple-shelled CeO2 hollow microspheres via calcination in air as shown in Fig. 5(f).91
3.5 Nanostructured CeO2 based materials
Considering the popularity of nanostructured CeO2 in different photocatalytic applications, a wide variety of facet based CeO2 heterojunctional systems such as CeO2–metal oxide, CeO2–metal sulphide/selenide, CeO2–metal phosphide, CeO2–carbonaceous materials, CeO2–metals,111–133etc. have been synthesized and utilized in different energy and environmental applications thoroughly. More importantly, manipulation of the morphology of non-CeO2 based components is very essential regarding the design of efficient interfaced nanostructured CeO2 based composite photocatalysts. This is because the dimensionality of the other units and interfacial contact between them mainly determine the activity of the composites.134 On the basis of dimensionality and size, CeO2 based composite materials are classified into 0D–2D, 0D–1D, 1D–2D, 2D–2D, 0D–3D, etc. as schematically represented in Fig. 6(a). Their synthetic procedure follows either in situ growth methods or ex situ assembly approaches or the one-pot method. But most often two or more methods are combined in these multistep reaction procedures to prepare different nanostructured CeO2 based materials. For example, small-sized 2D nanosheets can grow vertically or wrap on the surface of relatively large 1D CeO2NRs. Du et al. reported small MnO2 nanosheets decorated over CeO2 nanowires via an in situ growth method. They reported a two-step hydrothermal method in which first CeO2 nanowires were synthesized from Ce(OH)3 nuclei, and then these nanowires were dispersed in KMnO4 solution to obtain the 2D–1D heterostructure.111 In another method reported by Jiang et al., CeO2 nanorods were derived from the Ce-MOF precursor. As shown in Fig. 6(b and c), ZnIn2S4 nanosheets were beautifully anchored on top of CeO2 nanorods. They carried out the beautiful 2D–1D heterostructure synthesis via a low-temperature solvothermal procedure. As shown in the HRTEM image, a beautiful interface is formed between the 111 plane of CeO2 and the 102 plane of ZnIn2S4 forming a facet merged interface.112 Additionally, CeO2 nanosheets decorated over Au nanorods were reported by Jia et al., where simple site-selective growth of crystalline CeO2 nanosheets on the end of AuNRs was observed resulting in the formation of a 2D–1D heterostructure. The AuNR capped CeO2 heterostructure was prepared as follows: at first the AuNRs were prepared by using CTAB as a surfactant. Then the nanorods were re-dispersed again in CTAB solution and K2PtCl4 was subsequently added to the above solution followed by the addition of a cerium precursor at moderate temperature. The design of the site-selective heterostructure was based on the following points: (i) the AuNRs have a different crystal structure and curvature difference (at the side and the end), so the less dense molecular chains of CTAB at the ends offer less steric hindrance for the other species to reach the AuNR than those of the sides, and hence PtCl42− ions preferentially adsorb at the two ends of AuNRs as shown in Fig. 6(d), (ii) further the ceria precursor is rapidly hydrolyzed into Ce(OH)3 at elevated temperature and is easily oxidized by the pre-adsorbed PtCl42− to produce the nuclei for the further growth of CeO2. The preferential adsorption of PtCl42− and the autoredox reaction between PtCl42− and Ce(OH)3 result in site-selective nucleation and growth of CeO2 nanosheets at the end of the Au nanorod heteronanostructure as shown in the TEM images in Fig. 6(e and f).113 Further, Feng and co-workers prepared a FeOOH/CeO2 heterolayered nanotube (1D–1D) over Ni foam through the electrodeposition technique by using ZnO as a hard template. First, they deposited the CeO2 layer over the ZnO NRs placed vertically over the nickel foam substrate, and then they deposited the FeOOH layer over the top of CeO2 followed by removal of the ZnO template to yield a core–shell type 1D–1D heterostructure.114
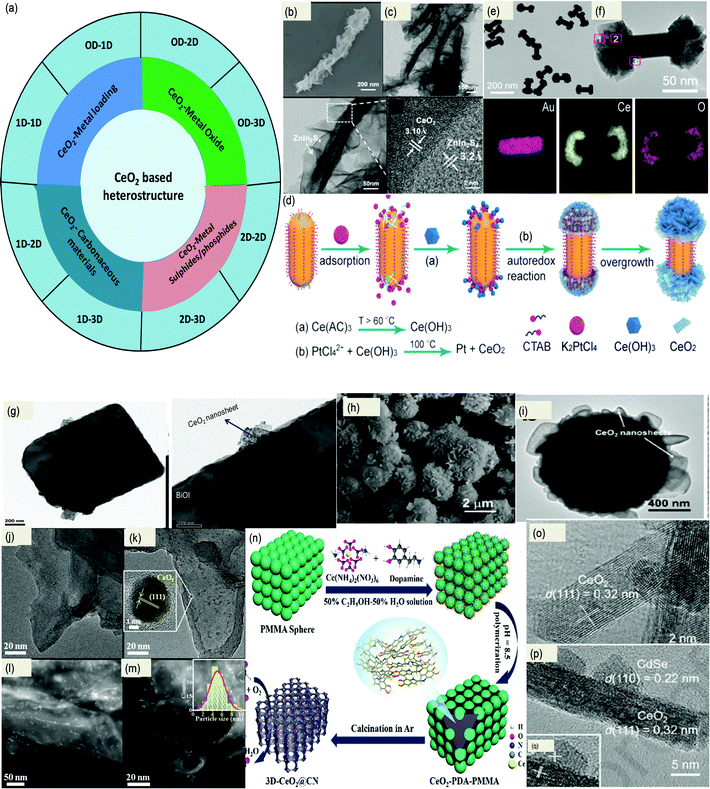 |
| Fig. 6 (a) Schematic representation of different types of CeO2 based heterostructure. (b) and (c) FESEM and TEM (HRTEM) images of the CeO2–ZnIn2S4 heterostructure showing the 2D–1D interface between 111 (CeO2) and 102 (ZnIn2S4) planes. Reprinted from ref. 112 with permission from Elsevier. (d) Schematic illustration of the synthesis process of a Au/end-CeO2 nanostructure. (e and f) TEM, HAADF-STEM and the corresponding elemental map images of the Au/end-CeO2 nanostructures. Reprinted with permission from ref. 113. (g) TEM images showing the face-to face BiOI and CeO2 2D–2D heterostructure. Reprinted with permission from ref. 117, copyright 2019, 2018 American Chemical Society. (h and i) SEM and TEM images of the 3D–2D heterostructure consisting of Bi2O3 and CeO2. Reprinted from ref. 119 with permission from Elsevier. (j and k) TEM and HRTEM images showing the uniform distribution of CeO2 QDs over g-C3N4 and (l and m) corresponding HAADF images and particle size distribution. Reprinted from ref. 123 with permission from Wiley. (n) Schematic representation of the synthesis procedure of 0D–3D CeO2@CN.128 Reproduced by permission of The Royal Society of Chemistry. (o–q) TEM and HRTEM images showing the CdSe QD decorated CeO2 NRs with lattice fringes and a clear interface. Reprinted from ref. 131 with permission from Elsevier. | |
Compared to other heterostructures, 2D–2D materials possess maximum hetero-interfaces and strong physical and electronic coupling effects, which further facilitate the anti-recombination process and thus contribute to high photocatalytic performance. For example Wen et al. reported a face to face heterostructure, where they first prepared CeO2 microplates via a sol–gel auto-combustion method and then BiOBr nanosheets were loaded onto the surface of CeO2 with the assistance of ethylene glycol by a facile deposition–precipitation technique.116 Further, Parida and co-workers prepared a series of 2D/2D-BiOI/CeO2 nanocomposites through a simple precipitation technique followed by ex situ coupling. Initially, they prepared a CeO2 nanosheet coupled with a dodecyl sulphide ion layer and then sonicated it in formamide solution. In this process the CeO2 nanosheet got free and rectangular BiOI microplates were introduced onto this resulting in a beautiful face to face 2D–2D heterostructure as shown in Fig. 6(g).117 Moreover, Zhang et al. delineated a one-pot synthetic strategy to produce vertical 2D–2D Co3O4–CeO2 nanosheets over Ti mesh via the hydrothermal method; then the samples were further selectively calcined in the presence of sodium hypophosphite to produce an efficient CoP–CeO2/Ti hybrid.118 Furthermore, Yang et al. prepared a 3D–2D core–shell type Bi2O3@CeO2 heterostructure through a simple two-step hydrothermal process followed by high-temperature calcination. They observed that the high-temperature treatment facilitates the outward diffusion of the interior Bi2O3 leading to the formation of a more prominent 3D–2D heterojunction effective for photocatalysis. As shown in Fig. 6(h and i) 2D CeO2 nanosheets are very beautifully attached to the Bi2O3 sphere leading to an efficient heterostructure.119 Gong et al. prepared a CeO2@MoS2 (3D–2D) hybrid heterostructure via a facile two-step wet chemistry strategy. At first, they prepared CeO2 hollow spheres by a template-assisted method and then few-layered ultrathin MoS2 nanosheets were deposited on the hollow CeO2 spheres through a hydrothermal process.120
Furthermore, CeO2 QDs are in the limelight because of their outstanding size-derived properties and visible light absorbing nature; however, the associated drawbacks like self-aggregation and high charge carrier recombination restrict their photoactivity. So CeO2 QDs are often combined with 1D nanorods,121,122 2D nanosheets123–126 and also with 3D127–129 structured materials to improve their catalytic activity. Li et al. prepared a 0D–1D heterostructure where CeO2 QDs were decorated over sulphur doped carbon nitride nanotubes through an in situ deposition–precipitation technique without using any surfactant or template. They found that the CeO2 QDs are distributed inside as well as outside of S–CN nanotubes.121 Likewise, Xia and the group proposed a strategy for the preparation of CeO2 QDs on a template. They prepared quantum sized nanoceria on to the surface of polymeric carbon nitride nanosheets (PCN) without using for growth inhibitor essential for QDs preparation. The synthetic strategy they reported is as follows: the cerium precursor was mixed with the suspension of PCN; here Ce ions adsorbed on the surface of PCN because a strong coulomb interaction existed between the positively charged Ce ion and the lone pair electron of nitrogen atoms. Upon further heating, the adsorbed Ce ions hydrolyzed with water in the presence of DMF and further calcination yielded QDs of CeO2 uniformly decorated over PCN as shown in Fig. 6(j and k). It was observed from HAADF-TEM images as shown in Fig. 6(l and m) that 5 nm sized CeO2 QDs are uniformly anchored over PCN nanosheets producing a 0D–2D heterojunction.123 Again, Zhang et al. reported a 0D–2D heterostructure between 10 nm CeO2 nanoparticles and ZnIn2S4 nanosheets. At first, through a simple solvothermal method, the ZnIn2S4 nanosheet was prepared and the Ce3+ precursor was added into the alcohol–water mixed solution containing ZnIn2S4 to obtain the heterostructure. They termed the 0D CeO2 nanoparticle on the 2D ZnIn2S4 nanosheets as a “chess piece” on the “checkerboard” type morphology of CeO2.124 Further, Luo et al. reported an interesting synthesis procedure for the in situ generation of CeO2 QDs within the pores of 3D-nitrogen doped porous carbon. In the first step, self-assembled PMMA colloidal spheres were prepared and the voids were filled with ceric ammonium nitrate and dopamine solution. At pH 8.5, dopamine underwent self-polymerization and got adhered to the PMMA scaffold and in the meantime the cerium precursor was chelated by the same as shown in Fig. 6(n). Further, upon calcination the hard template (PMMA) was removed to generate the 0D–3D heterostructure.128
Besides these, 0D QD decorated CeO2 nanostructures have gained a huge positive response; for example, quantum sized particles are decorated over nanorods, nanosheets, and nanoflowers of CeO2 giving rise to various heterointerfaces with noticeable performance.130–133 In this direction, Ma et al. reported a 0D–1D heterostructure containing CdS QDs over CeO2 NRs through a solvothermal technique.130 In addition to this work, the team also studied a 0D–1D CdSe QDs–CeO2 nanorod heterostructure as shown in Fig. 6(o) through a wet chemistry method (details discussed below). They first dispersed CeO2 nanorods and then added a Cd salt precursor and a capping agent, i.e.L-cysteine, followed by dropwise addition of a Se precursor to obtain the heterostructure. From the TEM micrographs (Fig. 6(p and q)) a clear interface between 0D CdSe QDs(110) with 1D CeO2(111) nanorods is seen.131 Further Ma et al. also reported another heterostructure, i.e. CdS QD decorated Fe–CeO2 based nanoflowers (3D), through a simple solvothermal method in the presence of dodecanethiol and oleylamine.133
In the past decade, nanostructured CeO2 and CeO2 based materials have received increasing attention as photocatalysts for pollutant degradation and solar fuel generation because of the unique properties such as biocompatibility, chemical inertness, strong oxidizing capability, higher charge separation and transportation efficiency and most importantly absorption of more photonic energy. So in the following section, we have summarized different photocatalytic applications of CeO2 and CeO2 based materials, with a thorough description of the theoretical concept along with some examples.
4. Photocatalytic water reduction and oxidation
Water splitting is a process of breaking water into its elemental constituents such as di-hydrogen and oxygen. It is a very promising approach but the process is a multielectron, endothermic uphill reaction that requires a high positive Gibb's free energy. Thus specific energy such as solar light is required to cleave a high energy HOH bond. Generally, 2.458 eV energy is required to split one water molecule to generate one hydrogen molecule, which works under 1.23 V potential difference for the displacement of two electrons. So a photocatalyst that has the ability to decrease the overpotential required to carry out the reaction is the need of the hour.135–140
4.1 Hydrogen evolution
The hydrogen evolution reaction is a major half-reaction of water splitting which generates sustainable clean H2 energy. To replace fossil fuels, different fuels have been investigated recently, among which hydrogen has emerged as an appealing clean and green energy resource for future generation energy systems. It has a high energy density as compared to other hydrocarbon fuels and doesn’t release any harmful toxic gases into the environment after its use. Thus, it can minimize pollution as well as reduce greenhouse gas emissions. Currently, most of the H2 is prepared from either the hydrocarbon steam reforming process or the coal gasification process, which are high energy-consuming processes and require very high temperature processing. So its generation by utilising solar energy through water splitting is the most suitable and promising approach.135–157 In this regard CeO2 and CeO2 based materials are explored both theoretically and practically towards the photocatalytic H2 evolution reaction as summarized in Table 1.
Table 1 Complete results of the H2 evolution performance of CeO2 and CeO2 based heterostructures
Materials |
Light source |
Experimental conditions |
Activity |
Ref. |
Defective CeO2 NRs |
300 W Xe lamp |
Na2S–Na2SO3 sacrificial agent |
5.020 μmol h−1 g−1 |
71
|
Single crystalline hexagonal CeO2 NRs(110) |
300 W Xe lamp |
Na2S–Na2SO3 sacrificial agent |
741 μmol h−1 g−1 |
76
|
Au@CeO2 |
50 W Xe lamp |
Methanol sacrificial agent, Pt co-catalyst |
8.7 μmol h−1 mg−1 |
162
|
Au@CeO2 |
300 W Xe lamp (λ > 420 nm) |
Methanol sacrificial agent |
4.05 μmol mg−1 h−1 |
163
|
CdS QDs/CeO2NRs |
300 W Xe lamp (λ > 300 nm) |
Na2S–Na2SO3 sacrificial agent |
101.12 μmol h−1 g−1 |
130
|
CdSe QDs/CeO2NRs 111 facet |
300 W Xe lamp (λ > 300 nm) |
Na2S–Na2SO3 sacrificial agent |
283.32 μmol h−1 g−1 |
131
|
CdS/CeO2 |
300 W Xe lamp (λ > 420 nm) |
Lactic acid |
8.4 mmol h−1 g−1 |
165
|
Mn0.2Cd0.8S QDs/2D CeO2 |
Xenon lamp with a power of 5 W, λ > 400 nm |
Na2S–Na2SO3 sacrificial agent |
8.73 mmol g−1 h−1 |
166
|
MoS2(2 wt%)–CeO2 |
150 W Xe lamp |
Methanol sacrificial agent |
2.542 mmol h−1 g−1 |
167
|
1D/2D-CeO2/ZnIn2S4 |
300 W Xe lamp AM 1.5G filter |
50 μL of BA was dispersed in 5 mL of acetonitrile |
1496.6 μmol gcat−1 h−1 |
112
|
0D/2D CeO2/ZnIn2S4 |
300 W Xe lamp (λ > 420 nm) |
Na2S–Na2SO3 sacrificial agent |
847.42 μmol h−1 g−1 |
124
|
SnS2–Ce2S3–CeO2 |
300 W Xe lamp AM 1.5G filter |
TEOA |
240 μmol g−1 h−1 |
168
|
CeO2/ZnS–CuS |
300 W Xe lamp |
Methanol sacrificial agent |
13.47 mmol g−1 h−1 |
169
|
CeO2 QDs/carbon |
300 W Xe lamp |
Methanol sacrificial agent |
582 μmol g−1 h−1 |
129
|
CeO2@N,S-C |
300 W Xe lamp |
TEOA |
555 μmol h−1 g−1 |
170
|
CeO2/g-C3N4 |
300 W Xe lamp (λ > 420 nm) |
TEOA, 1.5 wt% Pt |
0.83 mmol h−1 g−1 |
171
|
c-CeO2(100) and g-C3N4 |
300 W Xe lamp (λ > 420 nm) |
TEOA, 3 wt% Pt |
860 μmol h−1 g−1 |
173
|
rCN{110} > cCN{100} > oCN{111} |
300 W Xe lamp (λ > 420 nm) |
TEOA, 3 wt% Pt |
rCN – 1100 μmol h−1 g−1 |
174
|
CeO2 QD-S-doped g-C3N4 NR |
300 W Xe lamp (λ > 420 nm) |
TEOA, 1 wt% Pt |
2923.8 μmol h−1 g−1 |
121
|
CeO2/ZnONTs FTO |
300 W Xe lamp |
Na2S–Na2SO3 sacrificial agent |
2.7 μmol cm−2 h−1 |
175
|
CeO2–ZrO2 |
300 W Xe lamp |
Na2S–Na2SO3 sacrificial agent |
≈4 μmol g−1 h−1 |
176
|
W18O49/CeO2 |
300 W Xe lamp (λ > 400 nm) |
TEOA |
0.2061 mmol g−1 h−1 |
177
|
MOF-derived NiO/CeO2 |
300 W Xe lamp |
Methanol sacrificial agent |
29.6 μmol h−1 g−1 |
178
|
CeO2/CeVO4/V2O5 |
300 W Xe lamp |
MB aqueous solution (50 mg L−1) |
47.42 μmol g−1 h−1 |
179
|
CeO2–MoS2/g-C3N4 as 0D/2D |
UV light emitting diodes (UV-LEDs) (λ > 420 nm) |
Na2S–Na2SO3 sacrificial agent |
1308 μmol g−1 h−1 |
139
|
(110) CeO2-IT/2HMoS2 |
UV-light emitting diodes |
Na2S–Na2SO3 sacrificial agent |
73.1 μmol h−1 |
180
|
CeO2–CuO QDs/graphene |
300 W Xe lamp |
Methanol sacrificial agent |
2481 μmol g−1 h−1 |
181
|
3DOM Ag/CeO2–ZrO2 |
300 W Xe lamp |
Na2S–Na2SO3 sacrificial agent |
6.62 μmol h−1 |
182
|
Ag–ZnO–CeO2 |
300 W Xe lamp |
Glycerol |
18 345 μmol g−1 h−1 |
183
|
Rod–rod |
AM 1.5G filter |
MoS2/CeO2–Co3O4 |
5 W LED lamp (λ > 420 nm) |
TEOA |
221 μmol in 3 h |
184
|
Ni–P/CeO2–TiO2 |
Solar stimulator (1 sun) |
— |
1300 μmol/5 h |
185
|
Water splitting over ceria includes 2 major steps: the first is water hydroxylation and the second is H2 formation. First water molecularly adsorbs by the oxygen atom on the top of the Ce atom of CeO2(111), while water adsorbs dissociatively near the oxygen vacancy of defect enriched CeO2(111) and in both cases one H of water bonds with the surface oxygen atom. Then water dissociation into hydroxyl takes place and the surface vacancies facilitate the water dissociation step. And finally hydroxyl decomposition occurs and liberates H2 through an asymmetric process, which involves oxidation of Ce3+ to Ce4+.158–160 Further, in the case of excessive hydroxylated CeO2, water hydroxylation and liberation of H2 might involve a new intermediate, i.e. Ce–H. It is found that the OH species plays a very important role in water splitting and its formation and H2 liberation mainly depend upon the surface vacancy.160 Further Mullins et al. did theoretical calculations over both planes of reduced CeO2(100) and CeO2(111). They found that dissociation is much more favorable on CeO2(100) than on CeO2(111); however, in the reduced state H2 generation occurs much more on CeO2(111) than on the former due to the different adsorption sites. On reduced CeO2−x(111) the dissociated water easily reoxidizes the ceria substrate, liberating H2.161 In accordance with the above prediction different CeO2 based materials were explored in the water splitting reaction as follows. Lu et al. synthesized single crystalline hexagonal CeO2 NRs, directly grown on Ti substrates with highly exposed (110) planes, which showed significant photocatalytic activity for hydrogen evolution (741 μmol g−1) in Na2S–Na2SO3 solution. They also tested the hydrogen evolution activity in methanol as a scavenger, where CeO2 NRs exhibited a higher activity than commercial CeO2, CdS as well as P25 due to the presence of exposed 110 active planes; however the yield rate was one fourth of that obtained with the sodium sulphide/sulphite scavenger because of the special redox capacity of the latter. Moreover, they observed a red shift in the absorption edge of CeO2 NRs due to the presence of Ce3+ defects, hence harvesting more visible light which may be another cause of enhanced activity, and in addition the presence of Ce3+ acted as an intermediate medium which accelerated the hole consumption of the sacrificial agent, hence enhancing the availability of reducing electrons for the water reduction reaction.76 Further, Dong et al. reported another defective CeO2 NR towards photocatalytic hydrogen evolution. They prepared high surface area (65.26 m2 g−1) CeO2 NRs by a simple hydrothermal method with a higher percentage of Ce3+ (22.53%) and oxygen vacancies (0.74).71 The as-prepared nanorods displayed hydrogen production at a rate of 5.020 mmol g−1 h−1 under solar light irradiation, and the enhanced activity was attributed to the favourable band structure, which enabled the effective photonic energy absorption along with the redox shuttle of Ce3+ to Ce4+, which retarded the charge recombination.
However nanostructured CeO2 alone doesn’t give appreciable results as compared to others, because of its high recombination rate of photogenerated excitons and the low ability for solar light absorption. Hence, CeO2 is often combined with noble metals162,163 or other photocatalysts/co-catalysts.164–169 Dao et al. developed a novel route for the synthesis of the Au@CeO2 core–shell functionalized with Pt. The Au@CeO2 nanostructure photocatalyst was further tested for the H2 evolution reaction under visible light and it exhibited H2 production efficiency at a rate of 8.7 μmol h−1 mg−1. The enhanced activity was attributed to the SPR effect of Au, which was helpful for visible light absorption and the corresponding charge carrier generation. Further, the photogenerated hot electrons travel via direct injection and cross tunnelling into the conduction band of CeO2, where they travel to the Pt co-catalyst and reduce the adsorbed H+.162 Further, a number of modified nanostructured CeO2 materials in combination with other low bandgap materials such as metal sulphides112,124,130,131,164–169 have been reported compared to neat CeO2. You et al. synthesized a type II heterojunction composite between CdS NPs and CeO2 NRs and tested its photocatalytic activity towards the hydrogen evolution reaction. Under visible-light irradiation (λ > 420 nm) the as-prepared 1
:
1 ratio CdS/CeO2 achieved an optimum H2 evolution rate of 8.4 mmol h−1 g−1, with a high apparent quantum yield (AQY) around 11.2%. The enhanced activity can be attributed to the promoted lifetime and separation efficiency at the heterojunction interface.165 Recently, Ma et al. reported the H2 evolution activity of CdS QD decorated CeO2 NRs and observed an optimum rate of H2 production, i.e. 101.12 μmol h−1 g−1 (3 atm% CdS) which was 45 times higher than that of neat CeO2 NRs under light irradiation (300 W Xe lamp λ > 300 nm) as shown in Fig. 7(a). The enhanced photoactivity for a prolonged period of 60 h was mainly attributed to higher light absorption ability, enhanced charge transfer and separation efficiency and greater lifetime of photogenerated charge carriers due to the presence of the CdS component. The efficient charge transfer mechanism was found to be of the Z-scheme type instead of the heterojunction type charge transfer mechanism as shown in Fig. 7(b) where higher reducible electrons resided at the conduction band of CdS while higher oxidizable holes were quenched by the sulphide/sulphite scavenger over the CeO2 surface. They represented solid evidence of the Z-scheme, where the catalyst responds to both hydroxyl and superoxide radicals in the spin trapping ESR experiment as shown in Fig. 7(c and d) despite the incapability of neat CdS for hydroxyl radical generation, thereby proving that the photogenerated charge separation occurred through the Z-scheme rather than the conventional double charge transfer mechanism.130 Moreover, in addition to CdS, Ma et al. reported CdSe QD decorated CeO2 NRs for photocatalytic hydrogen evolution and they found a rate of 283.32 μmol h−1 g−1, which is higher than that of the neat component. Further through DFT analysis they found that the 111 exposed facet interwinged heterostructure increases the water adsorption ability and creates a lower energy barrier for water dissociation, while the same Z-scheme mechanism was followed by this heterostructure also.131 Wang et al. reported another 0D–2D heterostructure where Mn0.2Cd0.8S quantum dots were decorated over a 2D CeO2 building block and gave rise to a perfect 0D(101)–2D(111) interface. They used different types of scavengers such as methanol, lactic acid and the sulphide + sulphite mixture and observed that the catalytic efficiency was continuously decreased in methanol and lactic acid solution, while the activity was retained in the sulphide+ sulphite solution. As the photocatalyst contains metal sulphide, upon light irradiation the metal sulphide corrodes down which retards its long term activity in the methanol scavenger solution. Hence, they observed that Mn0.2Cd0.8S–CeO2 exhibited a maximum hydrogen production rate of 8.73 mmol g−1 h−1 in the sulphite/sulphide scavenger solution with an apparent quantum efficiency greater than 2 at 400 nm. The introduction of CeO2 prolonged the lifetime of the photogenerated charge pair through the Z-scheme, and thereby increased the charge separation efficiency and lowered the charge transfer resistance.166 Recently, 1D/2D and 0D/2D heterostructures between CeO2 and ZnIn2S4 have gathered attention, as ZnIn2S4 can easily be tuned to two dimensional nanosheet materials.112,124 Jiang et al. prepared ZnIn2S4 nanosheets which were grown in situ on the surfaces of CeO2 nanorods. The 2D/1D composites exhibited a hydrogen production yield of 1496.6 μmol gcat−1 h−1 in tandem with the selective oxidation of aromatic alcohol, due to the synergistic advantage of both CeO2 and ZnIn2S4. The combined effect of both the moieties especially that of the excellent ZnIn2S4 nanosheets is helpful for visible-light absorption and CeO2 nanorod enabled one directional charge transport contributes to higher efficiency of the composite. Moreover, intimate interfacial contacts are formed in the solid Z-scheme heterostructures, which accelerate the charge transfer and separation while retaining the strong reducibility of electrons in the CB of ZnIn2S4 nanosheets and the oxidizability of holes in the VB of CeO2 nanorods.112 Moreover, in situ-MOF derived CeO2 based materials have also come to light.169,170 With respect to this, Wang et al. prepared a CeO2–ZnO nanoheterostructure from a Ce-doped ZIF-8 MOF as the precursor. Further through an in situ vulcanization process the above heterostructure was converted to CeO2–ZnS, which showed the best photocatalytic hydrogen production performance. However, further loading of the CuS co-catalyst via an in situ cation exchange method led to a hydrogen production rate of 13.47 mmol g−1 h−1 which was about 1.4 times that of CeO2/ZnS.169
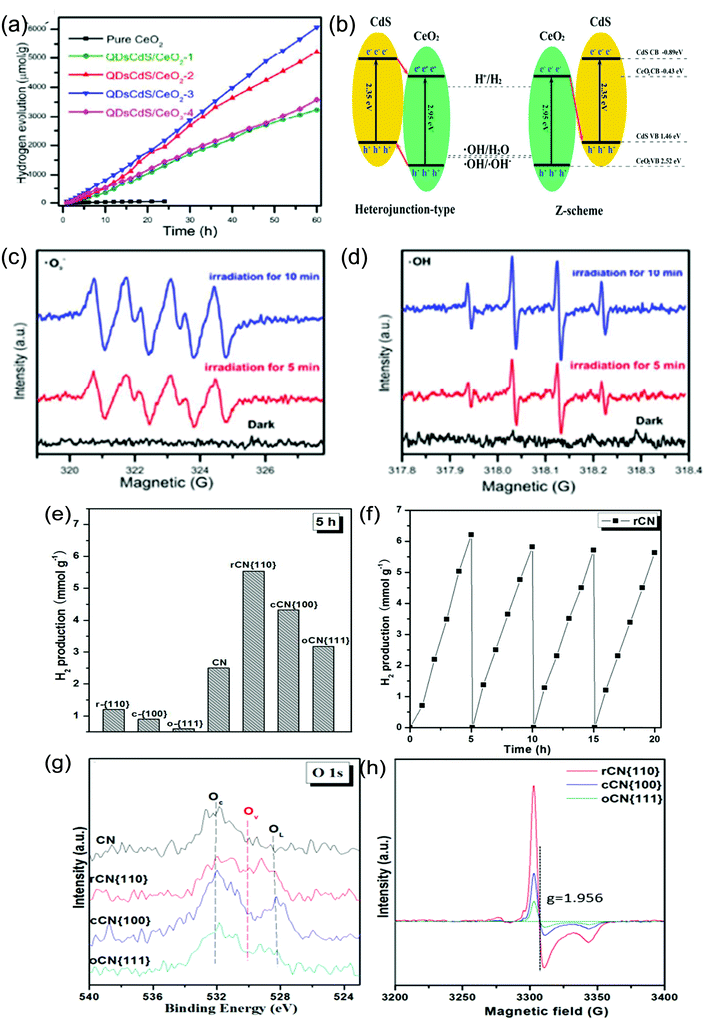 |
| Fig. 7 (a) Photocatalytic hydrogen evolution over CeO2 nanorods and CdS QD decorated CeO2 in 60 h. (b) The possible photocatalytic mechanism exploration of the heterojunction-type and Z-scheme over the CdS QDs/CeO2 nanorod 0D/1D heterostructures. (c and d) The DMPO spin-trapping ESR spectra of CdS QDs/CeO2-3 composites. Reprinted with permission from ref. 130, copyright 2018 American Chemical Society. (e) H2 production of CeO2 nanorods, cubes, octahedra, CN, rCN, cCN, and oCN composites under visible light irradiation after 5 h. (f) Reusability test of the rCN composite. (g) O 1s XPS spectra of CN, rCN, cCN, and oCN. (h) ESR results of rCN, cCN, and oCN. Reprinted from ref. 174 with permission from Elsevier. | |
Nanostructured CeO2 has also been modified with carbon-based materials such as carbon, graphene, and g-C3N4,121,129,171–174 and it was found to show better photocatalytic activity than neat CeO2. For example, recently Qian et al. reported a new biotemplate oriented synthetic strategy for the synthesis of zero dimensional CeO2 QDs embedded in a porous carbon tube. The biotemplate carbonization process provided a layer matrix and a small amount of oxygen for QD deposition and growth. The obtained 3D porous CeO2 QDs/carbon exhibited the highest H2 evolution rate which was 5.5 times higher than that of the bulk CeO2 due to the presence of defective CeO2 along with its quantum dot property. Upon visible light irradiation, the photogenerated electrons and holes were produced at the CeO2 surface, where the electrons drifted towards the carbon skeleton via the Ce–C bond, thereby accelerating charge pair separation as well as effective utilization of these energetic electrons towards the water reduction reaction.129 Next, Zou et al. synthesized CeO2 nanocubes decorated over g-C3N4 nanosheets and described the interaction through hydrogen bonds and a p–π hybrid between c-CeO2(100) and g-C3N4. Further, the H2 evolution activity was determined to be 4300 μmol g−1 for 5 h illumination, which was higher than that of pristine CeO2, g-C3N4 and irregular CeO2 nanoparticles/g-C3N4. The same group performed another extensive study and compared the hydrogen evolution activity of morphology dependent (cube, rod and octahedral) CeO2 coupled g-C3N4. They found that the CeO2 nanorod with an exposed 110 facet when combined with the g-C3N4 nanosheet gave a much higher hydrogen production value than the others because of the stronger built-in interface. In brief, they prepared morphology tunable CeO2 with (110), (100), and (111) exposed planes and found that the crystal planes of CeO2 greatly alter some of the characteristics in the CeO2 and g-C3N4 heterostructure. They observed that 110 exposed CeO2 nanorod modified g-C3N4 exhibited higher photocatalytic hydrogen production efficiency than other CeO2 modified samples as shown in Fig. 7(e) and the order was found to be CeO2 NR(110)/gC3N4 > CeO2 Cube(100)/g-C3N4 > CeO2 octahedron (111)/g-C3N4 > g-C3N4. And also the optimally prepared catalyst retained its photoactivity in more than one cycle for up to 20 h (Fig. 7(f)). Furthermore, as shown in Fig. 7(g and h), from the XPS and ESR analysis it was found that the 110 rod shaped CeO2 possesses more oxygen vacancies and higher Ce3+ concentration. Hence, the surface oxygen vacancies played a major role in determining the photoactivity. Further from DFT analysis, it was found that nanorod decorated g-C3N4 showed significant charge transport and separation at the interface, as the charge was increased in the C and N of g-C3N4 while it was decreased in the Ce atom of CeO2, which led to better charge separation under light illumination than in the other materials.173,174
Further, other metal oxide combined CeO2 nanostructures have also been explored in the field of the photocatalytic hydrogen evolution reaction.175–179 For example, Zeng et al. reported a CeO2/ZnONTs FTO heterojunction through a two-step electrodeposition technique and tested its photocatalytic H2 evolution activity which was found to be 2.7 μmol cm−2 h−1. The higher photocatalytic activity was attributed to a larger surface area, more active sites and effective electron–hole separation at the interface between two metal oxides.175 Recently Shen et al. prepared a novel heterojunction photocatalyst W18O49/CeO2via a hydrothermal strategy. Under visible light irradiation, the oxygen vacancy enriched heterostructure exhibited the highest hydrogen production efficiency of about 0.2061 mmol g−1 h−1 which was 1.93 times higher than that of pure CeO2. According to the DFT calculations, the authors claimed that between CeO2 and W18O49, the Z-scheme charge transfer mechanism occurred, which retarded the efficient charge recombination, and hence electrons accumulated over the CeO2 surface and reacted with H+ to yield H2.177 Apart from all of these, nanostructured CeO2 is also combined with more than one photocatalyst or co-catalyst towards the productive hydrogen evolution reaction.180–185
4.2 Oxygen evolution
The oxygen evolution reaction is another half reaction of water splitting, where water is oxidized to generate oxygen gas in the presence of a catalyst. It is a core process of many energy storage and conversion systems, such as solar cells, fuel cells, metal–air batteries, etc.186–197 And for the water electrolysis to produce sustainable H2 energy, water oxidation is a preliminary reaction. It is a multi-electron and multi-proton reaction, and is therefore kinetically as well as energetically a difficult/sluggish process. This is because for the generation of one molecule of O2, four protons and four electrons are required. In order to oxidize two water molecules simultaneously, the catalyst must be able to store four electrons–holes close together.197 So a catalyst which can absorb water and simultaneously oxidize two water molecules is a promising one. Since CeO2 has the ability to carry out water oxidation smoothly, a number of CeO2 based materials have been explored in this field which are summarized below.
In general water oxidation over CeO2 involves two major steps as confirmed from DFT studies. The first step is the sequential dehydrogenation of water, i.e. H2O → OH + H → O + 2H. In particular, on CeO2(110), H2O is adsorbed on the top Ce site and the adsorbed H2O dehydrogenates to OH and H. Then the formed OH group dissociates into O and H, as the H migrates to the neighbouring top O site. Further, the formation of O2 requires an additional step, in which an additional H2O further dehydrogenates to form the second OH and H. The second OH then combines with the O from the first H2O molecule to form OOH. The formed OOH species binds to the Ce site in the final state. Meanwhile, O2 is generated by the dehydrogenation of OOH via O–H bond scission.103 Zhang et al. studied the theoretical concept behind the water oxidation process involving normal and defective CeO2(110) nanorods. According to DFT, they found that the presence of oxygen vacancies on CeO2(110) effectively modulates the electronic structure which enhances the charge transfer required for water dehydrogenation and also narrows the band gap. In brief, as shown in Fig. 8(a) the energy profile of defective CeO2 for water dehydrogenation to O is very similar to that of perfect CeO2, but is easier. Moreover, the dissociation of OH is unfortunately very difficult in perfect CeO2 as the formed O and H tend to combine with each other producing OH again. But in the case of defective CeO2, OH dehydrogenation is much easier as the reverse reaction is very slow. Further, the presence of oxygen vacancies on CeO2(110) significantly reduces the activation energy of the rate limiting step (O–O bond formation) of water oxidation, due to the decreased O binding strength. Moreover, the distance between O and the second OH is shortened in the defective CeO2 compared to that of the perfect one, which indicates that lower activation energy is required for the formation of O2via the OOH pathway in the defective CeO2 as shown in Fig. 8(b). They found that normally in CeO2, the HOOH pathway is not favoured because of its high activation barrier, but the OOH pathway is favoured as described above. In addition to this, defective CeO2(110) possesses a higher rate constant for the rate limiting step which is about 2–3 orders of magnitude higher compared to that of the perfect CeO2(110). They also studied the photocatalytic oxygen generation under visible light of the above materials and found that the NaBH4 reduced CeO2, i.e. defective CeO2 NRs, exhibited the highest photocatalytic activity with the highest oxygen evolution rate (137.7 μmol g−1 h−1) than the pristine. For the first time, they reported how to control the defects by varying the NaBH4 amount. They found that on adding a particular amount of NaBH4, the vacancy concentration is optimized, and a further increase in the NaBH4 amount decreases the vacancy density, which strongly affects the photocatalytic hydrogen evolution activity.103
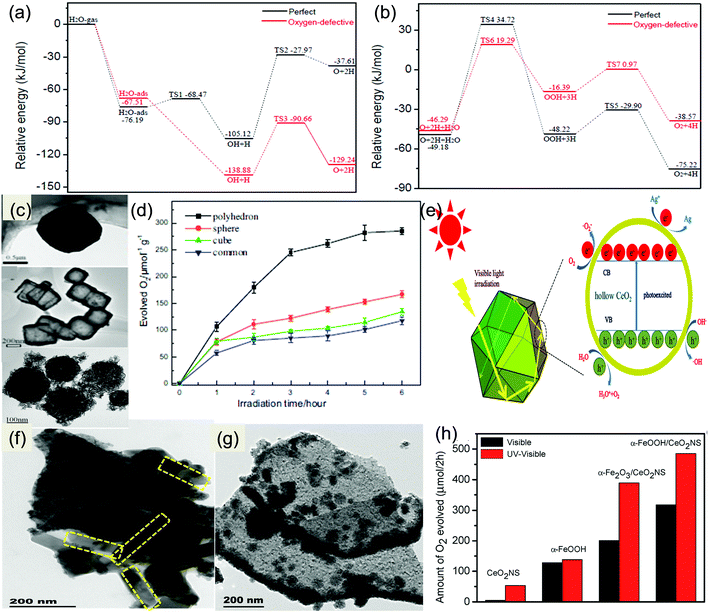 |
| Fig. 8 Potential energy profiles for (a) water dehydrogenation to O and (b) O–O bond formation. Reprinted from ref. 103 with permission from Elsevier. (c) TEM micrographs of polyhedron, cube and sphere shaped CeO2 (d) and their corresponding photocatalytic O2 evolution curves under visible light irradiation. (e) The schematic illustration of the photocatalytic O2 evolution mechanism over CeO2 hollow structures under visible light irradiation. Reprinted from ref. 90 with permission from Elsevier. (f and g) TEM micrographs of FeOOH/CeO2NS and Fe2O3/CeO2NS. (h) Oxygen evolution bar graph showing Fe modified CeO2.200 Reproduced by permission of The Royal Society of Chemistry. | |
Jiang et al. reported on the effect of crystal facets on the photocatalytic water oxidation reaction. They prepared CeO2 of two different shapes, i.e. cube (100) and rod (110 + 100), via a simple hydrothermal method, where for the rod morphology two different types of precursors (nitrate and chloride) were used. Under 5 sun illumination, CeO2 NRs prepared from the chloride precursor exhibited the highest oxygen evolution rate of 1.70 μmol h−1, which might be due to the presence of surface bound hydroxyl group at the defect sites and two active planes, i.e. 100 and 110, which retarded the photogenerated charge recombination ability.15 Further, Zhao et al. developed another nanostructured CeO2, i.e. CeO2 NRs, by hydrothermal synthesis followed by post-calcination under different atmosphere conditions. They found that Ar–H2-800 possesses more active oxygen (24.16%) which is directly related to Ce3+, and hence it emerged as a better water oxidation catalyst. They concluded that CeO2 NRs prepared under mixed H2 and Ar gas at 800 °C exhibit higher photocatalytic water oxidation ability at a yield rate of 707.73 μmol g−1, which was 10 times higher than that of CeO2 NRs calcined under air at 800 °C. The origin of the enhancement in the activity is mainly due to the increased content of surface oxygen vacancies and paramagnetic Ce3+ species under the reduced atmosphere which is helpful for reduction of the energy barrier for water adsorption and the corresponding photoxidation.69 Next, Qi et al. synthesized a multi-shell hollow microsphere through a general self-templating method and tested its activity towards photocatalytic water oxidation in the presence of AgNO3 solution (e− scavenger). They found that the triple shelled CeO2 hollow microsphere exhibited the highest O2 evolution activity of 78 mmol gcat−1 h−1 which was higher than that of the commercial CeO2 NPs. This outstanding performance of the catalyst was attributed to the hollow structure which absorbs more incident light because of the multiple reflections by the multi-shell and possesses a larger surface area with more active sites for water oxidation.91 Further Fang and co-workers synthesized different morphology oriented CeO2 such as polyhedral, cubic and sphere-shaped nanocages through the template (Cu2O) assisted method as shown in Fig. 8(c). A very interesting observation was made, i.e. polyhedral CeO2 (47.5 μmol g−1 h−1) nanocages possessed higher photocatalytic O2 evolution activity than cubic nanocages (22.4 μmol g−1 h−1) and sphere-shaped nanocages (27.8 μmol g−1 h−1) as shown in Fig. 8(d).90 As shown in Fig. 8(e), under light irradiation the CeO2 hollow structure absorbs photons and excites electron–hole pairs; then the photogenerated holes react with water to give molecular oxygen while the generated electrons are quenched by AgNO3. The activity improvement was ascribed to the polyhedral nanocage containing more active sites, a higher surface area and efficient photogenerated charge transport and separation. Additionally, the light absorption ability is enhanced in this particular nanostructure because of its inner shell which reflects the light properly. Primo et al. decorated gold particulate over quantum sized CeO2 for activity improvement and efficient light absorption. It was found that 1 wt% gold loaded CeO2 nanoparticles exhibited the highest oxygen evolution rate, i.e. 105 μmol h−1, which was much more than that of the standard WO3 photocatalyst (1.7 μmol h−1). This remarkable photocatalytic activity was mainly due to the small size (5 nm) CeO2, where they controlled the particle size of ceria (5 nm) by means of electrostatic binding of Ce 4p to alginate gel, subsequent supercritical CO2 drying, and calcination.198
Other than nanostructured CeO2, very limited work on modified CeO2 based materials has come into existance.199–201 For example, Fang et al. fabricated CoOx decorated CeO2 hollow nanocages for improved water oxidation. With an appropriate amount of CoOx loading, the CeO2 based hollow structure not only possessed a higher ability for light absorption but also showed a high O2 evolution rate, i.e. 200 μmol g−1 h−1, under visible-light irradiation with excellent durability.199 Further, Parida and co-workers attempted to exploit new visible light self-healing 1D–2D FeOOH nanorods/CeO2NS and 3D–2D Fe2O3/CeO2NS hybrids in 2-dimensional nanosheet architecture photocatalysts as shown in Fig. 8(f and g) by a facile hydrothermal method and evaluated their photocatalytic performance towards the oxygen evolution reaction under UV-visible light. The optimised FeOOH/CeO2NS and Fe2O3/CeO2NS systems exhibit unprecedented photocatalytic activity towards O2 evolution, i.e. 485 μmol/2 h and 389 μmol/2 h respectively (Fig. 8(h)). The enhanced performance of Fe/CeO2 is attributed to the 2D structure of CeO2 which provides the perfect site for growth of iron materials and extends the charge separation process throughout the nanohybrid. Additionally, the formation of a n–n junction across the heterointerface modifies the charge separation and transportation process. Further, the Ce3+/Ce4+ redox cycle acts as an activator which healed photocorrode Fe2+ and regenerating the structural damage caused by irradiation.200 Recently, Meng et al. synthesized a new type of molecular catalyst (MC) combined semiconductor heterostructure, i.e. a CoTCPP@CeO2 nanotube heterostructure. The optimized CoTCPP@CeO2 nanocomposite exhibited O2 evolution at a rate of 30 μmol g−1 h−1 with long term durability and was found to be superior to other MC combined CeO2, for example H2TCPP@CeO2 NTs, Co3O4@CeO2 NTs and TCPP@Co3O4@CeO2 NTs, under the same conditions. They proposed that initially, CoTCPP acts as a charge transporter to propel photoexcited electrons to CeO2 by d–f electron coupling, which effectively suppresses the photogenerated charge recombination. In addition to this, the interfacial charge transfer results in in situ generation of surface oxygen vacancies and CoOOH species, which are found to be the major sites for enhancing water oxidation.201
5. CO2 reduction reaction
The continuous rise in the atmospheric carbon dioxide level and depletion of fossil resources is a pressing concern that is directly threatening the balance of earth's ecosystems and sustainability of life for future generations. Among various on-going research activities for protection and remediation of the environment and solving the energy crisis, carbon dioxide capture and subsequent conversion into high liquid fuels including value-added chemicals is regarded as a potential strategy to slow down the alarming climate change and sustainably address the upcoming energy crises. Photocatalytic conversion of CO2 to renewable fuel using solar energy has attracted more attention in the past 3 to 4 decades. Since then, various nanostructured metal oxide photocatalysts have been examined for the reduction of CO2, and great achievements have been made. However, the rational design and synthesis of photocatalysts with high activity and selectivity towards the reduction of CO2 is highly challenging.202,203 CeO2 has been widely used as the catalyst for CO2 reduction, so both theoretical and experimental studies have eventually surfaced in this field, as summarized in Table 2.204–217
Table 2 Complete results of the CO2 photoreduction performance of CeO2 and CeO2 based heterostructures
Catalyst |
Light source |
Reaction conditions |
Activity |
Ref. |
CeO2(100)/CeO2(110) |
300 W Xe lamp |
Water, CO2 gas |
CH4 0.86 μmol h−1 g−1 1.12 μmol h−1 g−1 (Pt, MnOx co-catalyst) |
84
|
Pt/MnOx |
Defective CeO2 NRs |
500 W Xe lamp |
CO2 gas phase reduction |
CO 24–28 μmol g−1 in 6 h |
104
|
CeO2–SAC (defective) |
300 W Xe lamp |
NaHCO3 |
CH3OH – 0.702 μmol h−1 g−1 |
106
|
CeO2–CSC |
0.397 μmol h−1g−1 |
r-CeO2(110) |
300 W Xe lamp |
Water, CO2 gas |
CO 0.203 μmol h−1 g−1 |
206
|
c-CeO2(100) |
CO 0.130 μmol h−1 g−1 |
Ordered mesoporous CeO2–TiO2 |
300 W Xe arc lamp |
Water, CO2 gas |
CH4 – nearly 12 mmol g−1 in 325 min and CO – nearly 70 mmol g−1 in 325 min |
207
|
3D ordered macroporous TiO2–CeO2 |
300 W Xe lamp (λ > 420 nm) |
Water, CO2 gas |
CO – 2.06 μmol/400 min/100 mg |
208
|
ZnO–CeO2 |
300 W Xe lamp |
Water, CO2 gas |
CO – 0.52 μmol h−1 g−1 and CH4 – 0.06 μmol h−1 g−1 |
209
|
Vacancy Cu2O/CeO2 |
300 W Xe lamp |
Water, CO2 gas |
CO nearly 1.2 μmol g−1 in 8 h |
210
|
CeO2/Bi2MoO6 |
300 W Xe arc lamp (λ > 420 nm) |
Water, CO2 gas |
CH3OH – 32.5 μmol h−1 g−1 and C2H5OH – 25.9 μmol h−1 g−1 in 4 h |
211
|
CdS@CeO2 core/shell |
300 W Xe lamp (λ > 420 nm) |
Water, CO2 gas |
CH3OH – nearly 1100 μmol g−1 in 8 h |
212
|
CH4 – nearly 7 μmol g−1 in 8 h |
ZnIn2S4 flower/CeO2 |
300 W Xe lamp (λ > 420 nm) |
NaHCO3, H2SO4 |
CH3OH 0.542 μmol h−1 g−1 |
213
|
Defect rich g-C3N4@CeO2 |
300 W Xe lamp (λ > 420 nm) |
Water, CO2 gas |
CH4 – 3.5 μmol g−1, CH3OH – 5.2 μmol g−1 and CO – 16.8 μmol g−1 in 3 h |
214
|
m-CeO2/g-C3N4 |
300 W Xe arc lamp |
Water, CO2 gas |
CO – 0.590 μmol/50 mg/6 h and CH4 – 0.694 μmol/50 mg/6 h |
215
|
CeO2–3D-gC3N4 |
UV light |
NaOH, TEOA, CO2 gas |
CO 4.69 μmol h−1 g−1 and CH4 3.03 μmol h−1 g−1 |
216
|
Pt-cocatalyst |
CeO2–N-doped graphene–Cu2+ |
250 W Xe lamp |
NaHCO3, Na2SO3, CO2 gas |
CH3OH 507.3 μmol h−1 g−1 |
217
|
CeO2MOF |
300 W Xe lamp |
Water, CO2 gas |
CO 1.68 μmol h−1 g−1 |
218
|
The theoretical concept of CO2 adsorption, activation and subsequent reduction over the CeO2 surface is as follows: at first, the CO2 molecule is adsorbed in a horizontal configuration where the O atoms sit on top of the surface of Ce while the C atom sits on the four-fold hollow site of the top layer CeO2. Then the adsorbed CO2 is reduced to either a formate or carboxyl intermediate in the presence of adsorbed hydrogen, while in the absence of any adsorbed hydrogen, the CO2 directly forms carboxylate species. Further, the formed intermediate (formate/carboxyl) undergoes dissociation to form CO species. However in defective CeO2, CO2 is directly dissociated into CO by donating its one oxygen to the oxygen vacancy. On CO2 activation, the oxygen atom is directly incorporated into the vacant site via a redox mechanism and neutralizes the vacancy to generate the CO intermediate. Both the carboxyl and formate intermediates can produce CO while only the carboxyl intermediate will on further hydrogenation produce methanol on the ceria surface. The carboxylate pathway is more favourable for hydrogenation due to the presence of surface vacancies. Hence, the theoretical calculation gives full proof evidence for the effective CO2 reduction in both oxidized and reduced CeO2.204,205 In this regard, there are a number of experimental reports on CO2 photoreduction over CeO2 based materials with eye-catching results. For example, Dong and coworkers analyzed the CO2 reduction over (110) and (100) surfaces through both experimental and theoretical investigations and proposed a mechanism of CO2 photoreduction over defective CeO2 as shown in Fig. 9(a). They observed that the synergistic effect of both Lewis acid sites (oxygen vacancy) and Lewis base sites (hydroxyl group) was responsible for CO2 activation and its subsequent reduction. Combining the results of CO2-TPD, CO2-adsorption, DRIFTS and DFT studies (Fig. 9(b and c), the authors concluded that the CeO2(100) plane possesses more hydroxyl species than the CeO2(110) plane, which are beneficial for the adsorption and subsequent activation of CO2. Additionally, they illustrated the CO2 reduction mechanism as follows (shown in Fig. 9(a)): the O atom of the surface hydroxyl group of CeO2 donated electrons to the C atom of CO2 and eventually, the O atom of the CO2 reactant gave its p electrons to the oxygen vacancy of CeO2, resulting in the formation of HCO3− species. Next, the carboxylate (˙CO2−) radical ion intermediate was formed when the photoexcited electron transferred to the surface HCO3− species which was further confirmed by in situ ESR analysis. Finally, with the formation of OH, the CO gas was produced from the intermediate carboxylate species. Therefore, the synergistic interactions between the Lewis acidic and basic group are beneficial for CO2 photoreduction.95
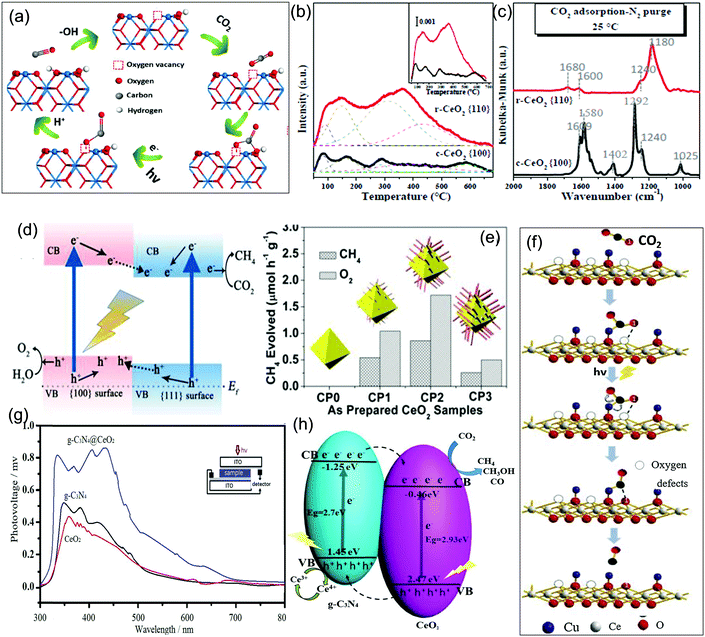 |
| Fig. 9 (a) The possible CO2 photoreduction mechanism on the oxygen-defective CeO2(110) surface. (b) and (c) CO2-TPD and CO2 adsorption DRIFTS of the CeO2(110) nanorod and the CeO2(100) nanocube. (d) Schematic illustration of the photogenerated charge transfer mechanism between two exposed planes within prism anchored octahedral CeO2. (e) CO2 photoreduction efficiency showing the best prism anchored octahedron. Reprinted with permission from ref. 84 and 95, copyright 2020, 2015 American Chemical Society. (f) Proposed mechanism of CO2 photoreduction on CuO decorated CeO2. Reprinted from ref. 210 with permission from Elsevier. (g) Photocatalytic CO2 reduction profile of CeO2, g-C3N4 and a series of g-C3N4@CeO2 heterostructures. (h) Plausible photogenerated charge pair separation and transfer and CO2 photoreduction mechanism over the g-C3N4@CeO2 heterostructure. Reprinted from ref. 214 with permission from Elsevier. | |
Additionally, Jiang et al. prepared defective CeO2 nanorods for the photocatalytic gas-phase CO2 reduction reaction and via a systematic TEM and HRTEM study they confirmed the lattice distortion and dislocation type surface defects. Further, they found that the prepared CeO2 can generate CO with nearly 100% selectivity with a yield of 24–28 μmol gcat−1 after 6 hours of irradiation, which was like a milestone for neat CeO2. However, after the prolonged irradiation (more than 20 h), the photoreduction activity was slowed down, as initially the local strain and oxygen vacancy help to activate adsorbed CO2, but after a certain time, these vacancies were gradually consumed leaving behind massive electrophilic oxygen species over the CeO2 surface which restricts the further photoreduction, thereby slowing down the formation yield of CO. They further found that the activity was recovered when the exhausted CeO2 was again treated with NaBH4 solution resulting in the subsequent defect formation.104 Further, Li et al. reported the first kind of nanostructured CeO2, i.e. hexahedron prism anchored octahedron, towards photocatalytic CO2 reduction. According to the DOS study, there is a slight energy difference between the CB and VB of the (100) plane of the hexahedron prism and the (111) plane exposed octahedron which forms a homojunction between them. This homojunction is mainly responsible for the effective charge pair separation and transfer, where the electrons flow from the higher Fermi level of the hexahedron prism with exposed (100) facets to the (111) octahedron surface and catalyze the adsorbed CO2 as shown in Fig. 9(d), while holes at the 100 surface react with water to generate oxygen. Hence, the hexahedron with a medium denser arm generated the most CH4 by the reduction of CO2 at the rate of 0.86 μmol h−1 g−1 while in presence of MnOx co-catalyst the production rate was further increased to 1.12 μmol h−1 g−1 as depicted in Fig. 9(e).84 Recently Hezam et al. reported a novel preparation method, i.e. solar light assisted combustion synthesis, for the production of 12–18 nm diameter CeO2 particles containing a high amount of surface defects. Under simulated solar light, the as-prepared Ov CeO2 exhibited the highest photocatalytic reduction of CO2 to CH3OH at the rate of 0.702 μmol h−1 g−1 with a quantum efficiency of 0.23% at 350 nm, while the conventional solution combustion assisted CeO2 displayed only a rate of 0.397 μmol h−1 g−1. From DFT simulation it was found that the generated oxygen vacancy increases its light absorption by narrowing the band gap of CeO2, and enhances its CO2 capture ability. Moreover, the mean crystallite size, low crystallinity and mesoporous structure played a crucial role in the selectivity of CO2 reduction to methanol.106 Further, Ullah et al. carried out photothermal CO2 reduction over transition metal (Cu, Co) loaded CeO2 and found that transition metal loaded CeO2 exhibits the highest activity as well as selectivity towards methane production compared to pristine CeO2. The improved photoactivity was attributed to the generation of hot electrons from transition metals which directly activate the CO2 molecule to undergo smooth degradation and further create in situ vacancies in the CeO2 support which act as localized charge density sites.206
In addition to these, a number of articles have recently been reported for various heterostructures/composites of CeO2 with different metal oxides,207,211 metal sulphides212,213 carbonaceous materials,214–218etc. For example, Wang et al. prepared an ordered mesoporous (OM) CeO2–TiO2 composite of 2D hexagonal structure with a high specific surface area and hierarchical porosity by using SBA-15 as the template. The introduction of CeO2 species increased the surface chemisorbed oxygen of the ordered mesoporous TiO2 and effectively extended the spectral response of the nanohybrid from UV to visible. The composite was effectively utilized for CO2 photoreduction under simulated solar light with excellent selectivity and catalytic efficiency. The work demonstrated that the 3DOM CeO2/TiO2 catalyst showed the highest CO production, i.e. nearly 70 mmol gcat−1, and nearly 11.5 mmol gcat−1 CH4 during 325 min light irradiation. The higher activity was attributed to the 2D-open pore structure, which allows fast interparticle reactant diffusion, and the presence of CeO2 which helped in the effective electric field formation through which photogenerated electrons effectively drifted from the TiO2 surface to CeO2 where they took part in the photoreduction reaction.207 In another work, Dai et al. synthesized a series of CeO2/Bi2MoO6 microsphere nanocomposites (0D–2D) towards CO2 photoreduction and studied the inside mechanism. They found that the CeO2 surface strengthens the bonding of CO2 and enhances the formation of intermediates like CO32− and HCO3−. Moreover, the heterojunction accelerates electron–hole transfer and separation which ultimately uplifts the reduction activity. For this system, the total yield of CH3OH and C2H5OH was 58.4 μmol gcat−1 over 5C-BM, which was about 4.1 and 1.9 times higher than that of the neat CeO2 (14.1 μmol gcat−1) and Bi2MoO6 (30.9 μmol gcat−1), respectively.211 Again, Dong and co-workers developed a very interesting p–n heterojunction composite of Cu2O/CeO2 for photon driven CO2 reduction. They found that the deposition of Cu2O over the CeO2 surface created more surface defects and also accelerated the charge transfer process at the Cu2O and CeO2 interface. Furthermore, Cu2O plays an important role by creating Lewis acid–base sites, while the oxygen vacancy over CeO2 increases the pace of CO2 reduction to CO. In brief they systematically studied the reaction pathway and the mechanism as presented in Fig. 9(f), and concluded that first Cu atoms of Cu2O perfectly bound the carbon atoms of CO2 molecules via d-orbital electrons leading to the formation of the carboxylate ion. Further, under light irradiation photo-generated electrons transferred from Cu to the C–O bond of carboxylate, and the O atom of carboxylate interacted with the oxygen vacancy (Ov) on the CeO2 surface, and then the O atom transferred to Ov. Finally, the above interaction promoted the breaking of the C–O bond of the adsorbed carboxylate species, and thus the CO molecule was generated.210
In addition to oxide heterostructures, metal sulphides have also been used as a component for CeO2 in the CO2 reduction reaction. For example, a core–shell structure of CdS@CeO2 towards visible light assisted CO2 reduction was reported by Ijaz et al. In the experiment performed, two types of reduction products are observed, i.e. methanol as the major product because of the thermodynamic and kinetic concern and methane as the minor product. The type II heterojunction at the core/shell interface enhances the photogenerated charge pair separation and hence improves the stability of the CdS core as the CeO2 outer layer inhibits the photocorrosion of CdS. In brief, under the visible light irradiation condition photogenerated electrons and holes were produced on the surface of CdS, and then the electrons transferred from CdS to the conduction band of CeO2. Further, on the surface of CeO2 these electrons reduced the adsorbed CO2 into the negative and metastable superoxide (˙CO2−) radicals, which then eventually led to the formation of CH4 at the rate of 1100 μmol g−1 for 8 h.212
Liang's group demonstrated an interesting synthetic strategy for the formation of a hollow heterostructure of g-C3N4@CeO2 with enough oxygen vacancies by using SiO2 as a template. The catalytic ability of the vacancy enriched heterostructure was tested towards CO2 photoreduction and the obtained products were CO (16.8 μmol g−1), CH3OH (5.2 μmol g−1) and CH4 (3.5 μmol g−1), demonstrating the higher ability of this heterostructure compared to most of the other reported g-C3N4 based photocatalysts. Further, the apparent quantum efficiency for CO2 reduction to methane was found to be 17.1% at 525 nm. They concluded that the reason behind the increment in the performance is mainly due to its unique structure and a delayed charge pair recombination rate as confirmed from SPS analysis (shown in Fig. 9(g)). Although both g-C3N4 and CeO2 showed SPS response the 3D heterostructure exhibited the most intensive response under visible light which confirmed the effective charge carrier separation.214 As shown in Fig. 9(h), the separation of excitons occurs at the g-C3N4 and CeO2 interface, while the photogenerated electrons accumulate over the CeO2 surface where the electrons combine with Ce4+ to generate Ce3+. Further, Ce3+ reacts with the gas phase CO2 to form ˙CO2− which is further reduced to yield CO, and hence the yield of CO is very high in this case. Further in the presence of adsorbed H and ˙CO2− hydrogenation occurs resulting in other products like methanol and methane.
6. Nitrogen reduction reaction (NRR)
NH3 is considered as an important chemical for both industries (explosive, resin, plastics and fibres) and agriculture (fertilizer). Recently, the global need for ammonia has seen a sharp increment due to its additional application, i.e. as a carbon-neutral fuel and specifically as a potential energy carrier due to its high hydrogen density (17.6 wt%), easy handling/storage and low liquefiable condition (8 bar). Currently, about 150 million tonnes of ammonia is produced per year via the traditional energy-intensive Haber–Bosch process (HB) to satisfy the population's food requirement and various other applications. This HB process generally operates under extremely harsh conditions, i.e. 400–450 °C and 100–200 atm, and also utilizes 1–3% of global electrical energy (i.e. 3.5 × 104 to 5 × 104 J gNH3−1). Moreover, the supplied H2 comes from natural gas (3–5%), and unfortunately releases a substantial amount of CO2 (1.87 tons per ton of NH3) which creates an environmental catastrophe.219–225 To replace the HB process, a renewable, scalable, and eco-friendly strategy towards ammonia production is highly essential. Among different ongoing strategies, photocatalytic reduction of N2 to NH3 is a very hot topic of research towards the development of renewable technologies. Photocatalytic nitrogen reduction generally operates under mild reaction conditions and utilizes renewable feedstock, i.e. solar light and water. So far many photocatalytic systems have been developed and tested for effective nitrogen fixation. It is found that the materials with intrinsic surface defects are the most suitable candidates for this process.220 In this perspective, CeO2 with surface oxygen defects and Ce3+ has emerged as one of the active catalytic systems for the NRR.226–232
In this context, Qi et al. using first principles calculations investigated the NRR process over CeO2 and found that defective CeO2 with Ce3+ sites (Lewis acid) facilitates N2 adsorption (Lewis base) via an acid–base adduct mechanism as shown in Fig. 10(a). The exposed CeO2 sites on CeO2((111), (110), (100)) can cause N2 to get adsorbed in a lying down manner, which encourages N2 activation and thus leads to an efficient reduction. The surface oxygen vacancy weakens the adsorbed N
N bond strength and lowers the adsorption energy of the N2H* intermediate as shown in Fig. 10(b) and thereby facilitates further reduction. In brief, in the pristine CeO2 with (111), (110) and (100) exposed facets, N2 adsorbs on the surface of Ce sites in a standing up manner indicating weak physical adsorption. Hence the N2 molecule can’t be activated on the surface of perfect/pristine CeO2 and further, the energy barrier for the first protonation of N2* seems to be very high leading to inefficiency of the NRR. However, defective CeO2(111), (110) and (100) facets containing multiple oxygen vacancies and unsaturated Ce3+ Lewis acid cluster sites (tri/bi and tetra-Ce sites) could cause N2 to be adsorbed in a lying down manner, which further promotes N2 activation. Moreover, the unsaturated tri and bi-Ce sites could stabilize the intermediate N2H*, while lowering ΔG for the first protonation, and then the alternate mechanistic pathway of ammonia formation proceeds. Further, it was found that the NRR activity over the different planes of CeO2 is determined by their unsaturated Ce sites which are in the following order: (100) > (110) > (111), as the (100) plane contains tetra and tri-Ce sites.226
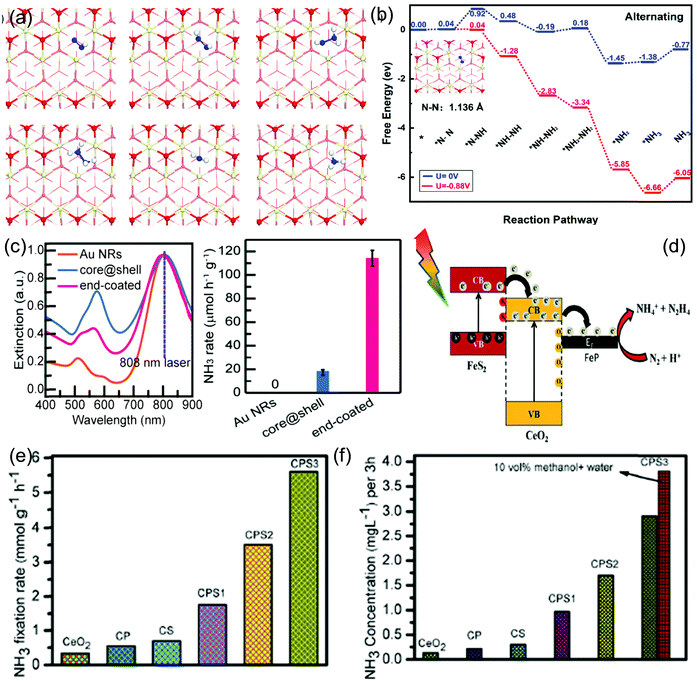 |
| Fig. 10 (a) Optimized geometries of the NRR pathway on defective CeO2(111). (b) Corresponding Gibbs free energy profiles. Reprinted from ref. 226 with permission from Elsevier. (c) Extinction spectra of three photocatalyst nanostructures and N2 photofixation rates of Au nanorods, Au@CeO2 and Au-end coated CeO2 photocatalysts under NIR light illumination. Reprinted with permission from ref. 113, copyright 2019 American Chemical Society. (d) Proposed mechanism of nitrogen reduction over FeS2/P-CeO2 based defective materials. (e) and (f) NH3 concentration from PNRR over a CeO2 based catalyst in acidic medium and distilled water.231 Reproduced by permission of The Royal Society of Chemistry. | |
Different CeO2 based electrocatalysts for NRR activity have also been reported so far227–229 with a high production rate and selectivity. For example, Xu et al. prepared oxygen vacancy enriched CeO2 nanorods and achieved a great enhancement for the electrocatalytic NRR. However, no significant works regarding CeO2 and CeO2 based materials have been explored in the field of photo-fixation of N2 (PNRR). Among the countable research works, Feng et al. prepared a series of ternary photocatalysts CeCO3OH/g-C3N4/CeO2 by using CeCl3 and graphitic carbon nitride (g-C3N4) as reaction precursors via facile in situ self-sacrificing hydrothermal methods. Without using any sacrificial agent, the photocatalyst prepared at 180 °C exhibited the highest nitrogen photoreduction activity, i.e. 1.16 mM g−1 h−1, which was four times that of pristine g-C3N4. The enhanced nitrogen photofixation performance was attributed to the surface defects in CeO2 where Ce3+ acts as a chemisorption site for N2 molecule adsorption and subsequent activation and the heterostructure interface provides a higher charge transfer and separation efficiency.230 In another case, Jia et al. proposed an innovative synthesis idea of the Au–CeO2 nanostructure, unlike the reported core–shell structure, via a facile wet-chemistry route, where crystalline CeO2 was selectively grown over the ends of gold nanorods (Au NRs) in the presence of a small amount of bi-functional K2PtCl4. A large number of oxygen vacancies were generated in the surface grown ceria which further enhanced the adsorption and the subsequent reduction of di-nitrogen molecules. They tuned their longitudinal plasmon wavelengths finely so that the materials can be adjusted as closely as possible to the laser wavelength as shown in Fig. 10(c). Further, the generation of plasmon-induced hot electrons and the presence of oxygen vacancies enabled the unique structural Au/end-CeO2 to function as a photocatalyst for nitrogen photofixation. The obtained nanostructured catalyst under 808 nm laser illumination led to a high rate of production of NH3, i.e. 114.3 μmol h−1 g−1, which was 6.2-fold more in comparison to the other synthesized core@shell (Au@CeO2) nanostructure (Fig. 10(c)). As a proof-of-concept, the photocatalytic N2 fixation ability of the Au/end-CeO2 nanostructures was also evaluated under 1 sun illumination and the NH3 generation rate was found to be 25.6 μmol h−1 g−1.113 The author's group have also studied different CeO2 based materials for photocatalytic nitrogen fixation.231,232 For example, 3D/2D-FeS2–FeP–CeO2 nanohybrids with high surface defects were successfully synthesized through an in situ hydrothermal technique followed by a combined sulphidation and phosphidation technique. The as-prepared samples were tested towards PNRR under UV-vis light without the use of any organic scavenger or noble metal co-catalyst. CeO2 acts as a building block photocatalyst and supports the FeP/FeS2 particles, and under light irradiation FeS2 acts as an electron donor and injects photoelectrons on to the CeO2. Further the presence of oxygen vacancies along with FeP encouraged the productive N2 activation and photoreduction to ammonia. The key to the remarkable photocatalytic performance was the presence of Fe based materials where FeP serves as the catalytic site for N2 adsorption and facilitates the photogenerated charge transfer mechanism from CeO2 to nitrogen as shown in Fig. 10(d). The optimized FeP–FeS2–CeO2 displayed a very high ammonia production, i.e. 2.91 mg L−1, without a sacrificial agent whereas in the presence of aqueous methanol solution, the ammonia production rate was increased significantly. This suggests that methanol both acts as a sacrificial electron donor and produces CO2*− which facilitates the N2 fixation ability. Further, the nitrogen fixation rate was 5.66 mmol h−1 g−1 with selectivity in acidic distilled water under mild reaction conditions (Fig. 10(e and f)).231
7. Photocatalytic pollution abatement
The 21st century is the era of rapid development covering all sectors of the world but the serious side effect of this progress may result in an environmental catastrophe. The continuous rise of industrial activities and different technological advancements have damaged the growth of society by releasing their additional waste to water bodies, soil and air without proper treatment causing a serious threat to biodiversity. Mainly the wastewater effluents contain maximum contaminants in the form of various organic pollutants such as dyes, phenolic compounds, antibiotics, halogenated hydrocarbons and inorganic toxic pollutants such as highly dangerous heavy metals and radioactive nuclides.233–239 These pollutants are highly toxic and take a very long time to degrade naturally. So the effective removal and degradation of these pollutants have taken many years of research. A variety of technologies such as chemical oxidation, adsorption, electrochemical conversion, and biodegradation have been used so far but most of them are highly energy demanding and cannot be used on a regular basis.240–245 However, the photocatalytic detoxification process has emerged as a more efficient, cost-effective and green strategy which has the ability to clean up all the detrimental pollutants from wastewater as well as air bodies. In this technique, highly energetic radicals such as hydroxyl, superoxide, and hydrogen peroxide along with photogenerated electrons and holes are produced which effectively convert the organic/inorganic pollutant to a non-toxic form very quickly.246–248 With regard to this, many researchers have developed different photocatalytic materials for their effective utilization towards pollutant removal from different sources.
In the context of the environment cleanup process, various nanostructured CeO2 and CeO2 based materials have been reported for air purification and wastewater treatment by efficient photocatalytic degradation/mineralization of different dyes (RhB, MB, acid orange, eosin yellow, etc.),249–274 antibiotics275–284 and organic pollutants (phenol-based materials),285–289 as summarized in Table 3. Morphology alteration enhances the surface defects such as Ce3+ state and oxygen vacancies, which increases the light absorption parameter with a red-shifted absorption edge and also retards the photogenerated electron–hole recombination rate. Further, these sites act as strong binding and dissociation sites for adsorbate molecules, and hence act as good sites for the photocatalytic degradation process. And it was also found that these CeO2 materials generate more radical species (hydroxyl and superoxide) because of the prolonged lifetime of excitons and the presence of surface defects. Recently, Amoresi et al. studied the photocatalytic decolourization efficiency of RhB over different morphology oriented CeO2. According to theoretical models and experimental studies, it was found that the photodegradation rate varies with the morphology and exposed planes. Since the electron density is different for different planes and is of the order (110) > (100) > (111) > (311), different morphologies showed different photocatalytic activity. For the bean (100) shaped morphology, h+ are the dominant species and for rods (110) it is e−, while e−/h+ are equally responsible for the photocatalytic activity of hexagon (311). For the (111) dominant morphology, OH* act as the major species for degradation purposes, since they have strong e−/h+ interaction. Hence different morphologies exhibited different photoactivity for a selected pollutant molecule.62
Table 3 Complete results of the pollutant degradation performance of CeO2 and CeO2 based heterostructures
Catalyst |
Light source |
Reaction conditions |
Activity |
Ref. |
CeO2 hierarchical NRs |
White light, 500 W Xe lamp |
20 mg L−1 MO, 20 mg |
98.2% in 180 min |
77
|
CeO2 hierarchical NWs |
99.3% in 180 min |
Single crystalline mesoporous CeO2 nanosheet |
250 W high pressure mercury lamp |
1.0 × 10−5 mol L−1 RhB, 20 mg |
Nearly 80% in 120 min |
79
|
Ordered meso-CeO2 |
1000 W Xe lamp (λ > 420 nm) |
70 mg L−1 AO 7, 50 mg |
95% in 7 h |
89
|
Flower-like CeO2 |
UV light (λ = 365 nm) |
0.3 mM AO 7, 1 cm2 |
∼65% in 10 h |
249
|
CeO2 microspheres |
1000 W Xe lamp (λ > 420 nm) |
50 mg L−1 AO 7, 50 mg |
69.7% in 180 min |
268
|
CeO2 NSs |
450 W Xe lamp (λ > 420 nm) |
26.7 μM MB, 30 mg |
90% in 6 h |
269
|
CeO2 NCs |
310 nm < λ < 400 nm |
RhB, 50 mg |
30% in 2 h |
270
|
CeO2 lamellar |
UV light, 120 W uviol lamp |
5 mg L−1 MB, 50 mg |
96.5% in 60 min |
271
|
Octahedral CeO2 |
UV |
5, 20 mg L−1 MO, 20 mg |
98.1% in 5 h |
272
|
CeO2 NTs |
92.8% in 5 h |
Au/CeO2 |
400 W Xe lamp (λ > 400 nm) |
10 mg L−1 MO, MB, 2 mg |
80% in 6 h, 95% in 5 h |
273
|
BiVO4/CeO2 |
Halogen lamp, visible (λ > 400 nm) |
2 × 10−5 M MB, 50 mg |
∼80% in 30 min |
251
|
CeO2/Fe2O3 nanospindles |
White, 150 Xe lamp |
0.1 mM EY, 1 mg |
98% in 25 min |
252
|
CuO/CeO2 |
15 W UV tube |
0.15 mM MB, 100 mg |
91.3% in 2 h |
253
|
CeO2/TiO2 nanobelt |
350 W mercury lamp (max. emission 356 nm) |
20 mg L−1 MO, 20 mg |
100% in 25 min |
254
|
300 W Xe arc lamp (λ > 410 nm) |
87% in 25 min |
CeO2–TiO2 |
300 W Xe arc lamp (λ > 400 nm) |
Bph-dye |
∼72% in 3 h |
255
|
CeO2/ZnO nanodisks |
Natural solar light |
50 mg L−1 direct blue 15, 50 mg |
93% in 5 h |
256
|
Hierarchical CeO2/ZnO |
300 W Xe lamp |
10 mg L−1 RhB, 50 Mg |
96% in 80 min |
257
|
CdS/CeO2 |
154 W halogen lamps |
3 mg L−1 MB, 20 mg |
70% in 120 min |
258
|
CdS NRs–CeO2 NPs |
300 W Xe lamp |
40 mg L−1 dye, 40 mg |
96.68% in 48 min |
259
|
CdS NWs/CeO2 nanoparticles |
300 W Xe arc lamp (λ > 400 nm) |
10 mg L−1 nitroaromatics, 6 mg |
4-nitrophenol ∼90% in 3 min |
260
|
Flower like CeO2/MoS2 |
150 W Xe lamp-visible light |
25 mg L−1 MO, 25 mg |
96.1% in 90 min |
261
|
CeO2/CuS composite nanofibers |
Strip blue LED lamp |
3 mg L−1 MB |
96.38% in 40 min |
262
|
CeO2/C3N4 |
300 W Xe lamp (λ > 400 nm) |
10 mg L−1 MB, 100 mg |
95% in 2 h |
274
|
CeO2/g-C3N4 |
300 W Xe lamp (λ > 400 nm) |
10 mg L−1 MB, 25 mg |
∼98.59% in 3.5 h |
263
|
CeO2/g-C3N4 |
36 W UV lamp (OS72) with a wavelength of 254 nm |
10 mg L−1 MB, 50 mg |
90.1% in 180 min |
264
|
CeO2/g-C3N4 |
8 W Xe lamp |
10−5 M concentration, 10 mg |
97% (MB dye) and 96% (Rh-B dye) in 60 min |
265
|
rGO/CeO2 NCs |
Hg lamp UV |
1 × 10−5 M MB, 30 mg |
∼87% in 90 min |
266
|
rGO/CeO2 |
Visible light source with intensity 14.5 W m−2 |
1 mM MO |
88.3% in 60 min |
267
|
CeO2 |
300 W Xe lamp |
10 mg L−1 CIP, 50 mg |
More than 90% in 15 min |
275
|
Hollow BiOCl@CeO2 |
300 W Xe lamp (λ > 400 nm) |
10 mg L−1 TC, 50 mg |
92% for TC in 120 min |
276
|
CeO2/Ti3C2–MXene |
350 W Xe lamp |
10 mg L−1 TC, 30 mg |
80.2% of TC in 60 min |
277
|
Nebula-like ZnO/CeO2@HNTs |
300 W Xe lamp (780–1100 nm) |
20 mg L−1 TC, 30 mg |
87% in 60 min |
278
|
Ag2O/CeO2 |
300 W Xe lamp (λ > 420 nm) |
10 mg L−1 enrofloxacin, 50 mg |
87.1% in 120 min |
279
|
CeO2/Co3O4 porous nanosheet |
Xe lamp (350 nm < λ < 780 nm) |
10 mg L−1 MB |
96.42% in 70 min |
280
|
5 mg |
85.35% in 30 min |
20 mg L−1 TC |
|
Bi2O3@CeO2 |
500 W Xe lamp (λ > 420 nm) |
10 mg L−1 TC, 20 mg |
100% in 180 min |
281
|
Shuttle-like CeO2/g-C3N4 |
150 W high pressure |
10 mg L−1 NOR, 50 mg |
88.6% in 60 min |
282
|
Xe lamp (λ > 420 nm) |
CeO2/nitrogen-doped carbon quantum dot/gC3N4 |
300 W Xe lamp (λ > 420 nm) |
20 mg L−1 TC, 100 mg |
100% in 60 min |
283
|
2D-CeO2 on 2D-MoS2 |
250 W Xe lamp (λ > 420 nm) |
10 mg L−1 CIP, 50 mg |
88.5% in 120 min |
284
|
Defective CeO2 |
Direct sunlight visible region (420–800 nm) |
50 ppm phenol 200 mg |
35% in 180 min |
105
|
50 ppm cholorphenol |
98% in 180 min |
50 ppm bromophenol |
96% in 180 min |
50 ppm nitrophenol |
99% in 180 min |
CeO2/g-C3N4 nanosheet |
300 W Xe lamp (λ > 420 nm) |
Phenol, bisphenol-A |
93.7% in 80 min (BPA) |
285
|
97.5% (phenol) |
CeO2/ZnCo–layered double hydroxide |
— |
20 mg L−1 phenol |
99.8% in 30 min |
286
|
CoS/CeO2 |
300 W Xe lamp (λ > 420 nm) |
25 mg L−1 phenol, 50 mg |
85.5% in 60 min |
287
|
20 mg L−1 TC |
96.5% in 60 min |
CeO2-rod/g-C3N4 |
300 W Xe lamp (λ > 300 nm) |
0.10 g benzylamine, 50 mg |
81.2% in 5 h |
288
|
Dye degradation
Zhang et al. reported CeO2 hierarchical nanowires (Fig. 11(a)) and nanorods (Fig. 11(b)) grown on Cu substrates for the photocatalytic degradation of methyl orange (MO) and found that as compared to CeO2 NPs, the 1-D nanostructure exhibited higher degradation efficiency. Both CeO2 HNRs and HNWs removed 98.2% and 99.3% of MO under light irradiation for 180 min, revealing a performance which is substantially higher than that of the commercial CeO2 NPs (68.8%) and P25 (89.5%) as shown in Fig. 11(c) respectively. The high performance in comparison to CeO2 NPs was achieved only due to their hierarchical 1D nanostructure derived active sites, resulting in a shorter diffusion length of photogenerated holes and increased surface areas. Further, of the two 1-D structures, although both the materials possessed a 3.2 eV band gap, the nanowires exhibited higher absorption in the wavelength range between 400 and 750 nm (Fig. 11(d)), suggesting higher photonic energy absorption.77 Further, Yu et al. reported uniform single crystalline hexagonal CeO2 nanosheets for RhB degradation and found that the degradation efficiency of the above nanosheets was more than that of the conventional CeO2 nanoparticles. The activity improvement was attributed to their 2D nanosheet structure which suppressed the charge pair recombination and their intrinsic single-crystal structure which decreased the charge transfer resistance, thereby yielding much stronger reducible electrons.79 Further, Arul et al. synthesized 3D hierarchical rose-flower-like CeO2 where CeO2 nanosheets are stalked to form a flower. Under UV light irradiation, the flower like catalyst showed a degradation rate of 65% for the AO7 dye. The 2D NSs provided better diffusion of generated holes and reduced the agglomeration of the 2D NSs which was responsible for the enhanced activity.249 In another experiment, Yuan et al. reported hierarchical CeO2 sheets for the photocatalytic decomposition of toxic acetaldehyde. They found that CeO2 HSs decomposed acetaldehyde more efficiently than CeO2 NPs and as well as the well known P25. This superior degradation activity is mainly attributed to the high surface to volume ratio of the catalyst and the presence of surface defects.250 Though nanostructured CeO2 possesses an impressive degradation property it is active only under UV-light illumination. Hence, the fabrication of a CeO2 based heterostructure is investigated, which visibly improves the interfacial charge transfer, light absorption and the charge separation and lowers the recombination resulting in enhanced photocatalytic degradation. So far, nanostructured CeO2 has been combined with different metal oxides,251–257 sulphides,258–262 carbonaceous materials,263–267etc. for efficient dye degradation. For example, Arul et al. reported CeO2/Fe2O3 spindles as recyclable photocatalysts for the degradation of Eosin Yellow (EY) under visible light irradiation. The degradation was achieved in a very short duration of time (98% in 25 min) in comparison to neat CeO2 and Fe2O3 NRs, because of the excellent charge separation and transfer property derived from the heterostructure (Fig. 11(e)). Fig. 11(f) shows the recycling study of the CeO2/Fe2O3 heterostructure, where the photocatalyst was found to be very stable and the photocatalytic efficiency was reduced by less than 10% even after 4 cyclic runs. Further they calculated the electrical energy per order of the photocatalyst, a term used to calculate the electrical energy required to remove unit mass of the pollutant. From the calculations, they found that the photocatalyst required much less energy to degrade the EY dye by one order of magnitude. Hence they deduced that the heterostructure possesses greater potential for use as a visible light driven catalyst. They deduced the photodegradation mechanism as shown in Fig. 11(g): upon light irradiation photogenerated electrons migrate from Fe2O3 to the Ce 4f band where they react with oxygen to generate superoxide radicals, while holes migrate from the VB of CeO2 towards the VB of Fe2O3 yielding hydroxyl radicals. Both the superoxide and hydroxyl radicals were found to be essential for the photodegradation process.252 Another low cost recyclable CeO2–metal oxide heterostructure was reported by Tian et al., where CeO2 nanoparticles assembled on the rough surface of TiO2 nanobelts producing the CeO2–TiO2 heterostructure. The heterostructure was tested for MO degradation under both UV and visible light, and they found that MO molecules were preferentially captured by CeO2 nanoparticles and were degraded by the free radicals and further released to the solution, and hence a capture, photodegradation and release process was followed. The heterostructure possessed enhanced photoactivity due to the efficient charge separation and transfer due to the well matching energy band between CeO2 and TiO2 and the large active surface area.254 Further Parida and co-workers prepared a series of 2D/2D-BiOI/CeO2 nanocomposites and tested their effectivity towards RhB decolourization efficiency. Among the very best catalysts, 40%BiOI–CeO2 was proven to be the best and within a very short time 89% decolourization efficiency was achieved for 100 ppm RhB under sunlight. The major cause behind the enhanced photoactivity was found to be the presence of excess surface oxygen vacancies and better charge separation efficiency through the Z-scheme at the interface.117 Further, Gu et al. reported a heterostructure photocatalyst consisting of one-dimensional (1D) CdS nanorods (NRs) and CeO2 nanoparticles (NPs). They found that the photocatalytic degradation of RhB was around 97% for the CdS/CeO2 heterostructure, which was much higher than that of its neat counterparts. The rate constant of CdS/CeO2 was nearly 28 times and 3.4 times higher than that of neat CeO2 and CdS respectively, due to the inhibition of electron–hole pair recombination benefitting from efficient electron transfer from CdS NRs to CeO2 NPs producing effective superoxide radicals. They studied the degradation pathway of RhB and found that after 16 min N-de-ethylated intermediates were formed but further with time, these products’ intensity decreased down. Further, a high mineralization rate (86% TOC removal) was observed, which confirmed the complete mineralization of RhB.259 Additionally, She et al. reported an important synthetic strategy to design a beautiful heterostructure between the CeO2(111) cube and the g-C3N4 nanosheet as shown in Fig. 11(h and i). The heterostructure exhibited 98.59% degradation efficiency of MB within 3.5 h under visible light which was much higher than that of the neat materials. The variation in the optical absorption spectra of MB degradation over 5%CeO2–gC3N4 is shown in Fig. 11(j). With light irradiation, as the time passed the colour of MB gradually disappeared which confirmed the photodegradation process.263 Verma et al. reported an in situ CeO2/rGO nanocomposite prepared through the one step ammonia assisted hydrothermal method for the elimination of MO under visible light. The heterostructure between CeO2 (0D) and graphene (2D) increased charge separation and transportation ability simultaneously and extended the range of the solar absorption spectrum as well as increased the dye molecule absorptivity over its surface. In brief, under light irradiation photogenerated electrons and holes are produced over the CeO2 surface; since rGO acts as a sink, electrons drift to the rGO surface and produce superoxide radicals, while the holes at the CeO2 surface react with water to generate hydroxyl radicals. Through the π–π interaction between rGO and the MO dye molecule, the dyes are efficiently adsorbed over the surface of rGO where they react with both superoxide and hydroxyl radicals to yield the degraded product.267
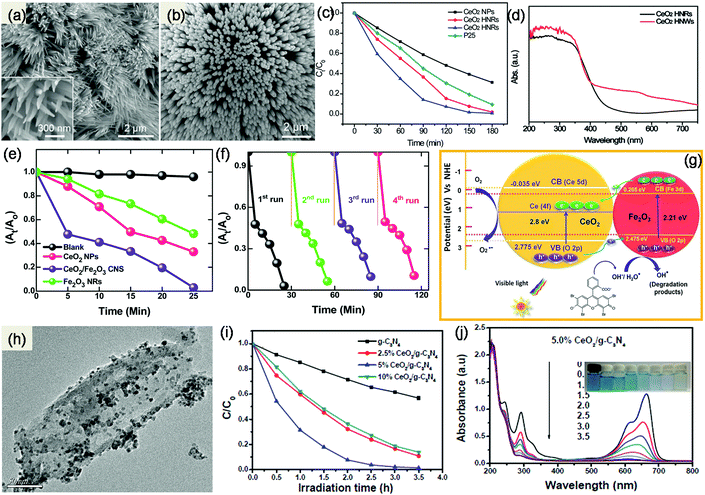 |
| Fig. 11 (a and b) SEM micrographs of CeO2 nanowires and nanorods respectively. (c) Photocatalytic degradation of MO over CeO2 HNRs and CeO2 HNWs in addition to that over CeO2 NPs and P25 with respect to light irradiation time. (d) UV-DRS spectra of CeO2 nanowires and nanorods.77 Reproduced by permission of The Royal Society of Chemistry. (e) Degradation efficiency of the blank EY with CeO2 nanoparticles, CeO2/Fe2O3 and Fe2O3 nanorod catalysts versus irradiation time. (f) Reusability test of the CeO2–Fe2O3 photocatalyst in degrading EY under visible light irradiation. (g) Proposed photodegradation mechanism of CeO2/Fe2O3 CNSs in degrading the EY dye under visible light irradiation.252 Reproduced by permission of The Royal Society of Chemistry. (h) TEM micrographs of CeO2–g-C3N4. (i) Photodegradation efficiency of the MB dye by g-C3N4 and CeO2/g-C3N4 catalysts versus light irradiation. (j) Changes in the ultraviolet-visible absorbance spectra of MB dye solutions with optimized CeO2–g-C3N4.263 Reproduced by permission of The Royal Society of Chemistry. | |
Antibiotics removal
Nowadays, the excessive use of antibiotics like tetracycline, norfloxacin, enrofloxacin, ciprofloxacin (CIP), etc. is a major headache for society, as these are non-biodegradable and cause adverse effects on the ecosystem. Amoresi et al. verified the morphological effect of different exposed plane CeO2 on CIP drug molecule photodegradation. They found that nanostructured CeO2 with different exposed planes has a site selective tendency for CIP molecule cleavage and yields different degraded products. In brief, the CIP molecule contains multiple functional groups; hence electrostatic potential maps show negative sites corresponding to nitrogen atoms and oxygen atoms of COOH termination and positive sites corresponding to low electron density aromatic rings and cyclopropyl groups as shown in Fig. 12(a). As we have already discussed in the above section, rod and bean morphologies with the (110) and (100) predominant surfaces have a more negative surface layer, hence show higher affinity towards the positive site of the CIP drug during the photodegradation process. On the other hand, the hexagon with the (311) exposed plane and the rod/cube morphology with the (111) exposed plane show good affinity for the negative site of the CIP molecule, as these morphologies contain a more positive surface layer.62 Further, a number of bismuth based materials along with other low-dimensional materials have been studied with CeO2 towards the elimination of different antibiotics.127,276–284 Recently an efficient hollow BiOCl@CeO2 heterostructured microsphere with type-II staggered-arrangement was reported for the photodegradation of an antibiotic, i.e. tetracycline (TC). The authors observed that the above heterostructure degraded 92% TC within 120 min owing to the distinctive hollow structures and better charge carrier transportation and separation. Due to the matched band energy between n-CeO2 and p-BiOCl an effective p–n junction was formed via Fermi level equilibration, which enhanced the interfacial charge transfer, because of which energetic electrons are now available at the CeO2 surface while holes are present at BiOCl. From the ESR trapping experiment, the authors concluded that both superoxide and hydroxyls were found to be active species, while the higher peak strength of DMPO–O2− confirmed the greater participation of superoxide radicals in TC degradation.276 Liu et al. presented a method of preparation of a 1D–2D heterostructure composite between shuttle like CeO2 and g-C3N4 nanosheets and tested its photocatalytic activity towards norfloxacin degradation. The group found that the composite exhibited higher mineralization efficiency, i.e. 63.8%, than that of neat 1D-CeO2 (31.8%) and g-C3N4 (39.4%) in a duration of 60 min. They found that gradual shedding of functional groups in norfloxacin molecules occurred during the course of reaction and ultimately the molecules decomposed into CO2 and H2O.282 A series of 2D/2D MoS2/CeO2 heterojunctions were successfully prepared by Ji et al. following a facile hydrothermal method. In comparison to neat MoS2 and CeO2, the optimized MoS2/CeO2 heterojunction (88.5%) exhibited higher photocatalytic performance for ciprofloxacin (CIP) degradation as shown in Fig. 12(b). The enhanced degradation efficiency of the MoS2/CeO2 heterojunction was attributed to its 2D/2D structure. Due to the presence of an effective 2D–2D interface, the photogenerated charge carriers were effectively separated and transferred, and meanwhile, the generated oxygen vacancies broadened the light absorption range of the heterojunction and overall both the factors helped to generate reactive oxidizing species for the degradation purpose (Fig. 12(c)). Further, they analyzed the CIP degradation pathway which can be described as follows: as CIP contains a piperazine ring, the generated hydroxyl radicals at first cleaved the piperazine ring to yield B (m/z = 348). Then the degradation reaction continued, where by losing the F and OH groups, C (m/z = 327) was formed. Further, the product D (m/z = 283) was formed from the subsequent cleavage of the carboxyl group. Meanwhile, E (m/z = 249) was formed by the cleavage of the carbon–carbon double bond adjacent to the carboxylic acid group. Finally, F (m/z = 133) was further degraded into smaller molecules, i.e. CO2 and H2O. The complete degradation pathway is given in Fig. 12(d).284
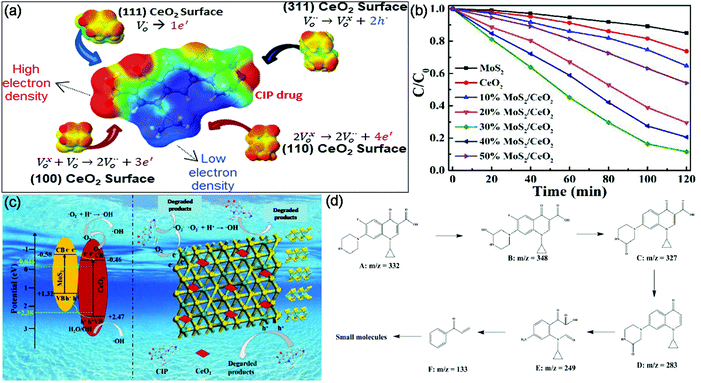 |
| Fig. 12 (a) Electrostatic potential maps showing different positive and negative sites for the ciprofloxacin molecule and the attacking affinity of (111), (311), (110), and (100) surfaces of CeO2 towards CIP. Reprinted with permission from ref. 62, copyright 2019 American Chemical Society. (b) Degradation efficiency of the CIP drug in the presence of different MoS2/CeO2 samples under visible light irradiation. (c) Schematic illustration of charge transfer and generation of active species for photodegradation by optimized MoS2/CeO2. (d) Proposed degradation pathway of the CIP drug. Reprinted from ref. 284 with permission from Elsevier. | |
Phenolic compound degradation to non-toxic products
Phenolic group organic materials are now the major threat to air, water and soil. In light of this, different CeO2 based materials were synthesized and tested for the effective removal of these pollutants. For example, Aslam et al. studied the photocatalytic degradation of phenolic compounds such as phenol, 2-bromophenol (bp), 2-chlorophenol (cp) and 2-nitrophenol (np) over a uniform particle sized (11 nm) CeO2 nanostructure upon exposure to the visible region (420–800 nm) and the complete spectrum of sunlight (Fig. 13(a)) and found an enhanced degradation rate in the full spectrum of sunlight. The exposure to direct sunlight enhanced the defect formation as the irradiated photons help the surface oxygen to escape from the CeO2 surface as shown in Fig. 13(b). The induction of defects composed of CeO2−x states which act as trap and transfer centers for the effective separation of electrons resulted in the formation of reactive oxidative species. Further, they found that Ce3+ played a key role in the generation of superoxide anion radicals (Fig. 13(c)) which were held responsible for complete mineralization of 2-NP and 2-CP, while less toxic aromatic intermediates were formed from phenol and 2-BP.105 Further, Ma et al. reported a type-II n–n heterostructure containing CeO2 over g-C3N4 nanosheets and tested its efficiency towards bisphenol-A degradation. Under visible light irradiation, only 65% removal efficiency was obtained for the g-C3N4 nanosheet and 14.4% for CeO2. However, the removal efficiency was found to be 93.7% at a time span of 80 min as shown in Fig. 13(d) with an apparent rate constant of 0.0199 min−1 for the type-II heterostructure. The higher efficiency of the heterostructure is mainly due to the efficient photogenerated charge transfer and separation process across the interface of the n–n junction. As the VB potential of n-type CNNS is unable to produce hydroxyl radicals, only the photogenerated superoxide combined with holes actively takes part in the degradation process as shown in Fig. 13(e).285 Recently, Gao et al. prepared a CeO2 incorporated ZnCo layered double hydroxide and employed it for phenol degradation in the presence of peroxymonosulfate. The generated oxidising species such as sulphate, hydroxyl, superoxide and holes were held responsible for the 99.8% degradation of phenol.286 Moreover, Chai et al. reported another 1D/2D heterostructure containing CeO2 NRs and g-C3N4 NSs and tested its efficiency towards oxidative coupling of benzylamine. Under UV-visible light irradiation, the 1D/2D heterostructure exhibited a 3 times higher oxidation rate constant compared to neat g-C3N4 and CeO2. They found that the presence of surface defects on CeO2 was the major reason behind the higher oxidation activity. In brief, the oxygen vacancy traps the oxygen and generates superoxide radicals which break to form hydroxyl species; along with the hydroxyl species, holes also take part in the oxidative coupling process.288
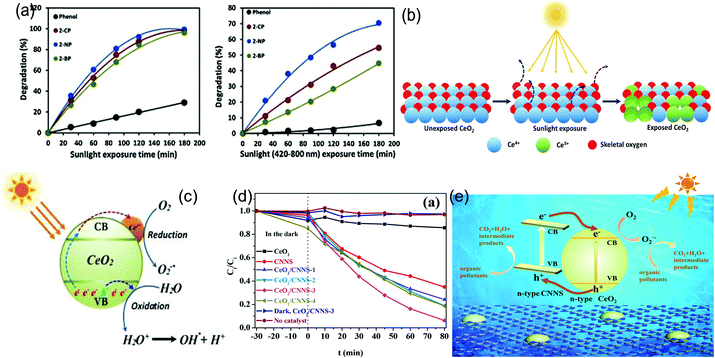 |
| Fig. 13 (a) Degradation curve of 50 ppm phenol, 2-CP, 2-BP, and 2-NP over CeO2 under direct sunlight and in the visible region (420–800 nm). (b) Schematic illustration of defect formation in CeO2 under sunlight exposure. (c) Schematic illustration of the generation of active species over defective CeO2. Reprinted from ref. 105 with permission from Elsevier. (d) Photocatalytic degradation of phenol in the presence of different CeO2–CNNS heterojunctions. (e) Plausible mechanism of charge transfer and photodegradation of phenol in CeO2 and CNNS n–n junction. Reprinted with permission from ref. 285, copyright 2019 American Chemical Society. | |
Heavy metal removal
Worldwide industrialization and technological advancement have brought a lot of troubles to the environment by releasing large quantities of heavy metals to the wastewater which represents a serious threat to global health. Now the heavy metal contaminants are beyond the recommended limit (Cr (0.1 mg L−1), Pb (0.015 mg L−1), As (0.010 mg L−1), Cd (0.005 mg L−1) and Hg (0.00003 mg L−1)) and are non-biodegradable which has attracted wide public concern. Unlike organic pollutants or dyes, heavy metals can’t be easily broken down biologically or chemically; however they can be reduced/transformed to a less toxic form.289–294 Hence, a variety of nanostructured CeO2 and CeO2 based heterostructures have been designed in this respect.295–304
To start with, Wu et al. synthesized CeO2 nanotubes (NTs) via the template-assisted method and tested their photocatalytic activity for Cr(VI) reduction in the presence of oxalic acid. Under UV light irradiation, the photocatalytic reduction efficiency of 100 ppm Cr(VI) reached up to 99.6% within 50 min (Fig. 14(a)) with an apparent rate constant of 0.10294 min−1. The effective reduction of Cr(VI) to Cr(III) was confirmed by the XPS spectra shown in Fig. 14(b) where a prominent Cr 2p peak corresponding to Cr(III) is visualised. The template directed CeO2 nanotube showed good potential towards chromium reduction because of the high photonic energy capture ability arising from its one dimensional characteristic and more importantly the oxalic acid–CeO2 surface complex formation. In brief, the small molecular weight oxalic acid formed a metal complex with the Ce of CeO2, and enabled intramolecular electron transfer from the oxalic acid ligand to the Ce metal leading to the formation of organic radicals promoting Cr(VI) reduction under light irradiation as shown in Fig. 14(c).75 Further, a lot of work has been reported on the photoreduction of Cr(VI) by using different CeO2 based materials. For example, Yang and co-workers reported 0D/2D CeO2 QDs/BiOX (X
=
Cl, Br) nanoplate heterojunctions. Under 5
W white LED light irradiation, the as-prepared 0D/2D heterojunction exhibited high photocatalytic performance not only for the reduction of Cr(VI) but also for the degradation of tetracycline (TC). After 1 h irradiation, the photoreduction rates of Cr(VI) over CeO2 QDs/BiOBr (CBB) and CBC were found to be 97% and 57% respectively with a pseudo first order kinetics as shown in Fig. 14(d and e). As compared to CeO2 QD decorated BiOBr, the BiOCl based composite possessed lower photoactivity due to its higher band gap and incapability of visible light absorption. However, the superior photocatalytic activity of the composite compared to pristine materials was attributed to mutual interaction of carriers leading to the efficient transfer and separation of photoexcited carriers and increment in the light absorption ability. Further, the formation of a strongly coupled interface between BiOX nanoplates and CeO2 QDs and inner Ce4+/Ce3+ redox centres enhances the activity further (Fig. 14(f)).297 Later by the same group, another heterojunction between CeO2 QDs and Bi2MoO6 (BMO) was reported which exhibited more efficient charge carrier transfer and separation capacity. Under light irradiation, the optimized CeO2–BMO heterojunction showed maximum Cr(VI) reduction (97%) within 90 min, which was higher than that of CeO2 (30%) and Bi2MoO6 (19%) respectively.298 Parida and his team recently reported a 1D–2D heterostructure between CeO2 nanorods and BiFeO3 nanosheets and in situ decorated nitrogen doped carbon over it. Interestingly, MCeO2–BFO was found to be the best photocatalyst (98.2%) for Cr(VI) reduction compared to the neat counterparts CeO2 (35%), MCeO2 (56%) and BFO (75%), which was attributed to the effective charge separation and transfer at the heterojunction.232 Li et al. fabricated sandwiched TiO2@Pt@CeO2 double shell hollow spheres and tested their photocatalytic performance towards hexavalent chromium reduction. Under solar light irradiation, they found the highest reduction rate for TiO2@Pt@CeO2 (1.901) as compared to neat TiO2 (1.040) and CeO2 (0.992). The enhanced photoreduction activity was mainly due to the unique sandwiched double shell structure and the presence of two oxides (TiO2 and CeO2). Further, the addition of the Pt co-catalyst (electron trap site) stores and shuttles the photogenerated excitons, and increases the formation of active radicals responsible for photoreduction reaction.300 Additionally, Kashinath and his unit reported a facile in situ fabrication method for the development of cerium oxide decorated graphene oxide (CeO2–GO) binary nanocomposites. The hexagonal nano CeO2 were embedded on the layered graphene oxide sheets via a simple microwave irradiation technique and the binary hybrid was tested for the removal of hexavalent chromium ions, dye degradation and antibacterial activities. Under UV (ultraviolet) light irradiation, the binary hybrid showed a rapid hexavalent chromium removal efficiency compared to neat GO and CeO2.301
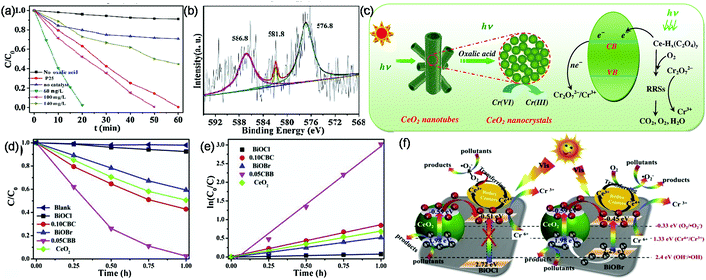 |
| Fig. 14 (a) Photoreduction activity of Cr(VI) solutions of different ppm over CeO2 nanotubes in the presence of oxalic acid. (b) High-resolution Cr 2p XPS spectra of the CeO2 catalyst after photoreduction. (c) Proposed scheme of the underlying mechanism of Cr(VI) photoreduction assisted by oxalic acid over CeO2 nanotubes under UV light irradiation.75 (d) and (e) The hexavalent chromium photoreduction curves and (e) the pseudo-first-order kinetics curves of BiOX, CeO2 and BiOX modified CeO2. (f) Schematic illustration of the photogenerated charge transfer and separation mechanism across CeO2/BiOCl and CeO2/BiOBr heterojunction photocatalysts.297 Reprinted with permission from Elsevier. | |
8. Conclusion and further prospective
Nanostructured CeO2 and CeO2 based heterostructure materials with distinct morphology, exposed planes/facets and interfaces have aroused extensive research interest across the globe due to their extraordinary properties and widespread application in the field of photocatalysis. In this review, we have systematically summarized various synthetic routes towards the fabrication of several morphology oriented CeO2 and its heterostructures as well as their application towards different solar energy conversion-based reactions (hydrogen production, O2 production, CO2 reduction and nitrogen reduction) and environmental pollutant (organic/inorganic) remediation. Additionally, theoretical aspects and experimental evidence behind the enhancement in the activity have been well explained. Although a large number of experimental and theoretical studies relating to nanostructured CeO2 and CeO2 based materials have been published in recent years, some fundamental aspects still need more attention. However, research in this area still faces a lot of challenges and encouragingly is filled with a lot of opportunities to explore new science. CeO2 has two key features, i.e. abundant oxygen defects and flexible oxidation state (Ce3+ ↔ Ce4+) shuttling ability, which make it a promising candidate for various photocatalytic applications. This rare earth oxide and its composites are also extensively studied for different electrocatalytic reactions because upon forming a hybrid, the number of active site increases which strongly influences the electronic structure of the other combining unit (novel metals, metal nitrides, phosphides, etc.) which ultimately improves the performance and durability of the system.
But in this review, we mainly focused on the photocatalytic aspect. However, the major shortcoming of CeO2 as a semiconductor photocatalyst is its low solar light absorption efficiency, fast carrier recombination and higher bandgap. Yet, intrinsic Ov have the ability to alter the band gap of CeO2 without causing serious damage to the crystal lattice. Hence, a low band gap CeO2 (3 to 2.6 eV) with a stable surface structure can bring about a revolution in the field of visible light driven photocatalysis. We have also discussed different modification techniques adopted for enhancing the catalytic efficiency of CeO2 and its composites by taking examples from reported literature.
Adding more to it, the oxygen vacancy is largely responsible for the higher photoactivity; however its controlled generation is very challenging and needs more experimental exploration. Further, the surface oxygen vacancy is very crucial for determining the tensile or compressive strain, which is also an issue for vacancy stabilization. For example, Wu et al. stated that tensile strain stabilizes the reduced states of ceria such as oxygen vacancies and surface hydroxyls, while compressive strain destabilizes the reduced states. In addition to these, there are many theoretical and experimental studies which have stated the qualitative influence of the oxygen vacancy and Ce3+, but have not stated how to control the amount of defects to achieve an optimum result. So the controlled generation of surface defects and inhibition of the bulk vacancy still suffer from the lack of a viable facile synthetic route. In order to know more about the electronic and geometric structure of surface vacancies over CeO2 based materials, more advanced techniques such as scanning tunnelling microscopy, HDAAF, XAFS, XANES, etc. should be used in more detail and in a regular manner. Further, the effect of Ov and the Ce3+/Ce4+ ratio on photocatalytic reactions needs more in situ characterization and computation studies. Additionally, current articles mainly give qualitative ideas on the effect of Ov and Ce3+ on catalytic efficiency but it needs direct confirmation which can be achieved by advanced in situ characterization and theoretical optimization that can develop a strong understanding of structure–activity interlinkage and hence guide researchers to design extraordinary photocatalytic systems. In brief, the precise synthesis of CeO2 based systems with a required amount of Ov and Ce3+/Ce4+ is very essential as it helps in providing useful information on the catalytic mechanism.
Considerable work has been done with regard to the preparation of morphology oriented CeO2 based materials and their catalytic as well as photocatalytic application. However, exposure of one facet or controlled growth of one or two facets changes the photoactivity drastically in comparison to the bulk nanostructure. For example, the exposed (100) plane is more suitable for dye degradation, while the (311) plane is more efficient for organic pollutant removal. Hence, this field needs more scientific attention. In this aspect, theoretical studies can show the path of designing desired exposed facet CeO2 based materials towards a particular type of photocatalytic reaction. In addition to the above stated modification, it is also quite effective to prepare specific nanostructured oriented CeO2 based systems such as core–shell, porous, hollow structures, hydrophilic/hydrophobic surfaces, etc. to achieve benchmark conversion efficiency.
Moreover, a viable, soft and precise synthetic route for designing nanostructured CeO2 and CeO2 based materials with higher yield also needs more attention. Different green, environmentally friendly approaches should be followed for the preparation of morphology oriented CeO2. In addition to using organic solvents and a lot of structure directing agents, common solvents like water, alcohol and biosurfactants should be used for the preparation of the above.
Further, in the preparation of CeO2 based heterostructures selection of a compatible lower dimensional other half photocatalyst with a distinctly shaped facet is also crucial for constructing an efficient photocatalytic system. So the design of a CeO2 based photocatalytic system with appropriate interfacial contact between CeO2 and the other photocatalyst still needs more study. This interface generally inhibits the photogenerated charge pair recombination, and hence the atomic level interaction of different nanostructures also needs more scientific research. In addition to this, the interfacial charge separation and transfer across the interface of two materials and the mechanism behind it are still in ambiguity. The charge transfer may be junctional type (p–n, n–n) or may be Z-scheme type or heterostructure type; hence more thorough theoretical as well as experimental evidence is required in this field. Moreover, coupling of CeO2 with other materials apart from conventional metal oxides, CdS, g-C3N4, and materials like metal phosphides, selenides, and borides can provide a better compatible partner for CeO2, and hence this field needs more experimental verification. Furthermore, the charge conducting ability of CeO2 framed systems can be increased by either integrating CeO2 with highly conductive substances or nucleating CeO2 on conductive materials like carbon cloth, metal foam, etc.
Theoretical analysis of different photocatalytic reactions especially water oxidation, CO2 reduction and active radical generation over normal and defective CeO2 with different exposed planes needs more systematic study. Further, there are a handful of attractive reports citing the photocatalytic dye degradation and organic pollutant oxidation, but very few studies have been carried out on photocatalytic water splitting and the NRR. However the oxygen vacancy enriched CeO2 has shown some outstanding results in photocatalytic water splitting. So, efficient Ov enriched CeO2 based catalysts with appropriate exposed facets, higher light absorption capacity and lower photogenerated charge recombination still need continuous research. Further, with the increasing efforts of the research community, we strongly believe that future CeO2 based photocatalytic systems will act as promising materials towards sustainable energy generation and environmental pollution abatement on an industrial scale.
Conflicts of interest
There are no conflicts to declare.
Acknowledgements
The authors are very much thankful to the SOA University management for their support and encouragement.
References
- J. Tian, Z. Zhao, A. Kumar, R. I. Boughton and H. Liu, Recent progress in design, synthesis, and applications of one-dimensional TiO2 nanostructured surface heterostructures: A review, Chem. Soc. Rev., 2014, 43, 6920–6937 RSC.
- C. Xu, P. R. Anusuyadevi, C. Aymonier, R. Luque and S. Marre, Nanostructured materials for photocatalysis, Chem. Soc. Rev., 2019, 48, 3868–3902 RSC.
- L. Mohapatra and K. Parida, A review of solar and visible light active oxo-bridged materials for energy and environment, Catal. Sci. Technol., 2017, 7, 2153–2164 RSC.
- P. Kuang, J. Low, B. Cheng, J. Yu and J. Fan, MXene-based photocatalysts, J. Mater. Sci. Technol., 2020, 56, 18 CrossRef.
- S. Wageh, A. A. Al-Ghamdi, R. Jafer, X. Li and P. Zhang, A new heterojunction in photocatalysis: S-scheme heterojunction, Chin. J. Catal., 2021, 42, 667 CrossRef CAS.
- X. Liu, S. Gu, Y. Zhao, G. Zhou and W. Li, Bi2WO6 and Bi2MoO6 photocatalysis: A brief review, J. Mater. Sci. Technol., 2020, 56, 45–68 CrossRef.
- Y. Li, M. Zhou, B. Cheng and Y. Shao, Recent advances in g-C3N4-based heterojunction photocatalysts, J. Mater. Sci. Technol., 2020, 56, 1–17 CrossRef.
- Y. Li, X. Li, H. Zhang, J. Fan and Q. Xiang, Design and application of active sites in g-C3N4-based photocatalysts, J. Mater. Sci. Technol., 2020, 56, 69–88 CrossRef.
- Y. Wang, Q. Wang, X. Zhan, F. Wang, M. Safdar and J. He, Visible light driven type II heterostructures and their enhanced photocatalysis properties: A review, Nanoscale, 2013, 5, 8326–8339 RSC.
- J. Baxter, Z. Bian, G. Chen, D. Danielson, M. S. Dresselhaus, A. G. Fedorov, T. S. Fisher, C. W. Jones, E. Maginn, U. Kortshagen and A. Manthiram, Nanoscale design to enable the revolution in renewable energy, Energy Environ. Sci., 2009, 2, 559–588 RSC.
- K. L. Materna, R. H. Crabtree and G. W. Brudvig, Anchoring groups for photocatalytic water oxidation on metal oxide surfaces, Chem. Soc. Rev., 2017, 46, 6099 RSC.
- S. Zhu and D. Wang, Photocatalysis: basic principles, diverse forms of implementations and emerging scientific opportunities, Adv. Energy Mater., 2017, 7, 1700841 CrossRef.
- K. M. Young, B. M. Klahr, O. Zandi and T. W. Hamann, Photocatalytic water oxidation with hematite electrodes, Catal. Sci. Technol., 2013, 3, 1660–1671 RSC.
- W. Tu, W. Guo, J. Hu, H. He, H. Li, Z. Li, W. Luo, Y. Zhou and Z. Zou, State-of-the-art advancements of crystal facet-exposed photocatalysts beyond TiO2: Design and dependent performance for solar energy conversion and environment applications, Mater. Today, 2020, 33, 75–86 CrossRef CAS.
- D. Jiang, W. Wang, L. Zhang, Y. Zheng and Z. Wang, Insights into the surface-defect dependence of photoreactivity over CeO2 nanocrystals with well-defined crystal facets, ACS Catal., 2015, 5, 4851–4858 CrossRef CAS.
- G. Liu, C. Y. Jimmy, G. Q. M. Lu and H. M. Cheng, Crystal facet engineering of semiconductor photocatalysts: Motivations, advances and unique properties, Chem. Commun., 2011, 47, 6763–6783 RSC.
- S. Wang, G. Liu and L. Wang, Crystal facet engineering of photoelectrodes for photoelectrochemical water splitting, Chem. Rev., 2019, 119, 5192–5247 CrossRef CAS PubMed.
- M. H. Huang, G. Naresh and H. S. Chen, Facet-dependent electrical, photocatalytic, and optical properties of semiconductor crystals and their implications for applications, ACS Appl. Mater. Interfaces, 2018, 10, 4–15 CrossRef CAS PubMed.
- W. Xu, Y. Bai and Y. Yin, Surface engineering of nanostructured energy materials, Adv. Mater., 2018, 30, 1802091 CrossRef PubMed.
- S. Bai, L. Wang, Z. Li and Y. Xiong, Facet-engineered surface and interface design of photocatalytic materials, Adv. Sci., 2017, 4, 1600216 CrossRef PubMed.
- S. Bai, W. Jiang, Z. Li and Y. Xiong, Surface and interface engineering in photocatalysis, ChemNanoMat, 2015, 1, 223–239 CrossRef CAS.
- M. D’Arienzo, J. Carbajo, A. Bahamonde, M. Crippa, S. Polizzi, R. Scotti, L. Wahba and F. Morazzoni, Photogenerated defects in shape-controlled TiO2 anatase nanocrystals: A probe to evaluate the role of crystal facets in photocatalytic processes, J. Am. Chem. Soc., 2011, 133, 17652–17661 CrossRef PubMed.
- M. R. D. Bomio, R. L. Tranquilin, F. V. D. Motta, C. A. Paskocimas, R. M. D. Nascimento, L. Gracia, J. Andres and E. Longo, Toward understanding the photocatalytic activity of PbMoO4 powders with predominant (111), (100), (011), and (110) facets. A combined experimental and theoretical study, J. Phys. Chem. C, 2013, 117(41), 21382–21395 CrossRef CAS.
- J. Zhu, F. Fan, R. Chen, H. An, Z. Feng and C. Li, Direct imaging of highly anisotropic photogenerated charge separations on different facets of a single BiVO4 photocatalyst, Angew. Chem., 2015, 127(31), 9239–9242 CrossRef.
- G. Naresh, P. L. Hsieh, V. Meena, S. K. Lee, Y. H. Chiu, M. Madasu, A. T. Lee, H. Y. Tsai, T. H. Lai, Y. J. Hsu and Y. C. Lo, Facet-dependent photocatalytic behaviors of ZnS-decorated Cu2O polyhedra arising from tunable interfacial band alignment, ACS Appl. Mater. Interfaces, 2018, 11(3), 3582–3589 CrossRef PubMed.
- L. Sun, L. Xiang, X. Zhao, C. J. Jia, J. Yang, Z. Jin, X. Cheng and W. Fan, Enhanced visible-light photocatalytic activity of BiOI/BiOCl heterojunctions: Key role of crystal facet combination, ACS Catal., 2015, 5(6), 3540–3551 CrossRef CAS.
- T. Montini, M. Melchionna, M. Monai and P. Fornasiero, Fundamentals and catalytic applications of CeO2-based materials, Chem. Rev., 2016, 116, 5987–6041 CrossRef CAS PubMed.
- J. Xiong, J. Di, J. Xia, W. Zhu and H. Li, Surface defect engineering in 2D nanomaterials for photocatalysis, Adv. Funct. Mater., 2018, 28, 1801983 CrossRef.
- X. Sun, X. Zhang and Y. Xie, Surface defects in two-dimensional photocatalysts for efficient organic synthesis, Matter, 2020, 2, 842–861 CrossRef.
- W. Zhou and H. Fu, Defect-mediated electron–hole separation in semiconductor photocatalysis, Inorg. Chem. Front., 2018, 5, 1240–1254 RSC.
- M. Kong, Y. Li, X. Chen, T. Tian, P. Fang, F. Zheng and X. Zhao, Tuning the relative concentration ratio of bulk defects to surface defects in TiO2 nanocrystals leads to high photocatalytic efficiency, J. Am. Chem. Soc., 2011, 133, 16414–16417 CrossRef CAS PubMed.
- F. Wang, W. Ge, T. Shen, B. Ye, Z. Fu and Y. Lu, The effect of bulk/surface defects ratio change on the photocatalysis of TiO2 nanosheet film, Appl. Surf. Sci., 2017, 410, 513–518 CrossRef CAS.
- F. Bai, L. Xu, X. Zhai, X. Chen and W. Yang, Vacancy in ultrathin 2D nanomaterials toward sustainable energy application, Adv. Energy Mater., 2020, 10, 1902107 CrossRef CAS.
- S. Bai, N. Zhang, C. Gao and Y. Xiong, Defect engineering in photocatalytic materials, Nano Energy, 2018, 53, 296–336 CrossRef CAS.
- Z. Zafar, S. Yi, J. Li, C. Li, Y. Zhu, A. Zada, W. Yao, Z. Liu and X. Yue, Recent development in defects engineered photocatalysts: An overview of the experimental and theoretical strategies, Energy Environ. Mater., 2021, 1–47 Search PubMed.
- H. Li, J. Shang, Z. Ai and L. Zhang, Efficient visible light nitrogen fixation with BiOBr nanosheets of oxygen vacancies on the exposed {001} facets, J. Am. Chem. Soc., 2015, 137(19), 6393–6399 CrossRef CAS PubMed.
- N. Zhang, X. Li, H. Ye, S. Chen, H. Ju, D. Liu, Y. Lin, W. Ye, C. Wang, Q. Xu and J. Zhu, Oxide defect engineering enables to couple solar energy into oxygen activation, J. Am. Chem. Soc., 2016, 138(28), 8928–8935 CrossRef CAS PubMed.
- S. Zhang, X. Liu, C. Liu, S. Luo, L. Wang, T. Cai, Y. Zeng, J. Yuan, W. Dong, Y. Pei and Y. Liu, MoS2 quantum dot growth induced by S vacancies in a ZnIn2S4 monolayer: Atomic-level heterostructure for photocatalytic hydrogen production, ACS Nano, 2018, 12(1), 751–758 CrossRef CAS PubMed.
- C. Sun, H. Li and L. Chen, Nanostructured ceria-based materials: Synthesis, properties, and applications, Energy Environ. Sci., 2012, 5, 8475–8505 RSC.
- E. Murray, T. Tsai and S. A. Barnett, A direct-methane fuel cell with a ceria-based anode, Nature, 1999, 400, 649 CrossRef CAS.
- Z. Shao, S. M. Haile, J. Ahn, D. Ronney, Z. Zhan and S. A. Barnett, A thermally self-sustained micro solid-oxide fuel-cell stack with high power density, Nature, 2005, 435, 795 CrossRef CAS PubMed.
- T. Morimoto, H. Tomonaga and A. Mitani, Ultraviolet ray absorbing coatings on glass for automobiles, Thin Solid Films, 1999, 351, 61–65 CrossRef CAS.
- S. Zhang, Z. Xia, Y. Zou, F. Cao, Y. Liu, Y. Ma and Y. Qu, Interfacial frustrated Lewis pairs of CeO2 activate CO2 for selective tandem transformation of olefins and CO2 into cyclic carbonates, J. Am. Chem. Soc., 2019, 141, 11353–11357 CrossRef CAS PubMed.
- M. Mogensen, N. M. Sammes and G. A. Tompsett, Physical, chemical and electrochemical properties of pure and doped ceria, Solid State Ionics, 2000, 129, 63–94 CrossRef CAS.
- D. Zhang, X. Du, L. Shi and R. Gao, Shape-controlled synthesis and catalytic application of ceria nanomaterials, Dalton Trans., 2012, 41, 14455–14475 RSC.
- Z. Wang and R. Yu, Hollow micro/nanostructured ceria-based materials: Synthetic strategies and versatile applications, Adv. Mater., 2018, 31, 1800592 CrossRef PubMed.
- S. Xie, Z. Wang, F. Cheng, P. Zhang, W. Mai and Y. Tong, Ceria and ceria-based nanostructured materials for photoenergy applications, Nano Energy, 2017, 34, 313–337 CrossRef CAS.
- J. Wang, X. Xiao, Y. Liu, K. Pan, H. Pang and S. Wei, The application of CeO2-based materials in electrocatalysis, J. Mater. Chem. A, 2019, 7, 17675–17702 RSC.
- J. Graciani, A. M. Márquez, J. J. Plata, Y. Ortega, N. C. Hernández, A. Meyer, C. M. Zicovich-Wilson and J. F. Sanz, Comparative study on the performance of hybrid DFT functionals in highly correlated oxides: The case of CeO2 and Ce2O3, J. Chem. Theory Comput., 2011, 7, 56–65 CrossRef CAS PubMed.
- Y. Ma, W. Gao, Z. Zhang, S. Zhang, Z. Tian, Y. Liu, J. C. Ho and Y. Qu, Regulating the surface of nanoceria and its applications in heterogeneous catalysis, Surf. Sci. Rep., 2018, 73, 1–36 CrossRef.
- F. A. Kröger and H. J. Vink, Relations between the concentrations of imperfections in crystalline solids, Solid State Phys., 1956, 3, 307–435 Search PubMed.
- B. Huang, R. Gillen and J. Robertson, Study of CeO2 and its native defects by density functional theory with repulsive potential, J. Phys. Chem. C, 2014, 118, 24248–24256 CrossRef CAS.
- Y. P. Lan and H. Y. Sohn, Effect of oxygen vacancies and phases on catalytic properties of hydrogen-treated nanoceria particles, Mater. Res. Express, 2018, 5, 035501 CrossRef.
- R. C. de Oliveira, R. A. C. Amoresi, N. L. Marana, M. A. Zaghete, M. Ponce, A. J. Chiquito, J. R. Sambrano, E. Longo and A. Z. Simoes, Influence of synthesis time on the morphology and properties of CeO2 nanoparticles: An experimental–theoretical study, Cryst. Growth Des., 2020, 20, 5031–5042 CrossRef CAS.
- Y. Huang, C.-F. Yan, C.-Q. Guo and Y. Shi, Experimental and first-principles DFT study on oxygen vacancies on cerium dioxide and its effect on enhanced photocatalytic hydrogen production, Int. J. Hydrogen Eng., 2016, 41, 7919–7926 CrossRef CAS.
- C. R. A. Catlow, Atomistic mechanisms of ionic transport in fast-ion conductors, Faraday Trans., 1990, 86, 1167–1176 RSC.
- F. Esch, S. Fabris, L. Zhou, T. Montini, C. Africh, P. Fornasiero, G. Comelli and R. Rosei, Electron localization determines defect formation on ceria substrates, Science, 2005, 309, 752–755 CrossRef CAS PubMed.
- R. C. Deus, R. A. C. Amoresi, P. M. Desimone, F. Schipani, L. S. R. Rocha, M. A. Ponce, A. Z. Simoes and E. Longo, Electrical behavior of cerium dioxide films exposed to different gases atmospheres, Ceram. Int., 2016, 42, 15023–15029 CrossRef CAS.
- S. Y. Chen, C. H. Tsai, M. Z. Huang, D. C. Yan, T. W. Huang, A. Gloter, C. L. Chen, H. J. Lin, C. T. Chen and C. L. Dong, Concentration dependence of oxygen vacancy on the magnetism of CeO2 nanoparticles, J. Phys. Chem. C, 2012, 116, 8707–8713 CrossRef CAS.
- Z. Liu, C. C. Sorrell, P. Koshy and J. N. Hart, DFT study of methanol adsorption on defect-free CeO2 low-index surfaces, ChemPhysChem, 2019, 20, 2074–2081 CrossRef CAS PubMed.
- M. Nolan, S. C. Parker and G. W. Watson, The electronic structure of oxygen vacancy defects at the low index surfaces of ceria, Surf. Sci., 2005, 595, 223–232 CrossRef CAS.
- R. A. Amoresi, R. C. Oliveira, N. L. Marana, P. B. De Almeida, P. S. Prata, M. A. Zaghete, E. Longo, J. R. Sambrano and A. Z. Simoes, CeO2 nanoparticle morphologies and their corresponding crystalline planes for the photocatalytic degradation of organic pollutants, ACS Appl. Nano Mater., 2019, 2, 6513–6526 CrossRef CAS.
- Z. Wu, M. Li, J. Howe, H. M. Meyer III and S. H. Overbury, Probing defect sites on CeO2 nanocrystals with well-defined surface planes by Raman spectroscopy and O2 adsorption, Langmuir, 2010, 26, 16595–16606 CrossRef CAS PubMed.
- X. Zheng, Y. Li, L. Zhang, L. Shen, Y. Xiao, Y. Zhang, C. Au and L. Jiang, Insight into the effect of morphology on catalytic performance of porous CeO2 nanocrystals for H2S selective oxidation, Appl. Catal., B, 2019, 252, 98–110 CrossRef CAS.
- Y. Xin, X. Yang, P. Jiang, Z. Zhang, Z. Wang and Y. Zhang, Synthesis of CeO2-based quantum dots through a polyol-hydrolysis method for fuel-borne catalysts, ChemCatChem, 2011, 3, 1772–1778 CrossRef CAS.
- M. S. Hassan, R. Khan, T. Amna, J. Yang, I. H. Lee, M. Y. Sun, M. H. EL-Newehy, S. S. Al-Deyab and M. S. Khil, The influence of synthesis method on size and toxicity of CeO2 quantum dots: Potential in the environmental remediation, Ceram. Int., 2016, 42, 576–582 CrossRef CAS.
- T. Lehnen, J. Schläfer and S. Mathur, Rapid microwave synthesis of CeO2 quantum dots, Z. Anorg. Allg. Chem., 2014, 640, 819–825 CrossRef CAS.
- D. Arumugam, M. Thangapian, A. Jayaram, G. S. Okram, N. P. Lalla and M. F. B. Amirtham, Induced aggregation of steric stabilizing anionic-rich 2-amino-3-chloro-5-trifluoromethylpyridine on CeO2 QDs: Surface charge and electro-osmotic flow analysis, J. Phys. Chem. C, 2016, 120, 26544–26555 CrossRef CAS.
- K. Zhao, J. Qi, H. Yin, Z. Wang, S. Zhao, X. Ma, J. Wan, L. Chang, Y. Gao, R. Yu and Z. Tang, Efficient water oxidation under visible light by tuning surface defects on ceria nanorods, J. Mater. Chem. A, 2015, 3, 20465–20470 RSC.
- F. Cao, M. Zhang, K. Yang, Z. Tian, J. Li and Y. Qu, Single crystalline CeO2 nanotubes, Nano Res., 2021, 14, 715–719 CrossRef CAS.
- B. Dong, L. Li, Z. Dong, R. Xu and Y. Wu, Fabrication of CeO2 nanorods for enhanced solar photocatalysts, Int. J. Hydrogen Energy, 2018, 43, 5275–5282 CrossRef CAS.
- S. C. Kuiry, S. D. Patil, S. Deshpe and S. Seal, Spontaneous self-assembly of cerium oxide nanoparticles to nanorods through supraaggregate formation, J. Phys. Chem. B, 2005, 109, 6936–6939 CrossRef CAS PubMed.
- K. L. Yu, G. L. Ruan, Y. H. Ben and J. Zou, Convenient synthesis of CeO2 nanotubes, J. Mater. Sci. Eng., B, 2007, 139, 197–200 CrossRef CAS.
- Y. J. Feng, L. L. Liu and X. D. Wang, Hydrothermal synthesis and automotive exhaust catalytic performance of CeO2 nanotube arrays, J. Mater. Chem., 2011, 21, 15442–15448 RSC.
- J. Wu, J. Wang, Y. Du, H. Li, Y. Yang and X. Jia, Chemically controlled growth of porous CeO2 nanotubes for Cr(VI) photoreduction, Appl. Catal., B, 2015, 174–175, 435–444 CrossRef CAS.
- X. Lu, T. Zhai, H. Cui, J. Shi, S. Xie, Y. Huang, C. Liang and Y. Tong, Redox cycles promoting photocatalytic hydrogen evolution of CeO2 nanorods, J. Mater. Chem., 2011, 21, 5569–5572 RSC.
- C. Zhang, X. Zhang, Y. Wang, S. Xie, Y. Liu, X. Lu and Y. Tong, Facile electrochemical synthesis of CeO2 hierarchical nanorods and nanowires with excellent photocatalytic activities, New J. Chem., 2014, 38, 2581–2586 RSC.
- T. Yu, B. Lim and Y. Xia, Aqueous-phase synthesis of single-crystal ceria nanosheets, Angew. Chem., Int. Ed., 2010, 49, 4484–4487 CrossRef CAS PubMed.
- Y. Yu, Y. Zhu and M. Meng, Preparation, formation mechanism and photocatalysis of ultrathin mesoporous single-crystal-like CeO2 nanosheets, Dalton Trans., 2013, 42, 12087–12092 RSC.
- Y. Huang, B. Long, M. Tang, Z. Rui, M. S. Balogun, Y. Tong and H. Ji, Bifunctional catalytic material: an ultrastable and high-performance surface defect CeO2 nanosheets for formaldehyde thermal oxidation and photocatalytic oxidation, Appl. Catal., B, 2016, 181, 779–787 CrossRef CAS.
- D. Wang, Y. Kang, V. Doan-Nguyen, J. Chen, R. Küngas, N. L. Wieder, K. Bakhmutsky, R. J. Gorte and C. B. Murray, Synthesis and oxygen storage capacity of two-dimensional ceria nanocrystals, Angew. Chem., Int. Ed., 2011, 50, 4378–4381 CrossRef CAS PubMed.
- T. Taniguchi, Y. Sonoda, M. Echikawa, Y. Watanabe, K. Hatakeyama, S. Ida, M. Koinuma and Y. Matsumoto, Intense photoluminescence from ceria-based nanoscale lamellar hybrid, ACS Appl. Mater. Interfaces, 2012, 4, 1010–1015 CrossRef CAS PubMed.
- H. Ding, J. Yang, S. Ma, N. Yigit, J. Xu, G. Rupprechter and J. Wang, Large dimensional CeO2 Nanoflakes by microwave-assisted synthesis: Lamellar nano-channels and surface oxygen vacancies promote catalytic activity, ChemCatChem, 2018, 10, 4100–4108 CrossRef CAS.
- P. Li, Y. Zhou, Z. Zhao, Q. Xu, X. Wang, M. Xiao and Z. Zou, Hexahedron prism-anchored octahedronal CeO2: Crystal facet-based homojunction promoting efficient solar fuel synthesis, J. Am. Chem. Soc., 2015, 137, 9547–9550 CrossRef CAS PubMed.
- N. S. Arul, D. Mangalaraj and J. I. Han, Solvothermal synthesis of three-dimensional CeO2 micropillows and their photocatalytic property, Phys. Status Solidi RRL, 2014, 8, 643–647 CrossRef CAS.
- C. Sun, J. Sun, G. Xiao, H. Zhang, X. Qiu, H. Li and L. Chen, Mesoscale organization of nearly monodisperse flowerlike ceria microspheres, J. Phys. Chem. B, 2006, 110, 13445–13452 CrossRef CAS PubMed.
- C. Sun, H. Li and L. Chen, Study of flowerlike CeO2 microspheres used as catalyst supports for CO oxidation reaction, J. Phys. Chem. Solids, 2007, 68, 1785–1790 CrossRef CAS.
- J. Sun, Y. Wang, J. Li, G. Xiao, L. Zhang, H. Li, Y. Cheng, C. Sun, Z. Cheng, Z. Dong and L. Chen, H2 production from stable ethanol steam reforming over catalyst of NiO based on flowerlike CeO2 microspheres, Int. J. Hydrogen Energy, 2010, 35, 3087–3091 CrossRef CAS.
- P. Ji, J. Zhang, F. Chen and M. Anpo, Ordered mesoporous CeO2 synthesized by nanocasting from cubic Ia3d mesoporous MCM-48 silica: Formation, characterization and photocatalytic activity, J. Phys. Chem. C, 2008, 112, 17809–17813 CrossRef CAS.
- S. Fang, Y. Xin, L. Ge, C. Han, P. Qiu and L. Wu, Facile synthesis of CeO2 hollow structures with controllable morphology by template-engaged etching of Cu2O and their visible light photocatalytic performance, Appl. Catal., B, 2015, 179, 458–467 CrossRef CAS.
- J. Qi, K. Zhao, G. Li, Y. Gao, H. Zhao, R. Yu and Z. Tang, Multi-shelled CeO2 hollow microspheres as superior photocatalysts for water oxidation, Nanoscale, 2014, 6, 4072–4077 RSC.
- N. Lv, J. Zhang, G. Li, X. Wang and J. Ni, Novel strategy for facile synthesis of C-shaped CeO2 nanotubes with enhanced catalytic properties, J. Phys. Chem. C, 2017, 121, 11926–11931 CrossRef CAS.
- H.-X. Mai, L.-D. Sun, Y.-W. Zhang, R. Si, W. Feng, H.-P. Zhang, H.-C. Liu and C.-H. Yan, Shape-selective synthesis and oxygen storage behavior of ceria nanopolyhedra, nanorods, and nanocubes, J. Phys. Chem. B, 2005, 109, 24380–24385 CrossRef CAS PubMed.
-
Q. Wu, F. Zhang, P. Xiao, H. Tao, X. Wang, Z. Hu and Y. Lü, Great influence of anions for controllable synthesis of CeO2 nanostructures: From nanorods to nanocubes. J. Phys. Chem. C, 2008, 112, 17076–17080 Search PubMed.
- C. Zhu, X. Wei, W. Li, Y. Pu, J. Sun, K. Tang, H. Wan, C. Ge, W. Zou and L. Dong, Crystal-Plane Effects of CeO2{110} and CeO2{100} on Photocatalytic CO2 Reduction: Synergistic Interactions of Oxygen Defects and Hydroxyl Groups, ACS Sustainable Chem. Eng., 2020, 8, 14397–14406 CrossRef CAS.
- D. Majumder, I. Chakraborty, K. Mandal and S. Roy, Facet-dependent photodegradation of methylene blue using pristine CeO2 nanostructures, ACS Omega, 2019, 4(2), 4243–4251 CrossRef CAS PubMed.
- H. Imagawa and S. Sun, Controlled synthesis of monodisperse CeO2 nanoplates developed from assembled nanoparticles, J. Phys. Chem. C, 2012, 116(4), 2761–2765 CrossRef CAS.
- H. L. Lin, C. Y. Wu and R. K. Chiang, Facile synthesis of CeO2 nanoplates and nanorods by [100] oriented growth, J. Colloid Interface Sci., 2010, 341(1), 12–17 CrossRef CAS PubMed.
- X. Hao, A. Yoko, C. Chen, K. Inoue, M. Saito, G. Seong, S. Takami, T. Adschiri and Y. Ikuhara, Atomic-scale valence state distribution inside ultrafine CeO2 nanocubes and its size dependence, Small, 2018, 14(42), 1802915 CrossRef PubMed.
- S. Yang and L. Gao, Controlled synthesis and self-assembly of CeO2 nanocubes, J. Am. Chem. Soc., 2006, 128(29), 9330–9331 CrossRef CAS PubMed.
- B. Liu, M. Yao, B. Liu, Z. Li, R. Liu, Q. Li, D. Li, B. Zou, T. Cui, G. Zou and J. Liu, High-pressure studies on CeO2 nano-octahedrons with a (111)-terminated surface., J. Phys. Chem. C, 2011, 115(11), 4546–4551 CrossRef CAS.
- T. Tsuzuki, R. He, A. Dodd and M. Saunders, Challenges in determining the location of dopants, to study the influence of metal doping on the photocatalytic activities of ZnO nanopowders, Nanomaterials, 2019, 9(3), 481 CrossRef CAS PubMed.
- Y. C. Zhang, Z. Li, L. Zhang, L. Pan, X. Zhang, L. Wang and J. J. Zou, Role of oxygen vacancies in photocatalytic water oxidation on ceria oxide: Experiment and DFT studies, Appl. Catal., B, 2018, 224, 101–108 CrossRef CAS.
- D. Jiang, W. Wang, E. Gao, S. Sun and L. Zhang, Highly selective defect-mediated photochemical CO2 conversion over fluorite ceria under ambient conditions, Chem. Commun., 2014, 50, 2005–2007 RSC.
- M. Aslam, M. T. Qamar, M. T. Soomro, I. M. Ismail, N. Salah, T. Almeelbi, M. A. Gondal and A. Hameed, The effect of sunlight induced surface defects on the photocatalytic activity of nanosized CeO2 for the degradation of phenol and its derivatives, Appl. Catal., B, 2016, 180, 391–402 CrossRef CAS.
- A. Hezam, K. Namratha, Q. A. Drmosh, D. Ponnamma, J. Wang, S. Prasad, M. Ahamed, C. Cheng and K. Byrappa, CeO2 nanostructures enriched with oxygen vacancies for photocatalytic CO2 reduction, ACS Appl. Nano Mater., 2019, 3, 138–148 CrossRef.
- D. Kandi, S. Martha and K. M. Parida, Quantum dots as enhancer in photocatalytic hydrogen evolution: a review, Int. J. Hydrogen Energy, 2017, 42, 9467–9481 CrossRef CAS.
- Y. N. Xia, P. D. Yang, Y. G. Sun, Y. Y. Wu, B. Mayers, B. Gates, Y. D. Yin, F. Kim and Y. Q. Yan, One-dimensional nanostructures: Synthesis, characterization, and applications, Adv. Mater., 2003, 15, 353–389 CrossRef CAS.
- J. Di, J. Xiong, H. Li and Z. Liu, Ultrathin 2D photocatalysts: Electronic-structure tailoring, hybridization, and applications, Adv. Mater., 2018, 30, 1704548 CrossRef PubMed.
- Y. Sakamoto, M. Kaneda, O. Terasaki, D. Y. Zhao, J. M. Kim, G. Stucky, H. J. Shin and R. Ryoo, Direct imaging of the pores and cages of three-dimensional mesoporous materials, Nature, 2000, 408, 449–453 CrossRef CAS PubMed.
- H. Du, Y. Wang, H. Ariyan, A. Younis, J. Scott, B. Qu, T. Wan, X. Lin, J. Chen and D. Chu, Design and synthesis of CeO2 nanowire/MnO2 nanosheet heterogeneous structure for enhanced catalytic properties, Mater. Today Commun., 2017, 11, 103–111 CrossRef CAS.
- C. Jiang, H. Wang, Y. Wang and H. Ji, All solid-state Z-scheme CeO2/ZnIn2S4 hybrid for the photocatalytic selective oxidation of aromatic alcohols coupled with hydrogen evolution, Appl. Catal., B, 2020, 277, 119235 CrossRef CAS.
- H. Jia, A. Du, H. Zhang, J. Yang, R. Jiang, J. Wang and C. Y. Zhang, Site-selective growth of crystalline ceria with oxygen vacancies on gold nanocrystals for near-infrared nitrogen photofixation, J. Am. Chem. Soc., 2019, 141, 5083–5086 CrossRef CAS PubMed.
- J. X. Feng, S. H. Ye, H. Xu, Y. X. Tong and G. R. Li, Design and synthesis of FeOOH/CeO2 heterolayered nanotube electrocatalysts for the oxygen evolution reaction, Adv. Mater., 2016, 28, 4698–4703 CrossRef CAS PubMed.
- Q. Ma, Q. Zhang, S. Chu, J. Guo, H. Li and Z. Lin, ZIF-67-induced double-tubular 1D CeO2/Co3O4 heterostructures allowing electron transfer synergetic mechanism for enhanced photocatalytic performance, Mater. Lett., 2021, 289, 129391 CrossRef CAS.
- X. J. Wen, C. Zhang, C. G. Niu, L. Zhang, G. M. Zeng and X. G. Zhang, Highly enhanced visible light photocatalytic activity of CeO2 through fabricating a novel p–n junction BiOBr/CeO2, Catal. Commun., 2017, 90, 51–55 CrossRef CAS.
- S. Sultana, S. Mansingh and K. M. Parida, Facile synthesis of CeO2 nanosheets decorated upon BiOI microplate: A surface oxygen vacancy promoted Z-scheme-based 2D-2D nanocomposite photocatalyst with enhanced photocatalytic activity, J. Phys. Chem. C, 2018, 122, 808–819 CrossRef CAS.
- R. Zhang, X. Ren, S. Hao, R. Ge, Z. Liu, A. M. Asiri, L. Chen, Q. Zhang and X. Sun, Selective phosphidation: an effective strategy toward CoP/CeO2 interface engineering for superior alkaline hydrogen evolution electrocatalysis, J. Mater. Chem. A, 2018, 6, 1985–1990 RSC.
- X. Yang, Y. Zhang, Y. Wang, C. Xin, P. Zhang, D. Liu, B. B. Mamba, K. K. Kefeni, A. T. Kuvarega and J. Gui, Hollow β-Bi2O3@ CeO2 heterostructure microsphere with controllable crystal phase for efficient photocatalysis, Chem. Eng. J., 2020, 387, 124100 CrossRef CAS.
- X. Gong, Y. Q. Gu, N. Li, H. Zhao, C. J. Jia and Y. Du, Thermally stable hierarchical nanostructures of ultrathin MoS2 nanosheet-coated CeO2 hollow spheres as catalyst for ammonia decomposition, Inorg. Chem., 2016, 55, 3992–3999 CrossRef CAS PubMed.
- M. Li, C. Chen, L. Xu, Y. Jia, Y. Liu and X. Liu, Surface defect-rich ceria quantum dots anchored on sulfur-doped carbon nitride nanotubes with enhanced charge separation for solar hydrogen production, J. Energy Chem., 2020, 52, 51–59 CrossRef.
- K. Wang, Y. Liang, J. Yang, G. Yang, Z. Zeng, R. Xu and X. Xie, Free-standing and flexible 0D CeO2 nanodot/1D La(OH)3 nanofiber heterojunction net as a novel efficient and easily recyclable photocatalyst, Inorg. Chem. Front., 2020, 7, 4701–4710 RSC.
- P. Xia, S. Cao, B. Zhu, M. Liu, M. Shi, J. Yu and Y. Zhang, Designing a 0D/2D S-scheme heterojunction over polymeric carbon nitride for visible-light photocatalytic inactivation of bacteria, Angew. Chem., Int. Ed., 2020, 59, 5218–5225 CrossRef CAS PubMed.
- M. Zhang, J. Yao, M. Arif, B. Qiu, H. Yin, X. Liu and S. M. Chen, 0D/2D CeO2/ZnIn2S4 Z-scheme heterojunction for visible-light-driven photocatalytic H2 evolution, Appl. Surf. Sci., 2020, 526, 145749 CrossRef CAS.
- H. Duan, T. Wang, X. Wu, Z. Su, J. Zhuang, S. Liu, R. Zhu, C. Chen and H. Pang, CeO2 quantum dots doped Ni-Co hydroxide nanosheets for ultrahigh energy density asymmetric supercapacitors, Chin. Chem. Lett., 2020, 31, 2330–2332 CrossRef CAS.
- Q. Li, Z. Yan, N. Wang, Z. Xu, G. Wang and G. Huang, 0D/2D CeO2 quantum dot/NiO nanoplate supported an ultralow-content Pt catalyst for the efficient oxidation of formaldehyde at room temperature, Catal. Sci. Technol., 2020, 10, 4030–4041 RSC.
- C. C. Hao, Y. B. Tang, W. L. Shi, F. Y. Chen and F. Guo, Facile solvothermal synthesis of a Z-Scheme 0D/3D CeO2/ZnIn2S4 heterojunction with enhanced photocatalytic performance under visible light irradiation, Chem. Eng. J., 2020, 409, 128168 CrossRef.
- D. Luo, B. Chen, X. Li, Z. Liu, X. Liu, X. Liu, C. Shi and X. S. Zhao, Three-dimensional nitrogen-doped porous carbon anchored CeO2 quantum dots as an efficient catalyst for formaldehyde oxidation, J. Mater. Chem. A, 2018, 6, 7897–7902 RSC.
- J. Qian, Z. Chen, H. Sun, F. Chen, X. Xu, Z. Wu, P. Li and W. Ge, Enhanced photocatalytic H2 production on three-dimensional porous CeO2/carbon nanostructure. ACS Sustain, Chem. Eng., 2018, 6, 9691–9698 RSC.
- Y. Ma, Y. Bian, Y. Liu, A. Zhu, H. Wu, H. Cui, D. Chu and J. Pan, Construction of Z-scheme system for enhanced photocatalytic H2 evolution based on CdS quantum dots/CeO2 nanorods heterojunction, ACS Sustainable Chem. Eng., 2018, 6, 2552–2562 CrossRef CAS.
- Y. Ma, P. Ou, Z. Wang, A. Zhu, L. Lu, Y. Zhang, W. Zeng, J. Song and J. Pan, Interface engineering in CeO2 (111) facets decorated with CdSe quantum dots for photocatalytic hydrogen evolution, J. Colloid Interface Sci., 2020, 579, 707–713 CrossRef CAS PubMed.
- S. Sultana, S. Mansingh, M. Scurrell and K. M. Parida, Controlled synthesis of CeO2NS–Au–CdSQDs ternary nanoheterostructure: A promising visible light responsive photocatalyst for H2 evolution, Inorg. Chem., 2017, 56, 12297–12307 CrossRef CAS PubMed.
- D. Ma, D. Sun, Y. Zou, S. Mao, Y. Lv, Y. Wang, J. Li and J. W. Shi, The synergy between electronic anchoring effect and internal electric field in CdS quantum dots decorated dandelion-like Fe–CeO2 nanoflowers for improved photocatalytic hydrogen evolution, J. Colloid Interface Sci., 2019, 549, 179–188 CrossRef CAS PubMed.
- B. Luo, G. Liu and L. Wang, Recent advances in 2D materials for photocatalysis, Nanoscale, 2016, 8, 6904–6920 RSC.
- N. Fajrina and M. Tahir, A critical review in strategies to improve photocatalytic water splitting towards hydrogen production, Int. J. Hydrogen Energy, 2019, 44, 540–577 CrossRef CAS.
- G. A. Olah, Beyond oil and gas: the methanol economy, Angew. Chem., Int. Ed., 2005, 44, 2636–2639 CrossRef CAS PubMed.
- Z. Liang, R. Shen, Y. H. Ng, P. Zhang, Q. Xiang and X. Li, A review on 2D MoS2 cocatalysts in photocatalytic H2 production, J. Mater. Sci. Technol., 2020, 56, 89 CrossRef.
- D. Ren, Z. Liang, Y. H. Ng, P. Zhang, Q. Xiang and X. Li, Strongly coupled 2D-2D nanojunctions between P-doped Ni2S (Ni2SP) cocatalysts and CdS nanosheets for efficient photocatalytic H2 evolution, Chem. Eng. J., 2020, 390, 124496 CrossRef CAS.
- C. Zhu, Y. Wang, Z. Jiang, F. Xu, Q. Xian, C. Sun, Q. Tong, W. Zou, X. Duan and S. Wang, CeO2 nanocrystal-modified layered MoS2/g-C3N4 as 0D/2D ternary composite for visible-light photocatalytic hydrogen evolution: Interfacial consecutive multi-step electron transfer and enhanced H2O reactant adsorption, Appl. Catal., B, 2019, 259, 118072 CrossRef CAS.
- Z. Jiang, Q. Chen, Q. Zheng, R. Shen, P. Zhang and X. Li, Constructing 1D/2D Schottky-based heterojunctions between Mn0.2Cd0.8S nanorods and Ti3C2 nanosheets for boosted photocatalytic H2 evolution, Acta Phys.-Chim. Sin., 2021, 37, 2010059 Search PubMed.
- H. Yu, Y. Zhao, C. Zhou, L. Shang, Y. Peng, Y. Cao, L. Z. Wu, C. H. Tung and T. Zhang, Carbon quantum dots/TiO2 composites for efficient photocatalytic hydrogen evolution, J. Mater. Chem. A, 2014, 2(10), 3344–3351 RSC.
- Z. Shao, X. Meng, H. Lai, D. Zhang, X. Pu, C. Su, H. Li, X. Ren and Y. Geng, Coralline-like Ni2P decorated novel tetrapod-bundle Cd0.9Zn0.1S ZB/WZ homojunctions for highly efficient visible-light photocatalytic hydrogen evolution, Chin. J. Catal., 2021, 42, 25 CrossRef.
- R. Shen, K. He, A. Zhang, N. Li, Y. H. Ng, P. Zhang, J. Hu and X. Li,
In situ construction of metallic Ni3C@ Ni core–shell cocatalysts over g-C3N4 nanosheets for shell-thickness-dependent photocatalytic H2 production, Appl. Catal., B, 2021, 291, 120104 CrossRef CAS.
- R. Shen, X. Lu, Q. Zheng, Q. Chen, Y. H. Ng, P. Zhang and X. Li, Tracking S-scheme charge transfer pathways in Mo2C/CdS H2-evolution photocatalysts, Sol. RRL, 2021, 2100177 CrossRef CAS.
- N. Xiao, S. Li, S. Liu, B. Xu, Y. Li, Y. Gao, L. Ge and G. Lu, Novel PtPd alloy nanoparticle-decorated g-C3N4 nanosheets with enhanced photocatalytic activity for H2 evolution under visible light irradiation, Chin. J. Catal., 2019, 40, 352–361 CrossRef CAS.
- M. K. Bhunia, K. Yamauchi and K. Takanabe, Harvesting solar light with crystalline carbon nitrides for efficient photocatalytic hydrogen evolution, Angew. Chem., 2014, 126(41), 11181–11185 CrossRef.
- Z. Hu, Z. Shen and C. Y. Jimmy, Phosphorus containing materials for photocatalytic hydrogen evolution, Green Chem., 2017, 19(3), 588–613 RSC.
- X. L. Li, X. J. Wang, J. Y. Zhu, Y. P. Li, J. Zhao and F. T. Li, Fabrication of two-dimensional Ni2P/ZnIn2S4 heterostructures for enhanced photocatalytic hydrogen evolution, Chem. Eng. J., 2018, 353, 15–24 CrossRef CAS.
- M. Wang, S. Shen, L. Li, Z. Tang and J. Yang, Effects of sacrificial reagents on photocatalytic hydrogen evolution over different photocatalysts, J. Mater. Sci., 2017, 52(9), 5155–5164 CrossRef CAS.
- L. Wang, C. Zhu, L. Yin and W. Huang, Construction of Pt–M (M = Co, Ni, Fe)/g-C3N4 Composites for Highly Efficient Photocatalytic H2 Generation, Acta Phys.-Chim. Sin., 2020, 36, 1907001 Search PubMed.
- S. Sun, X. Zhang, X. Liu, L. Pan, X. Zhang and J. Zou, Design and construction of cocatalysts for photocatalytic water splitting, Acta Phys.-Chim. Sin., 2020, 36, 1905007 Search PubMed.
- P. Jinbo, S. Sheng, Z. Wei, T. Jie, D. Hongzhi, W. Jinbo, C. Lang, A. Chak-Tong and Y. Shuang-Feng, Recent progress in photocatalytic hydrogen evolution, Acta Phys.-Chim. Sin., 2020, 36, 1905068 Search PubMed.
- M. Schwarze, D. Stellmach, M. Schröder, K. Kailasam, R. Reske, A. Thomas and R. Schomäcker, Quantification of photocatalytic hydrogen evolution, Phys. Chem. Chem. Phys., 2013, 15(10), 3466–3472 RSC.
- X. Wang, L. Chen, S. Y. Chong, M. A. Little, Y. Wu, W. H. Zhu, R. Clowes, Y. Yan, M. A. Zwijnenburg, R. S. Sprick and A. I. Cooper, Sulfone-containing covalent organic frameworks for photocatalytic hydrogen evolution from water, Nat. Chem., 2018, 10(12), 1180–1189 CrossRef CAS PubMed.
- L. Ge and C. Han, Synthesis of MWNTs/g-C3N4 composite photocatalysts with efficient visible light photocatalytic hydrogen evolution activity, Appl. Catal., B, 2012, 117, 268–274 CrossRef.
- G. Zhang, Z. A. Lan and X. Wang, Conjugated polymers: Catalysts for photocatalytic hydrogen evolution, Angew. Chem., Int. Ed., 2016, 55(51), 15712–15727 CrossRef PubMed.
- Z. Shao, X. Meng, H. Lai, D. Zhang, X. Pu, C. Su, H. Li, X. Ren and Y. Geng, Coralline-like Ni2P decorated novel tetrapod-bundle Cd0.9Zn0.1SZB/WZ homojunctions for highly efficient visible-light photocatalytic hydrogen evolution, Chin. J. Catal., 2021, 42, 439–449 CrossRef CAS.
- B. Chen, Y. Ma, L. Ding, L. Xu, Z. Wu, Q. Yuan and W. Huang, Reactivity of hydroxyls and water on a CeO2(111) thin film surface: The role of oxygen vacancy, J. Phys. Chem. C, 2013, 117, 5800–5810 CrossRef CAS.
- T. Wu, T. Vegge and H. A. Hansen, Improved electrocatalytic water splitting reaction on CeO2(111) by strain engineering: A DFT+U study, ACS Catal., 2019, 9, 4853–4861 CrossRef CAS.
- H. A. Hansen and C. Wolverton, Kinetics and thermodynamics of H2O dissociation on reduced CeO2(111), J. Phys. Chem. C, 2014, 118, 27402–27414 CrossRef CAS.
- D. R. Mullins, P. M. Albrecht, T. L. Chen, F. C. Calaza, M. D. Biegalski, H. M. Christen and S. H. Overbury, Water dissociation on CeO2(100) and CeO2(111) thin films, J. Phys. Chem. C, 2012, 116, 19419–19428 CrossRef CAS.
- D. Van Dao, T. T. Nguyen, T. D. Le, S. H. Kim, J. K. Yang, I. H. Lee and Y. T. Yu, Plasmonically driven photocatalytic hydrogen evolution activity of a Pt-functionalized Au@CeO2 core–shell catalyst under visible light, J. Mater. Chem. A, 2020, 8, 7687–7694 RSC.
- D. Van Dao, T. T. Nguyen, P. Uthirakumar, Y. H. Cho, G. C. Kim, J. K. Yang, D. T. Tran, T. D. Le, H. Choi, H. Y. Kim and Y. T. Yu, Insightful understanding of hot-carrier generation and transfer in plasmonic Au@CeO2 core–shell photocatalysts for light-driven hydrogen evolution improvement, Appl. Catal., B, 2021, 286, 119947 CrossRef.
- M. Sridharan, P. Kamaraj, M. Arthanareeswari, J. Arockiaselvi and E. Sundaravadivel, Quaternary CZTS nanoparticle decorated CeO2 as a noble metal free p–n heterojunction photocatalyst for efficient hydrogen evolution, Catal. Sci. Technol., 2019, 9, 3686–3696 RSC.
- D. You, B. Pan, F. Jiang, Y. Zhou and W. Su, CdS nanoparticles/CeO2 nanorods composite with high-efficiency visible-light-driven photocatalytic activity, Appl. Surf. Sci., 2016, 363, 154–160 CrossRef CAS.
- Y. Wang, X. Hao, L. Zhang, Y. Li and Z. Jin, Rational design of all-solid-state 0D/2D Mn0.2Cd0.8S/CeO2 direct Z-scheme for photocatalytic hydrogen evolution, Energy Fuels, 2020, 34, 2599–2611 CrossRef CAS.
- G. Swain, S. Sultana, B. Naik and K. Parida, Coupling of crumpled-type novel MoS2 with CeO2 nanoparticles: A noble-metal-free p–n heterojunction composite for visible light photocatalytic H2 production, ACS Omega, 2017, 2, 3745–3753 CrossRef CAS PubMed.
- Z. Cheng, F. Wang, T. A. Shifa, C. Jiang, Q. Liu and J. He, Efficient photocatalytic hydrogen evolution via band alignment tailoring: Controllable transition from type-I to type-II, Small, 2017, 13, 1702163 CrossRef PubMed.
- X. L. Wang, Y. Xiao, H. Yu, Y. Yang, X. T. Dong and L. Xia, Noble-metal-free MOF derived ZnS/CeO2 decorated with CuS cocatalyst photocatalyst with efficient photocatalytic hydrogen production character, ChemCatChem, 2020, 12, 5669–5678 CrossRef CAS.
- J. Hao, W. Zhan, L. Sun, G. Zhuang, X. Wang and X. Han, Combining N,S-codoped C and CeO2: A unique hinge-like structure for efficient photocatalytic hydrogen evolution, Inorg. Chem., 2019, 59, 937–942 CrossRef PubMed.
- X. Liu, L. He, X. Chen, L. Du, X. Gu, S. Wang, M. Fu, F. Dong and H. Huang, Facile synthesis of CeO2/g-C3N4 nanocomposites with significantly improved visible-light photocatalytic activity for hydrogen evolution, Int. J. Hydrogen Energy, 2019, 44, 16154–16163 CrossRef CAS.
- N. Gnanaseelan, M. Latha, A. Mantilla, K. Sathish-Kumar and F. Caballero-Briones, The role of redox states and junctions in photocatalytic hydrogen generation of MoS2–TiO2–rGO and CeO2–Ce2Ti3O8.7–TiO2–rGO composites, Mater. Sci. Semicond. Process., 2020, 118, 105185 CrossRef CAS.
- W. Zou, Y. Shao, Y. Pu, Y. Luo, J. Sun, K. Ma, C. Tang, F. Gao and L. Dong, Enhanced visible light photocatalytic hydrogen evolution via cubic CeO2 hybridized g-C3N4 composite, Appl. Catal., B, 2017, 218, 51–59 CrossRef CAS.
- W. Zou, B. Deng, X. Hu, Y. Zhou, Y. Pu, S. Yu, K. Ma, J. Sun, H. Wan and L. Dong, Crystal-plane-dependent metal oxide–support interaction in CeO2/g-C3N4 for photocatalytic hydrogen evolution, Appl. Catal., B, 2018, 238, 111–118 CrossRef CAS.
- C. H. Zeng, S. Xie, M. Yu, Y. Yang, X. Lu and Y. Tong, Facile synthesis of large-area CeO2/ZnO nanotube arrays for enhanced photocatalytic hydrogen evolution, J. Power Sources, 2014, 247, 545–550 CrossRef CAS.
- Y. Hao, L. Li, J. Zhang, H. Luo, X. Zhang and E. Chen, Multilayer and open structure of dendritic crosslinked CeO2–ZrO2 composite: Enhanced photocatalytic degradation and water splitting performance, Int. J. Hydrogen Energy, 2017, 42, 5916–5929 CrossRef CAS.
- C. H. Shen, X. J. Wen, Z. H. Fei, Z. T. Liu and Q. M. Mu, Novel Z-scheme W18O49/CeO2 heterojunction for improved photocatalytic hydrogen evolution, J. Colloid Interface Sci., 2020, 579, 297–306 CrossRef CAS PubMed.
- P. Li, M. Zhang, X. Li, C. Wang, R. Wang, B. Wang and H. Yan, MOF-derived NiO/CeO2 heterojunction: a photocatalyst for degrading pollutants and hydrogen evolution, J. Mater. Sci., 2020, 55, 15930–15944 CrossRef CAS.
- X. Cui, Z. Liu, G. Li, M. Zhang, Y. Song and J. Wang, Self-generating CeVO4 as conductive channel within CeO2/CeVO4/V2O5 to induce Z-scheme-charge-transfer driven photocatalytic degradation coupled with hydrogen production, Int. J. Hydrogen Energy, 2019, 44, 23921–23935 CrossRef CAS.
- C. Zhu, Q. Xian, Q. He, C. Chen, W. Zou, C. Sun, S. Wang and X. Duan, Edge-rich bicrystalline 1T/2H-MoS2 cocatalyst-decorated{110} terminated CeO2 nanorods for photocatalytic hydrogen evolution, ACS Appl. Mater. Interfaces, 2021, 13(30), 35818–35827 CrossRef CAS PubMed.
- J. Qian, Z. Chen, F. Chen, Y. Wang, Z. Wu, W. Zhang, Z. Wu and P. Li, Exploration of CeO2–CuO quantum dots in situ grown on graphene under hypha assistance for highly efficient solar-driven hydrogen production, Inorg. Chem., 2018, 57, 14532–14541 CrossRef CAS PubMed.
- Y. Hao, L. Li, D. Liu, H. Yu and Q. Zhou, The synergy of SPR effect and Z-scheme of Ag on enhanced photocatalytic performance of 3DOM Ag/CeO2-ZrO2 composite, Mol. Catal., 2018, 447, 37–46 CrossRef CAS.
- A. Hezam, J. Wang, Q. A. Drmosh, P. Karthik, M. A. Bajiri, K. Namratha, M. Zare, T. R. Lakshmeesha, S. Shivanna, C. Cheng and B. Neppolian, Rational construction of plasmonic Z-scheme Ag–ZnO–CeO2 heterostructures for highly enhanced solar photocatalytic H2 evolution, Appl. Surf. Sci., 2020, 541, 148457 CrossRef.
- H. Yu, J. Xu, Z. Liu, Y. Li and Z. Jin, Functionalization of sheet structure MoS2 with CeO2–Co3O4 for efficient photocatalytic hydrogen evolution, J. Mater. Sci., 2018, 53, 15271–15284 CrossRef CAS.
- M. A. Sha, P. C. Meenu, V. S. Sumi, T. C. Bhagya, B. R. Sreelekshmy and S. M. A. Shibli, Tuning of electron transfer by Ni–P decoration on CeO2–TiO2 heterojunction for enhancement in photocatalytic hydrogen generation, Mater. Sci. Semicond. Process., 2020, 105, 104742 CrossRef.
- S. Chen, J. Duan, P. Bian, Y. Tang, R. Zheng and S. Z. Qiao, Three-dimensional smart catalyst electrode for oxygen evolution reaction, Adv. Energy Mater., 2015, 5, 1500936 CrossRef.
- L. Yang, H. Zhou, T. Fan and D. Zhang, Semiconductor photocatalysts for water oxidation: Current status and challenges, Phys. Chem. Chem. Phys., 2014, 16, 6810–6826 RSC.
- Y. Gorlin and T. F. Jaramillo, A bifunctional nonprecious metal catalyst for oxygen reduction and water oxidation, J. Am. Chem. Soc., 2010, 132(39), 13612–13614 CrossRef CAS PubMed.
- G. Chen, D. A. Delafuente, S. Sarangapani and T. E. Mallouk, Combinatorial discovery of bifunctional oxygen reduction—water oxidation electrocatalysts for regenerative fuel cells, Catal. Today, 2001, 67(4), 341–355 CrossRef CAS.
- J. Liu, Y. Zou, B. Jin, K. Zhang and J. H. Park, Hydrogen peroxide production from solar water oxidation, ACS Energy Lett., 2019, 4(12), 3018–3027 CrossRef CAS.
- A. R. Jadhav, J. M. C. Puguan and H. Kim, Microwave-assisted synthesis of a stainless steel mesh-supported Co3O4 microrod array as a highly efficient catalyst for electrochemical water oxidation, ACS Sustainable Chem. Eng., 2017, 5(11), 11069–11079 CrossRef CAS.
- J. W. D. Ng, M. Tang and T. F. Jaramillo, A carbon-free, precious-metal-free, high-performance O2 electrode for regenerative fuel cells and metal–air batteries, Energy Environ. Sci., 2014, 7(6), 2017–2024 RSC.
- A. Vignesh, M. Prabu and S. Shanmugam, Porous LaCo1−xNixO3−δ nanostructures as an efficient electrocatalyst for water oxidation and for a zinc–air battery, ACS Appl. Mater. Interfaces, 2016, 8(9), 6019–6031 CrossRef CAS PubMed.
- K. J. Young, L. A. Martini, R. L. Milot, R. C. Snoeberger III, V. S. Batista, C. A. Schmuttenmaer, R. H. Crabtree and G. W. Brudvig, Light-driven water oxidation for solar fuels, Coord. Chem. Rev., 2012, 256(21–22), 2503–2520 CrossRef CAS PubMed.
- H. Dau and I. Zaharieva, Principles, efficiency, and blueprint character of solar-energy conversion in photosynthetic water oxidation, Acc. Chem. Res., 2009, 42(12), 1861–1870 CrossRef CAS PubMed.
- X. Fan, M. S. Balogun, Y. Huang and Y. Tong, Oxygen-deficient three-dimensional porous Co3O4 nanowires as an electrode material for water oxidation and energy storage, ChemElectroChem, 2017, 4(10), 2453–2459 CrossRef CAS.
- B. He, P. Kuang, X. Li, H. Chen, J. Yu and K. Fan, In Situ transformation of Prussian-blue analogue-derived bimetallic carbide nanocubes by water oxidation: Applications for energy storage and conversion, Chem. – Eur. J., 2020, 26(18), 4052–4062 CAS.
- A. Primo, T. Marino, A. Corma, R. Molinari and H. Garcia, Efficient visible-light photocatalytic water splitting by minute amounts of gold supported on nanoparticulate CeO2 obtained by a biopolymer templating method, J. Am. Chem. Soc., 2011, 133, 6930–6933 CrossRef CAS PubMed.
- S. Fang, S. Li, L. Ge, C. Han, P. Qiu and Y. Gao, Synthesis of novel CoOx decorated CeO2 hollow structures with an enhanced photocatalytic water oxidation performance under visible light irradiation, Dalton Trans., 2017, 46, 10578–10585 RSC.
- S. Sultana, S. Mansingh and K. M. Parida, Rational design of light induced self healed Fe based oxygen vacancy rich CeO2(CeO2 NS–FeOOH/Fe2O3) nanostructure materials for photocatalytic water oxidation and Cr(VI) reduction, J. Mater. Chem. A, 2018, 6, 11377–11389 RSC.
- J. Meng, Y. Zhao, H. Li, R. Chen, X. Sun and X. Sun, Metalloporphyrin immobilized CeO2: In situ generation of active sites and synergistic promotion of photocatalytic water oxidation, Catal. Sci. Technol., 2021, 11, 2560–2569 RSC.
- X. Li, J. Yu, M. Jaroniec and X. Chen, Cocatalysts for selective photoreduction of CO2 into solar fuels, Chem. Rev., 2019, 119, 3962–4179 CrossRef CAS PubMed.
- D. Voiry, H. S. Shin, K. Loh and M. Chhowalla, Low-dimensional catalysts for hydrogen evolution and CO2 reduction, Nat. Rev. Chem., 2018, 2, 1–17 CrossRef.
- N. Kumari, M. A. Haider, M. Agarwal, N. Sinha and S. Basu, Role of reduced CeO2(110) surface for CO2 reduction to CO and methanol, J. Phys. Chem. C, 2016, 120, 16626–16635 CrossRef CAS.
- N. Kumari, N. Sinha, M. A. Haider and S. Basu, CO2 reduction to methanol on CeO2(110) surface: A density functional theory study, Electrochim. Acta, 2015, 177, 21–29 CrossRef CAS.
- S. Ullah, E. C. Lovell, R. J. Wong, T. H. Tan, J. Scott and R. Amal, Light-enhanced CO2 reduction to CH4 using nonprecious transition-metal catalysts, ACS Sustainable Chem. Eng., 2020, 8, 5056–5066 CrossRef CAS.
- Y. Wang, B. Li, C. Zhang, L. Cui, S. Kang, X. Li and L. Zhou, Ordered mesoporous CeO2–TiO2 composites: Highly efficient photocatalysts for the reduction of CO2 with H2O under simulated solar irradiation, Appl. Catal., B, 2013, 130, 277–284 CrossRef.
- J. Jiao, Y. Wei, Z. Zhao, J. Liu, J. Li, A. Duan and G. Jiang, Photocatalysts of 3D ordered macroporous TiO2-supported CeO2 nanolayers: Design, preparation, and their catalytic performances for the reduction of CO2 with H2O under simulated solar irradiation, Ind. Eng. Chem. Res., 2014, 53, 17345–17354 CrossRef CAS.
- Z. Xiong, Z. Lei, Z. Xu, X. Chen, B. Gong, Y. Zhao, H. Zhao, J. Zhang and C. Zheng, Flame spray pyrolysis synthesized ZnO/CeO2 nanocomposites for enhanced CO2 photocatalytic reduction under UV-Vis light irradiation, J. CO2 Util., 2017, 18, 53–61 CrossRef CAS.
- Y. Pu, Y. Luo, X. Wei, J. Sun, L. Li, W. Zou and L. Dong, Synergistic effects of Cu2O-decorated CeO2 on photocatalytic CO2 reduction: Surface Lewis acid/base and oxygen defect, Appl. Catal., B, 2019, 254, 580–586 CrossRef CAS.
- W. Dai, X. Hu, T. Wang, W. Xiong, X. Luo and J. Zou, Hierarchical CeO2/Bi2MoO6 heterostructured nanocomposites for photoreduction of CO2 into hydrocarbons under visible light irradiation, Appl. Surf. Sci., 2018, 434, 481–491 CrossRef CAS.
- S. Ijaz, M. F. Ehsan, M. N. Ashiq, N. Karamat and T. He, Preparation of CdS@ CeO2 core/shell composite for photocatalytic reduction of CO2 under visible-light irradiation, Appl. Surf. Sci., 2016, 390, 550–559 CrossRef CAS.
- C. Yang, Q. Li, Y. Xia, K. Lv and M. Li, Enhanced visible-light photocatalytic CO2 reduction performance of Znln2S4 microspheres by using CeO2 as cocatalyst, Appl. Surf. Sci., 2019, 464, 388–395 CrossRef CAS.
- M. Liang, T. Borjigin, Y. Zhang, B. Liu, H. Liu and H. Guo, Controlled assemble of hollow heterostructured g-C3N4@ CeO2 with rich oxygen vacancies for enhanced photocatalytic CO2 reduction, Appl. Catal., B, 2019, 243, 566–575 CrossRef CAS.
- M. Li, L. Zhang, M. Wu, Y. Du, X. Fan, M. Wang, L. Zhang, Q. Kong and J. Shi, Mesostructured CeO2/g-C3N4 nanocomposites: Remarkably enhanced photocatalytic activity for CO2 reduction by mutual component activations, Nano Energy, 2016, 19, 145–155 CrossRef CAS.
- X. Zhao, J. Guan, J. Li, X. Li, H. Wang, P. Huo and Y. Yan, CeO2/3D g-C3N4 heterojunction deposited with Pt cocatalyst for enhanced photocatalytic CO2 reduction, Appl. Surf. Sci., 2021, 537, 147891 CrossRef CAS.
- S. Q. Liu, S. S. Zhou, Z. G. Chen, C. B. Liu, F. Chen and Z. Y. Wu, An artificial photosynthesis system based on CeO2 as light harvester and N-doped graphene Cu(II) complex as artificial metalloenzyme for CO2 reduction to methanol fuel, Catal. Commun., 2016, 73, 7–11 CrossRef CAS.
- T. Ye, W. Huang, L. Zeng, M. Li and J. Shi, CeO2−x platelet from monometallic cerium layered double hydroxides and its photocatalytic reduction of CO2, Appl. Catal., B, 2017, 210, 141–148 CrossRef CAS.
- C. Mao, J. Wang, Y. Zou, H. Li, G. Zhan, J. Li, J. Zhao and L. Zhang, Anion (O, N, C, and S) vacancies promoted photocatalytic nitrogen fixation, Green Chem., 2019, 21, 2852–2867 RSC.
- X. Chen, N. Li, Z. Kong, W. J. Ong and X. Zhao, Photocatalytic fixation of nitrogen to ammonia: State-of-the-art advancements and future prospects, Mater. Horiz., 2018, 5, 9–27 RSC.
- R. Zhang, X. Ren, X. Shi, F. Xie, B. Zheng, X. Guo and X. Sun, Enabling effective electrocatalytic N2 conversion to NH3 by the TiO2 nanosheets array under ambient conditions, ACS Appl. Mater. Interfaces, 2018, 10, 28251–28255 CrossRef CAS PubMed.
- L. Ye, C. Han, Z. Ma, Y. Leng, J. Li, X. Ji, D. Bi, H. Xie and Z. Huang, Ni2P loading on Cd0.5Zn0.5S solid solution for exceptional photocatalytic nitrogen fixation under visible light, Chem. Eng. J., 2017, 307, 311–318 CrossRef CAS.
- S. Sun, X. Li, W. Wang, L. Zhang and X. Sun, Photocatalytic robust solar energy reduction of dinitrogen to ammonia on ultrathin MoS2, Appl. Catal., B, 2017, 200, 323–329 CrossRef CAS.
- Y. Zhao, Y. Zhao, G. I. Waterhouse, L. Zheng, X. Cao, F. Teng, L. Z. Wu, C. H. Tung, D. O'Hare and T. Zhang, Layered-double-hydroxide nanosheets as efficient visible-light-driven photocatalysts for dinitrogen fixation, Adv. Mater., 2017, 29, 1703828 CrossRef PubMed.
- S. Mukherjee, D. A. Cullen, S. Karakalos, K. Liu, H. Zhang, S. Zhao, H. Xu, K. L. More, G. Wang and G. Wu, Metal–organic framework-derived nitrogen-doped highly disordered carbon for electrochemical ammonia synthesis using N2 and H2O in alkaline electrolytes, Nano Energy, 2018, 48, 217–226 CrossRef CAS.
- J. Qi, S. Zhou, K. Xie and S. Lin, Catalytic role of assembled Ce Lewis acid sites over ceria for electrocatalytic conversion of dinitrogen to ammonia, J. Energy Chem., 2021, 60, 249–258 CrossRef.
- B. Xu, L. Xia, F. Zhou, R. Zhao, H. Chen, T. Wang, Q. Zhou, Q. Liu, G. Cui, X. Xiong and F. Gong, Enhancing electrocatalytic N2 reduction to NH3 by CeO2 nanorod with oxygen vacancies, ACS Sustainable Chem. Eng., 2019, 7, 2889–2893 CrossRef CAS.
- H. Xie, H. Wang, Q. Geng, Z. Xing, W. Wang, J. Chen, L. Ji, L. Chang, Z. Wang and J. Mao, Oxygen vacancies of Cr-doped CeO2 nanorods that efficiently enhance the performance of electrocatalytic N2 fixation to NH3 under ambient conditions, Inorg. Chem., 2019, 58, 5423–5427 CrossRef CAS PubMed.
- H. Xie, Q. Geng, X. Li, T. Wang, Y. Luo, A. A. Alshehri, K. A. Alzahrani, B. Li, Z. Wang and J. Mao, Ceria-reduced graphene oxide nanocomposite as an efficient electrocatalyst towards artificial N2 conversion to NH3 under ambient conditions, Chem. Commun., 2019, 55, 10717–10720 RSC.
- X. Feng, H. Chen, F. Jiang and X. Wang, One-pot fabrication of a double Z-scheme CeCO3OH/gC3N4/CeO2 photocatalyst for nitrogen fixation under solar irradiation, Catal. Sci. Technol., 2019, 9, 2849–2857 RSC.
- S. Sultana, S. Mansingh and K. M. Parida, Phosphide protected FeS2 anchored oxygen defect oriented CeO2 NS based ternary hybrid for electrocatalytic and photocatalytic N2 reduction to NH3, J. Mater. Chem. A, 2019, 7, 9145–9153 RSC.
- S. Mansingh, S. Sultana, R. Acharya, M. K. Ghosh and K. M. Parida, Efficient photon conversion via double charge dynamics CeO2–BiFeO3 p–n heterojunction photocatalyst promising toward N2 fixation and phenol–Cr(VI) detoxification, Inorg. Chem., 2020, 59, 3856–3873 CrossRef CAS PubMed.
- X. Li, J. Xie, C. Jiang, J. Yu and P. Zhang, Review on design and evaluation of environmental photocatalysts, Front. Environ. Sci. Eng., 2018, 12, 1–32 CrossRef CAS.
- V. Hasija, P. Raizada, A. Sudhaik, K. Sharma, A. Kumar, P. Singh, S. B. Jonnalagadda and V. K. Thakur, Recent advances in noble metal free doped graphitic carbon nitride based nanohybrids for photocatalysis of organic contaminants in water: A review, Appl. Mater. Today, 2019, 15, 494–524 CrossRef.
-
M. L. Brusseau and J. F. Artiola, Chemical contaminants, in Environmental and pollution science, Academic Press, 2019, pp. 175–190 Search PubMed.
- N. Warren, I. J. Allan, J. E. Carter, W. A. House and A. Parker, Pesticides and other micro-organic contaminants in freshwater sedimentary environments—A review, Appl. Geochem., 2003, 18(2), 159–194 CrossRef CAS.
- P. Gu, J. Xing, T. Wen, R. Zhang, J. Wang, G. Zhao, T. Hayat, Y. Ai, Z. Lin and X. Wang, Experimental and theoretical calculation investigation on efficient Pb(II) adsorption on etched Ti3AlC2 nanofibers and nanosheets, Environ. Sci.: Nano, 2018, 5, 946–955 RSC.
- R. Ma, L. Yin, L. Li, S. Zhang, T. Wen, C. Zhang, X. Wang, Z. Chen, T. Hayat and X. Wang, Comparative investigation of Fe2O3 and Fe1−xS nanostructures for uranium decontamination, ACS Appl. Nano Mater., 2018, 1, 5543–5552 CrossRef CAS.
- P. Gu, S. Zhang, X. Li, X. Wang, T. Wen, R. Jehan, A. Alsaedi, T. Hayat and X. Wang, Recent advances in layered double hydroxide-based nanomaterials for the removal of radionuclides from aqueous solution, Environ. Pollut., 2018, 240, 493–505 CrossRef CAS PubMed.
- C. C. Wang, J. R. Li, X. L. Lv, Y. Q. Zhang and G. Guo, Photocatalytic organic pollutants degradation in metal–organic frameworks, Energy Environ. Sci., 2014, 7, 2831–2867 RSC.
- M. Hepel and J. Luo, Photoelectrochemical mineralization of textile diazo dye pollutants using nanocrystalline WO3 electrodes, Electrochim. Acta, 2001, 47(5), 729–740 CrossRef CAS.
- M. L. Marin, L. Santos-Juanes, A. Arques, A. M. Amat and M. A. Miranda, Organic photocatalysts for the oxidation of pollutants and model compounds, Chem. Rev., 2012, 112(3), 1710–1750 CrossRef CAS PubMed.
- P. Singh and A. Borthakur, A review on biodegradation and photocatalytic degradation of organic pollutants: A bibliometric and comparative analysis, J. Clean. Prod., 2018, 196, 1669–1680 CrossRef CAS.
- M. Zhang, Q. Shi, X. Song, H. Wang and Z. Bian, Recent electrochemical methods in electrochemical degradation of halogenated organics: A review, Environ. Sci. Pollut. Res., 2019, 26(11), 10457–10486 CrossRef CAS PubMed.
- Y. L. Pang, A. Z. Abdullah and S. Bhatia, Review on sonochemical methods in the presence of catalysts and chemical additives for treatment of organic pollutants in wastewater, Desalination, 2011, 277, 1–14 CrossRef CAS.
- M. Sillanpää, M. C. Ncibi and A. Matilainen, Advanced oxidation processes for the removal of natural organic matter from drinking water sources: A comprehensive review, J. Environ. Manage., 2018, 208, 56–76 CrossRef PubMed.
- G. Darabdhara, K. Boruah, P. Borthakur, N. Hussain, M. R. Das, T. Ahamad, S. M. Alshehri, V. Malgras, K. C. W. Wu and Y. Yamauchi, Reduced graphene oxide nanosheets decorated with Au–Pd bimetallic alloy nanoparticles towards efficient photocatalytic degradation of phenolic compounds in water, Nanoscale, 2016, 8, 8276–8287 RSC.
- D. Chatterjee and S. Dasgupta, Visible light induced photocatalytic degradation of organic pollutants, J. Photochem. Photobiol., C, 2005, 6(2–3), 186–205 CrossRef CAS.
- N. Sabari Arul, D. Mangalaraj and T. Whan Kim, Photocatalytic degradation mechanisms of self-assembled rose-flower-like CeO2 hierarchical nanostructures, Appl. Phys. Lett., 2013, 102, 223115 CrossRef.
- S. Yuan, Q. Zhang, B. Xu, Z. Jin, Y. Zhang, Y. Yang, M. Zhang and T. Ohno, Porous cerium dioxide hollow spheres and their photocatalytic performance, RSC Adv., 2014, 4, 62255–62261 RSC.
- N. Wetchakun, S. Chaiwichain, B. Inceesungvorn, K. Pingmuang, S. Phanichphant, A. I. Minett and J. Chen, BiVO4/CeO2 nanocomposites with high visible-light-induced photocatalytic activity, ACS Appl. Mater. Interfaces, 2012, 4, 3718–3723 CrossRef CAS PubMed.
- N. S. Arul, D. Mangalaraj, R. Ramachran, A. N. Grace and J. I. Han, Fabrication of CeO2/Fe2O3 composite nanospindles for enhanced visible light driven photocatalysts and supercapacitor electrodes, J. Mater. Chem. A, 2015, 3, 15248–15258 RSC.
- Z. Li, D. Liu, W. Huang, Y. Sun, S. Li and X. Wei, Applying facilely synthesized CuO/CeO2 photocatalyst to accelerate methylene blue degradation in hypersaline wastewater, Surf. Interface Anal., 2019, 51, 336–344 CrossRef CAS.
- J. Tian, Y. Sang, Z. Zhao, W. Zhou, D. Wang, X. Kang, H. Liu, J. Wang, S. Chen, H. Cai and H. Huang, Enhanced photocatalytic performances of CeO2/TiO2 nanobelt heterostructures, Small, 2013, 9, 3864–3872 CrossRef CAS PubMed.
- S. Ameen, M. S. Akhtar, H. K. Seo and H. S. Shin, Solution-processed CeO2/TiO2 nanocomposite as potent visible light photocatalyst for the degradation of bromophenol dye, Chem. Eng. J., 2014, 247, 193–198 CrossRef CAS.
- R. Lamba, A. Umar, S. K. Mehta and S. K. Kansal, CeO2ZnO hexagonal nanodisks: Efficient material for the degradation of direct blue 15 dye and its simulated dye bath effluent under solar light, J. Alloys Compd., 2015, 620, 67–73 CrossRef CAS.
- L. Zhu, H. Li, P. Xia, Z. Liu and D. Xiong, Hierarchical ZnO decorated with CeO2 nanoparticles as the direct Z-scheme heterojunction for enhanced photocatalytic activity, ACS Appl. Mater. Interfaces, 2018, 10, 39679–39687 CrossRef CAS PubMed.
- D. Channei, K. Chansaenpak, P. Jannoey and S. Phanichphant, The staggered heterojunction of CeO2/CdS nanocomposite for enhanced photocatalytic activity, Solid State Sci., 2019, 96, 105951 CrossRef CAS.
- S. Gu, Y. Chen, X. Yuan, H. Wang, X. Chen, Y. Liu, Q. Jiang, Z. Wu and G. Zeng, Facile synthesis of CeO2 nanoparticle sensitized CdS nanorod photocatalyst with improved visible-light photocatalytic degradation of rhodamine B, RSC Adv., 2015, 5, 79556–79564 RSC.
- X. Zhang, N. Zhang, Y. J. Xu and Z. R. Tang, One-dimensional CdS nanowires–CeO2 nanoparticles composites with boosted photocatalytic activity, New J. Chem., 2015, 39, 6756–6764 RSC.
- X. Liu, F. Meng, B. Yu and H. Wu, Self-assembly synthesis of flower-like CeO2/MoS2 heterojunction with enhancement of visible light photocatalytic activity for methyl orange, J. Mater. Sci.: Mater. Electron., 2020, 31, 6690–6697 CrossRef CAS.
- M. M. Sabzehmeidani, H. Karimi and M. Ghaedi, Visible light-induced photo-degradation of methylene blue by n–p heterojunction CeO2/CuS composite based on ribbon-like CeO2 nanofibers via electrospinning, Polyhedron, 2019, 170, 160–171 CrossRef CAS.
- X. She, H. Xu, H. Wang, J. Xia, Y. Song, J. Yan, Y. Xu, Q. Zhang, D. Du and H. Li, Controllable synthesis of CeO2/gC3N4 composites and their applications in the environment, Dalton Trans., 2015, 44, 7021–7031 RSC.
- X. Wei, X. Wang, Y. Pu, A. Liu, C. Chen, W. Zou, Y. Zheng, J. Huang, Y. Zhang, Y. Yang and M. Naushad, Facile ball-milling synthesis of CeO2/g-C3N4 Z-scheme heterojunction for synergistic adsorption and photodegradation of methylene blue: Characteristics, kinetics, models, and mechanisms, Chem. Eng. J., 2020, 127719 Search PubMed.
- A. Subashini, P. V. Prasath, S. Sagadevan, J. A. Lett, I. Fatimah, F. Mohammad, H. A. Al-Lohedan, S. F. Alshahateet and W. C. Oh, Enhanced photocatalytic degradation efficiency of graphitic carbon nitride-loaded CeO2 nanoparticles, Chem. Phys. Lett., 2021, 769, 138441 CrossRef CAS.
- W. Ming Yan, Z. Wei, Z. Dong En, L. Shu An, M. Wei Xing, T. Zhi Wei and C. Jun, CeO2 hollow nanospheres decorated reduced graphene oxide composite for efficient photocatalytic dye-degradation, Mater. Lett., 2014, 137, 229–232 CrossRef.
- R. Verma and S. K. Samdarshi,
In situ decorated optimized CeO2 on reduced graphene oxide with enhanced adsorptivity and visible light photocatalytic stability and reusability, J. Phys. Chem. C, 2016, 120, 22281–22290 CrossRef CAS.
- T. Feng, X. Wang and G. Feng, Synthesis of novel CeO2 microspheres with enhanced solar light photocatalyic properties, Mater. Lett., 2013, 100, 36–39 CrossRef CAS.
- J. W. Ko, J. H. Kim and C. B. Park, Synthesis of visible light-active CeO2 sheets via mussel-inspired CaCO3 mineralization, J. Mater. Chem. A, 2013, 1, 241–245 RSC.
- Y. C. Zhang, M. Lei, K. Huang, C. Liang, Y. J. Wang, S. S. Ding, R. Zhang, D. Y. Fan, H. J. Yang and Y. G. Wang, A facile route to mono-dispersed CeO2 nanocubes and their enhanced photocatalytic properties, Mater. Lett., 2014, 116, 46–49 CrossRef CAS.
- F. Chen, Y. Cao and D. Jia, Preparation and photocatalytic property of CeO2 lamellar, Appl. Surf. Sci., 2011, 257, 9226–9231 CrossRef CAS.
- M. Xu, S. Xie, X.-H. Lu, Z.-Q. Liu, Y. Huang, Y. Zhao, J. Ye and Y.-X. Tong, Controllable electrochemical synthesis and photocatalytic activity of CeO2 octahedra and nanotubes, J. Electrochem. Soc., 2011, 158, E41–E44 CrossRef CAS.
- M. M. Khan, S. A. Ansari, M. O. Ansari, B. Min, J. Lee and M. H. Cho, Biogenic fabrication of Au@CeO2 nanocomposite with enhanced visible light activity, J. Phys. Chem. C, 2014, 118, 9477–9484 CrossRef CAS.
- L. Huang, Y. Li, H. Xu, Y. Xu, J. Xia, K. Wang, H. Li and X. Cheng, Synthesis and characterization of CeO2/g-C3N4 composites with enhanced visible-light photocatatalytic activity, RSC Adv., 2013, 3, 22269–22279 RSC.
- S. Xing, T. Li, Y. Gao and J. Liu, Insight into the mechanism for photocatalytic degradation of ciprofloxacin with CeO2, Optik, 2019, 183, 266–272 CrossRef CAS.
- H. Wang, B. Liao, T. Lu, Y. Ai and G. Liu, Enhanced visible-light photocatalytic degradation of tetracycline by a novel hollow BiOCl@CeO2 heterostructured microspheres: Structural characterization and reaction mechanism, J. Hazard. Mater., 2020, 385, 121552 CrossRef CAS PubMed.
- J. Shen, J. Shen, W. Zhang, X. Yu, H. Tang, M. Zhang and Q. Liu, Built-in electric field induced CeO2/Ti3C2-MXene Schottky-junction for coupled photocatalytic tetracycline degradation and CO2 reduction, Ceram. Int., 2019, 45, 24146–24153 CrossRef CAS.
- Z. Ye, J. Li, M. Zhou, H. Wang, Y. Ma, P. Huo, L. Yu and Y. Yan, Well-dispersed nebula-like ZnO/CeO2@ HNTs heterostructure for efficient photocatalytic degradation of tetracycline, Chem. Eng. J., 2016, 304, 917–933 CrossRef CAS.
- X. J. Wen, C. G. Niu, L. Zhang, C. Liang and G. M. Zeng, A novel Ag2O/CeO2 heterojunction photocatalysts for photocatalytic degradation of enrofloxacin: possible degradation pathways, mineralization activity and an in depth mechanism insight, Appl. Catal., B, 2018, 221, 701–714 CrossRef CAS.
- B. Wu, C. Shan, X. Zhang, H. Zhao, S. Ma, Y. Shi, J. Yang, H. Bai and Q. Liu, CeO2/Co3O4 porous nanosheet prepared using rose petal as biotemplate for photo-catalytic degradation of organic contaminants, Appl. Surf. Sci., 2020, 543, 148677 CrossRef.
- X. Yang, Y. Zhang, Y. Wang, C. Xin, P. Zhang, D. Liu, B. B. Mamba, K. K. Kefeni, A. T. Kuvarega and J. Gui, Hollow β-Bi2O3@CeO2 heterostructure microsphere with controllable crystal phase for efficient photocatalysis, Chem. Eng. J., 2020, 387, 124100 CrossRef CAS.
- W. Liu, J. Zhou and J. Yao, Shuttle-like CeO2/g-C3N4 composite combined with persulfate for the enhanced photocatalytic degradation of norfloxacin under visible light, Ecotoxicol. Environ. Safety, 2020, 190, 110062 CrossRef CAS PubMed.
- H. Qi, C. Shi, X. Jiang, M. Teng, Z. Sun, Z. Huang, D. Pan, S. Liu and Z. Guo, Constructing CeO2/nitrogen-doped carbon quantum dot/gC3N4 heterojunction photocatalysts for highly efficient visible light photocatalysis, Nanoscale, 2020, 12, 19112–19120 RSC.
- R. Ji, Z. Zhu, W. Ma, X. Tang, Y. Liu and P. Huo, A heterojunction photocatalyst constructed by the modification of 2D-CeO2 on 2D-MoS2 nanosheets with enhanced degrading activity, Catal. Sci. Technol., 2020, 10, 788–800 RSC.
- R. Ma, S. Zhang, L. Li, P. Gu, T. Wen, A. Khan, S. Li, B. Li, S. Wang and X. Wang, Enhanced visible-light-induced photoactivity of type-II CeO2/g-C3N4 nanosheet toward organic pollutants degradation, ACS Sustainable Chem. Eng., 2019, 7, 9699–9708 CrossRef CAS.
- Q. Gao, Y. Cui, S. Wang, B. Liu and C. Liu, Enhanced photocatalytic activation of peroxymonosulfate by CeO2 incorporated ZnCo–layered double hydroxide toward organic pollutants removal, Sep. Purif. Technol., 2021, 263, 118413 CrossRef CAS.
- B. Yu, F. Meng, T. Zhou, A. Fan, Z. Zhao and M. Wasim Khan, Construction of CoS/CeO2 heterostructure nanocages with enhanced photocatalytic performance under visible light, J. Am. Ceram. Soc., 2020, 103, 6136–6148 CrossRef CAS.
- Y. Chai, L. Zhang, Q. Liu, F. Yang and W. L. Dai, Insights into the relationship of the heterojunction structure and excellent activity: Photo-oxidative coupling of benzylamine on CeO2-rod/g-C3N4 hybrid under mild reaction conditions, ACS Sustainable Chem. Eng., 2018, 6, 10526–10535 CrossRef CAS.
- A. Azimi, A. Azari, M. Rezakazemi and M. Ansarpour, Removal of heavy metals from industrial wastewaters: A review, ChemBioEng Rev., 2017, 4, 37–59 CrossRef.
- A. Ayangbenro and O. Babalola, A new strategy for heavy metal polluted environments: A review of microbial biosorbents, Int. J. Environ. Res. Publ. Health, 2017, 14, 94 CrossRef PubMed.
- C. E. Barrera-Díaz, V. Lugo-Lugo and B. Bilyeu, A review of chemical, electrochemical and biological methods for aqueous Cr(VI) reduction, J. Hazard. Mater., 2012, 223, 1–12 CrossRef PubMed.
- D. K. Padhi and K. Parida, Facile fabrication of α-FeOOH nanorod/RGO composite: A robust photocatalyst for reduction of Cr(VI) under visible light irradiation, J. Mater. Chem. A, 2014, 2, 10300–10312 RSC.
- Y. Li, Z. Liu, Y. Wu, J. Chen, J. Zhao, F. Jin and P. Na, Carbon dots–TiO2 nanosheets composites for photoreduction of Cr(VI) under sunlight illumination: Favorable role of carbon dots, Appl. Catal., B, 2018, 224, 508–517 CrossRef CAS.
- Q. Meng, Y. Zhou, G. Chen, Y. Hu, C. Lv, L. Qiang and W. Xing, Integrating both homojunction and heterojunction in QDs self-decorated Bi2MoO6/BCN composites to achieve an efficient photocatalyst for Cr(VI) reduction, Chem. Eng. J., 2018, 334, 334–343 CrossRef CAS.
- S. Peng, J. Yang, L. Guo, J. Wang, J. Zhao, J. Xu and Z. Li, Shape-dependent CeO2@ BiOI for degradation of aqueous Cr(VI), Adv. Mater. Interfaces, 2020, 7, 1901879 CrossRef CAS.
- Y. Xiao, S. Tan, D. Wang, J. Wu, T. Jia, Q. Liu, Y. Qi, X. Qi, P. He and M. Zhou, CeO2/BiOIO3 heterojunction with oxygen vacancies and Ce4+/Ce3+ redox centers synergistically enhanced photocatalytic removal heavy metal, Appl. Surf. Sci., 2020, 530, 147116 CrossRef CAS.
- J. Yang, Y. Liang, K. Li, G. Yang and S. Yin, One-step low-temperature synthesis of 0D CeO2 quantum dots/2D BiOX (X = Cl, Br) nanoplates heterojunctions for highly boosting photo-oxidation and reduction ability, Appl. Catal., B, 2019, 250, 17–30 CrossRef CAS.
- G. Yang, Y. Liang, K. Li, J. Yang, R. Xu and X. Xie, Construction of a Ce3+ doped CeO2/Bi2MoO6 heterojunction with a mutual component activation system for highly enhancing the visible-light photocatalytic activity for removal of TC or Cr(VI), Inorg. Chem. Front., 2019, 6, 1507–1517 RSC.
- H. Shen, M. Lin, L. Wang, Z. Huang, X. Wu, X. Jiang, Q. Li, C. L. Chen, J. Zhao, G. Jing and C. S. Yuan, Experimental and theoretical investigation of the enhancement of the photo-oxidation of Hg0 by CeO2-modified morphology-controlled anatase TiO2, J. Hazard. Mater., 2020, 406, 124535 CrossRef PubMed.
- S. Li, J. Cai, X. Wu, B. Liu, Q. Chen, Y. Li and F. Zheng, TiO2@ Pt@ CeO2 nanocomposite as a bifunctional catalyst for enhancing photo-reduction of Cr(VI) and photo-oxidation of benzyl alcohol, J. Hazard. Mater., 2018, 346, 52–61 CrossRef CAS PubMed.
- L. Kashinath, K. Namratha and K. Byrappa, Microwave mediated synthesis and characterization of CeO2–GO hybrid composite for removal of chromium ions and its antibacterial efficiency, J. Environ. Sci., 2019, 76, 65–79 CrossRef PubMed.
- L. Guo, J. Yang, H. Zhang, R. Wang, J. Xu and J. Wang, Highly enhanced visible-light photocatalytic activity via a novel surface structure of CeO2/g-C3N4 toward removal of 2,4-dichlorophenol and Cr(VI), ChemCatChem, 2021, 13, 2034–2044 CrossRef CAS.
- T. Jia, J. Wu, Y. Xiao, Q. Liu, Q. Wu, Y. Qi and X. Qi, Self-grown oxygen vacancies-rich CeO2/BiOBr Z-scheme heterojunction decorated with rGO as charge transfer channel for enhanced photocatalytic oxidation of elemental mercury, J. Colloid Interface Sci., 2020, 587, 402–416 CrossRef PubMed.
- J. Bai, X. Wang, G. Han, Z. Xie and G. Diao, CQDs decorated oxygen vacancy-rich CeO2/BiOCl heterojunctions for promoted visible light photoactivity towards chromium(VI) reduction and rhodamine B degradation, J. Alloys Compd., 2020, 859, 157837 CrossRef.
|
This journal is © The Royal Society of Chemistry 2021 |
Click here to see how this site uses Cookies. View our privacy policy here.