DOI:
10.1039/D1FO02579A
(Paper)
Food Funct., 2022,
13, 198-212
Diosmetin alleviated cerebral ischemia/reperfusion injury in vivo and in vitro by inhibiting oxidative stress via the SIRT1/Nrf2 signaling pathway
Received
7th August 2021
, Accepted 26th November 2021
First published on 27th November 2021
Abstract
Cerebral ischemia/reperfusion (I/R) injury is caused by blood flow recovery after an ischemic stroke, and effective treatments targeting I/R injury are still insufficient. Oxidative stress is known to play a pivotal role in the pathogenesis of cerebral I/R injury. Previous studies have revealed that diosmetin could protect against oxidative stress in cerebral I/R injury, but the underlying mechanisms have not been fully revealed. The present study was undertaken to investigate the effects and mechanisms of action of diosmetin on cerebral I/R injury. In vivo, rats were orally gavaged with diosmetin for seven days, and middle cerebral artery occlusion (MCAO) was established to simulate cerebral I/R injury. The neurological deficit score, cerebral infarct volume, and cortical pathological lesions were measured. In vitro, PC12 cells were exposed to oxygen-glucose deprivation/reoxygenation (OGD/R). To clarify the mechanism, the SIRT1 inhibitor EX527 and the small interfering RNA (siRNA) of SIRT1 were used to downregulate the SIRT1 protein level, respectively. The contents of superoxide dismutase (SOD), catalase (CAT), glutathione (GSH), and malondialdehyde (MDA) were determined with commercial kits. The protein expressions of SIRT1, total Nrf2 (T-Nrf2), nucleus Nrf2 (N-Nrf2), NQO1 and HO-1 were measured by western blotting. The results showed that diosmetin pretreatment improved neurological outcomes, decreased the cerebral infarct volume and pathological lesions, and inhibited oxidative stress in cerebral I/R rats. In PC12 cells, diosmetin increased cell viability, reduced lactate dehydrogenase (LDH) release and reactive oxygen species (ROS) level, and inhibited oxidative stress. Besides, diosmetin increased the protein expressions of SIRT1, T-Nrf2, N-Nrf2, NQO1 and HO-1 both in vivo and in vitro. However, administration of EX527 or silencing the SIRT1 gene with its siRNA eliminated the beneficial effects of diosmetin. Meanwhile, inhibition of SIRT1 decreased the levels of Nrf2 and the protein expressions of its downstream antioxidants NQO1 and HO-1. In conclusion, our data suggested that diosmetin could attenuate cerebral I/R injury by inhibiting oxidative stress via the SIRT1/Nrf2 signaling pathway.
1. Introduction
Ischemic stroke, which is caused by a transient or permanent interruption of cerebral blood flow, accounts for more than 80% of all strokes and is the most common cause of disability, mortality and morbidity worldwide.1 The primary therapeutic strategy for acute ischemic stroke is the restoration of cerebral blood flow. At present, recombinant tissue plasminogen activator (rt-PA) is the only approved pharmacotherapy for acute ischemic stroke.2 However, the application of rt-PA is limited due to the narrow therapeutic window, risk of hemorrhage and grievous secondary side effects.3 Moreover, recanalization of blood flow could further aggravate the nerve injury and neurological dysfunction caused by ischemia, which is known as cerebral ischemia/reperfusion (I/R) injury. Hence, more efforts should be devoted to developing novel agents for treating cerebral I/R injury and associated neurological injuries.
Abundant evidence shows that oxidative stress plays a pivotal role in the pathogenesis of cerebral I/R injury, which is caused by the overproduction of ROS and decreased activity of scavenger enzymes and protective antioxidants.4 The increase in ROS and intracellular oxidizing substances dramatically leads to oxidative damage of proteins, lipids, and nucleic acids, and subsequently results in cell and tissue dysfunction, and cell death.5 Additionally, cerebral I/R-induced production of ROS could also increase hemorrhagic infarction and brain edema, and disrupt the blood–brain barrier.4 Thus, improving the expression of the endogenous antioxidative proteins and reducing oxidative stress may be effective approaches for alleviating cerebral I/R injury. Nuclear factor erythroid 2-related factor 2 (Nrf2), a basic leucine zipper (bZIP) protein, is a crucial transcription factor that maintains cellular redox homeostasis. Under normal conditions, Nrf2 is retained by Kelch-like ECH-associated protein 1 (Keap1) in the cytoplasm. When oxidative stress occurs, Nrf2 translocates into the nucleus to bind to antioxidant response element (ARE), and then promotes the transcription of antioxidant enzymes and phase II detoxifying enzymes, including NAD(P)H, quinone oxidoreductase-1 (NQO1), heme oxygenase-1 (HO-1), superoxide dismutase (SOD), and catalase (CAT). Previous studies revealed that the activation of the Nrf2 pathway confers protection against cerebral I/R injury.6,7 In addition, the activation of Nrf2 is under the control of numerous regulators, one of which is silent information regulator 1 (SIRT1), a nicotinamide adenine dinucleotide (NAD)-dependent deacetylase that is involved in many physiological and pathological processes after cerebral I/R, such as oxidative stress, inflammation and apoptosis.8 The neuroprotective properties of SIRT1 have been demonstrated in cerebral ischemia and reperfusion,9 and accumulating studies have proved that SIRT1 plays a key role in regulating Nrf2 expression.10,11
Diosmetin, a bioflavonoid that is naturally abundant in legumes, olive leaves, citrus fruits, and Menthae Haplocalycis herba,12 has been found to possess a wide range of pharmacological activities, such as anti-oxidation,13 anti-inflammation,14 anti-apoptosis15 and anti-cancer activities.16 Accumulating evidence has demonstrated that diosmetin possesses a significant antioxidant capacity by preventing the generation of intracellular ROS, inhibiting the formation of MDA, and regulating intracellular antioxidant enzyme activities.17,18 Diosmetin has been found to exert protective effects against renal I/R injury by enhancing antioxidant capabilities via activating the Nrf2 pathway.19 Luan et al. reported that diosmetin effectively protected PC12 cells from oxygen-glucose deprivation/reoxygenation (OGD/R)-induced injury by regulating ROS via the Nrf2 pathway.18 Based on the research studies mentioned above, we hypothesized that diosmetin may be promising as a therapeutic agent and dietary supplement by inhibiting oxidative stress via activating the SIRT1/Nrf2 signaling pathway. In the present study, we established an animal model of cerebral I/R and an in vivo cell model of OGD/R to explore the protective effects of diosmetin. The influence of diosmetin on oxidative stress and the SIRT1/Nrf2 signaling pathway was investigated.
2. Materials and methods
2.1 Experimental animals
Male Sprague-Dawley rats (weight 200–240 g) were purchased from the Yichang Animal Center of China Three Gorges University (license No. SCXK (E) 2017-0012). All rats were kept in a well-ventilated animal house (22 ± 3 °C, 60 ± 5% humidity) under a 12 h light–dark cycle with free access to water and food. After one week of adaptive feeding, the rats were randomly divided into five groups with twelve rats in each group: sham group, I/R group, I/R + diosmetin (40 mg kg−1) group, I/R + diosmetin (80 mg kg−1) group, and I/R + diosmetin (80 mg kg−1) + EX527 group. The doses were selected in accordance with the preliminary experiment. This study was performed according to the National Institute of Health Guide for the Care and Use of Laboratory Animals. The experiments were approved by the Laboratory Animal Ethical Committee of China Three Gorges University (No. 2019070I).
2.2 Drug preparation and treatment schedule
Diosmetin (C16H12O6, molecular weight = 300.26, purity >98%, verified by high-performance liquid chromatography) was obtained from Chengdu Alfa Biotechnology Co., Ltd, China. Diosmetin powder was dissolved in saline with dimethyl sulfoxide (DMSO) to prepare different doses of peroral diosmetin solution (40 and 80 mg kg−1). The rats in the I/R + diosmetin (40 and 80 mg kg−1) groups were orally gavaged with the corresponding drug solution once a day for 7 days before surgery. The sham and I/R group rats received the same amount of physiological saline. The I/R + diosmetin (80 mg kg−1) + EX527 group rats were administered with diosmetin at a dose of 80 mg kg−1 once daily for 7 days and intraperitoneally injected with the SIRT1 inhibitor EX527 at a dose of 5 mg kg−1 every 2 days before surgery. The chemical structure of diosmetin and the schematic plan of work are shown in Fig. 1.
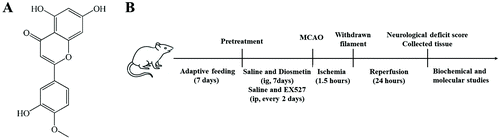 |
| Fig. 1 The chemical structure of diosmetin and the schematic plan of work. | |
2.3 Establishment of the cerebral I/R injury model
A cerebral I/R model was accomplished by middle cerebral artery occlusion (MCAO) as previously described.20 After 1 h of the last administration, the rats were anesthetized with 10% chloral hydrate (350 mg kg−1) by intraperitoneal injection. Then the rats were fixed on an operating board in the supine position. After iodophor disinfection, a 2 cm median incision was made on the neck to expose the right common carotid artery (CCA), external carotid artery (ECA), and internal carotid artery (ICA). A small cut was made at the proximal end of the CCA using eye scissors, and a nylon monofilament (Beijing Cinontech Co. Ltd, China) with a diameter of 0.24 mm was carefully inserted from the incision into the ICA until it blocked the origin of the middle cerebral artery (MCA). After 90 min of occlusion, the monofilament was gently removed to restore blood flow and allow 24 h reperfusion. The body temperature of the rats was maintained at 37 °C using a warming pad during the entire surgical procedure. Rats in the sham group were subjected to the same surgical procedure except for occlusion/reperfusion.
2.4 Evaluation of neurological deficit
The neurological deficit score (n = 12 for each group) was assessed by an investigator blinded to the study protocol by following a previously described method.20 The specific scoring method was: 0, no neurological deficit (normal walk); 1, a mild focal neurological deficit (failure to extend the opposite forepaw fully); 2, a moderate focal neurological deficit (circling to the contralateral side); 3, a severe focal neurological deficit (falling to the contralateral side); and 4, loss of consciousness and spontaneous motor activity.
2.5 Assessments of cerebral infarct volume
The cerebral infarct size was evaluated by 2,3,5-triphenyltetrazolium chloride (TTC, Sigma-Aldrich, St Louis, MO, USA) staining. Briefly, at 24 h after reperfusion, brain samples were harvested and maintained at −20 °C for 30 min. Then the brain samples were sectioned into five slices at a thickness of 2 mm. The brain sections were immersed in 0.2% solution of TTC at 37 °C for 30 min and fixed in 4% paraformaldehyde solution for 24 h. Pictures were taken and the infarct size was calculated using ImageJ software (Image J, Version 1.8.0, National Institutes of Health, Bethesda, MD, USA). The percentage infarct volume was calculated as (infarct area/area of whole section) × 100%.
2.6 Hematoxylin–eosin (HE) and Nissl staining
Rats were anesthetized with chloral hydrate and perfused with 200 ml normal saline, followed by 4% paraformaldehyde. Brain tissues were removed quickly and fixed in 4% paraformaldehyde for 24 h. Following this, the brain tissues were embedded in paraffin and cut into 4-μm-thick sections. Subsequently, the sections were stained with HE and Nissl for observation under a light microscope (Olympus BX51; Olympus Co., Tokyo, Japan).
2.7 Cell culture
Rat PC12 cells, neuron-like cells, were purchased from Procell Life Science & Technology Co., Ltd (Wuhan, China). The cells were cultured in RPIM-1640 medium containing 10% fetal bovine serum (FBS) at 37 °C under a humidified atmosphere of 5% CO2 and 95% air.
2.8 OGD/R injury and drug treatment
For the establishment of the OGD/R model, the normal medium was removed and the PC12 cells were washed three times with glucose-free DMEM. Then the cells were incubated for 2 h under a humidified atmosphere of 93% N2, 5% CO2, and 2% O2. Following the OGD treatment, the cells were subsequently returned to an incubator under normoxic conditions with or without different concentrations of diosmetin (final concentrations: 5, 10, 20, 40, 80, and 160 nM) for 24 h. Diosmetin was added to the culture 2 h prior to OGD insult and throughout the reoxygenation phase.
2.9 Cell transfection
To achieve SIRT1 gene silencing, small interfering RNA (siRNA) was designed and synthesized by GenePharma Co., Ltd (Wuhan. China). Sequences of siRNA-SIRT1 were as follows: sense 5′-CCAGUAGCACUAAUUCCAATT-3′, antisense 5′-UUGGAAUUAGUGCUACUGGTT-3′. PC12 cells were seeded in 6 wells for 24 h and transfected with SIRT1 or negative control siRNA duplexes using Lipofectamine 2000 reagent (Invitrogen, USA) according to the manufacturer's instructions.
2.10 Assessment of cell viability
The MTT assay was used to assess the cell viability of PC12 cells and to screen two suitable concentrations of diosmetin for subsequent experiments. The cells were divided into ten groups: control group, OGD/R group, OGD/R + DMSO group, diosmetin-treated groups (OGD/R + diosmetin 5, 10, 20, 40, 80, and 160 nM), and siRNA-negative control (siRNA-NC) group, and cultivated in 96-well microplates at 1 × 105 cells per well for 24 h. Then MTT (0.5 mg ml−1, 10 μL per hole) was added into each well under sterile conditions and incubated for 4 h at 37 °C. After that, DMSO solution (150 μL per hole) was added into each well to dissolve colored formazan. The plate was shaken for 10 min until the blue crystal products formed were dissolved completely, and the absorbance was measured at 568 nm. Cell viability was expressed as a percent of the control culture value.
2.11 Lactate dehydrogenase (LDH) release
LDH is a stable cytoplasmic enzyme present in most cells, and it is rapidly released into the cell culture supernatant when the plasma membrane is damaged. In the present study, cytotoxicity was quantitatively assessed by measuring the LDH leakage in culture medium by using a commercial kit (Nanjing Jiancheng Bioengineering, Jiangsu, China) according to the manufacturer's instructions. The medium which contained the detached cells was collected and centrifuged. The supernatant was used for the assay of LDH release. The absorbance was monitored on a microplate reader at 440 nm.
2.12 Detection of ROS accumulation
A 2′,7′-dichlorodihydrofluorescein diacetate (DCFH-DA) fluorescent probe from a Reactive Oxygen Species Assay Kit (Beyotime Biotech) was used to detect ROS generation according to the manufacturer's instructions. DCFH-DA penetrates the cell membrane freely and is hydrolyzed by esterase into DCFH, which cannot escape from the cell, and then ROS further oxidizes DCFH to DCF, which fluoresces bright green. PC12 cells were harvested and incubated in DCFH-DA (50 μM) and maintained at 37 °C for 60 min. Subsequently, the fluorescence intensity was measured using a fluorescence microscope at excitation and emission wavelengths of 488 nm and 525 nm respectively. The fluorescence intensity reflected the amount of ROS generated.
2.13 Determination of oxidative stress
The right cortex tissue and PC12 cell homogenates (10%) of rats were prepared by homogenizing the sample in physiological saline at a ratio of 1
:
9 using a mixer. SOD activity, CAT activity, glutathione (GSH) content, and malondialdehyde (MDA) content were determined with the corresponding assay kits (Nanjing Jiancheng Bioengineering Institute, Nanjing, China), respectively. SOD activity was measured using the water-soluble tetrazolium salt (WST-1) method. CAT activity was measured using the ammonium molybdate method. The GSH content was measured using the DTNB method. The MDA content was measured by the thiobarbituric acid (TBA) method.
2.14 Western blotting analysis
Brain cortex tissues and PC12 cells were homogenized in RIPA lysis buffer (Beyotime Biotechnology, Shanghai, China), centrifuged at 12
000 rpm at 4 °C for 10 min, and the supernatants were collected. Nuclear protein was extracted by using the nuclear and cytoplasmic protein extraction kit (Nanjing KeyGen Biotech Co., Ltd, Nanjing, China) according to the manufacturer's recommendations. Protein concentrations were measured using a BCA protein assay kit (Beyotime Biotechnology, Shanghai, China). An equivalent amount of protein (20 μg) was loaded and separated using 12% SDS-PAGE. After electrophoresis, the protein was transferred to a polyvinylidene difluoride (PVDF) membrane. After blocking with 5% skimmed milk powder for 1 h, the proteins were hybridized with the primary antibodies SIRT1 (1
:
1000, Affinity, USA), Nrf2 (1
:
1000, Absin, China), NQO1 (1
:
1000, Servicebio, China), HO-1 (1
:
1000, Proteintech, China), β-actin (1
:
10
000, Absin, China), GAPDH (1
:
1000, Xianzhi Bio, Hangzhou, China), and Lamin B (1
:
1000, BOSTER, China) overnight at 4 °C. Subsequently, the membrane was washed three times with TBST buffer and incubated with IgG secondary antibody for 2 h at room temperature. After washing with TBST, the antibody-bound proteins were detected with the ECL reagent. The cytosolic proteins (SIRT1, T-Nrf2, NQO1, and HO-1) and the nuclear protein (N-Nrf2) in vivo were normalized to β-actin and Histone H3, respectively, and the cytosolic proteins (SIRT1, T-Nrf2, NQO1, and HO-1) and the nuclear protein (N-Nrf2) in vitro were normalized to GAPDH and Lamin B, respectively, to obtain the final result expressed as the intensity ratio. The densitometry was analyzed using BandScan software 4.30.
2.15 Statistical analysis
All the experimental data were analyzed using SPSS 20.0 statistical software (IBM Corp., Armonk, NY, USA), and graphs were generated using GraphPad Prism 6.0 (GraphPad Software). Data were expressed as mean ± standard error of the mean (SEM). Multigroup comparisons were performed using one-way analysis of variance (ANOVA) and the post hoc least significant difference (LSD) test. A value of P < 0.05 was considered statistically significant.
3. Results
3.1 Diosmetin pretreatment improved neurological function and decreased cerebral infarct volume in cerebral I/R injury rats
To investigate the effect of diosmetin on cerebral I/R outcomes, the neurological function and cerebral infarct volume were determined. As shown in Fig. 2B, the rats in the sham group did not exhibit any signs of neurological behavioral dysfunction and the neurological deficit scores were significantly increased in the I/R group compared to the sham group. The scores of the groups treated with diosmetin at 40 mg kg−1 and 80 mg kg−1 were obviously lower than that of the I/R group (P < 0.05 and P < 0.01 respectively), and significantly higher than that of the sham group (P < 0.01). However, EX527 eliminated the decreased neurological scores of diosmetin pretreatment. As shown in Fig. 2A and C, TTC staining confirmed that the infarct volume was significantly reduced in the diosmetin-treated I/R group (40 mg kg−1 and 80 mg kg−1) in a dose-dependent manner compared with the I/R group (P < 0.05), and there was no difference between the diosmetin-treated I/R group (80 mg kg−1) and the sham group. However, EX527 blocked the reduction of cerebral infarction caused by diosmetin treatment (P < 0.05).
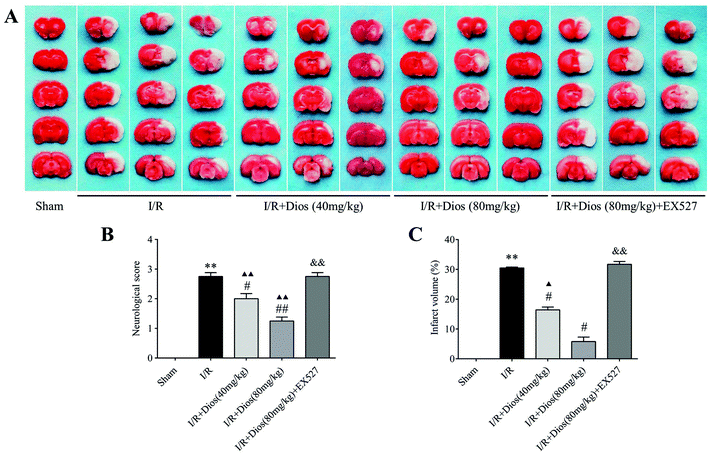 |
| Fig. 2 Effects of diosmetin on the cerebral infarct volume and neurological deficit following MCAO in rats. (A) Photographs of TTC-stained brain slices. (B) Percentage analysis of the cerebral infarct volume; values are expressed as mean ± SEM (n = 3). (C) Neurological deficit scores analysis; values are expressed as mean ± SEM (n = 12). **P < 0.01 vs. sham group. #P < 0.05 vs. I/R group. ##P < 0.01 vs. I/R group. ▲P < 0.05 vs. sham group. ▲▲P < 0.01 vs. sham group. &&P < 0.01 vs. I/R + diosmetin (80 mg kg−1) group. | |
3.2 Diosmetin pretreatment alleviated pathological lesions in the cortex of cerebral I/R rats
To further confirm the neuroprotective effects of diosmetin, sections were stained with HE and Nissl to evaluate the morphological alterations. As shown in Fig. 3A, HE staining showed that the tissues were normal, and no obvious vacuolated spaces were observed in the sham group. Cells were densely arranged with normal morphology, and the nucleolus was clearly visible. However, tissues and cells were severely damaged in the I/R group. Numerous vacuolated spaces were presented. The cells became sparse and disorderly arranged, and the nucleolus was pyknotic and dyed deeply. Compared with the I/R group, pretreatment with diosmetin relieved the pathological lesions. As shown in Fig. 3B, Nissl staining showed round nuclei and integral Nissl bodies in the sham group, whereas cerebral I/R resulted in severe loss of Nissl bodies compared with the sham group (P < 0.01). Diosmetin significantly improved the number of Nissl bodies and the neuron structure in a dose dependent manner compared with the I/R group (P < 0.01), and there was no difference between the diosmetin-treated I/R group (80 mg kg−1) and the sham group. However, EX527 blocked the protective effects of diosmetin on cortical tissues (Fig. 3C). The results revealed that diosmetin could relieve cell damage caused by cerebral I/R injury.
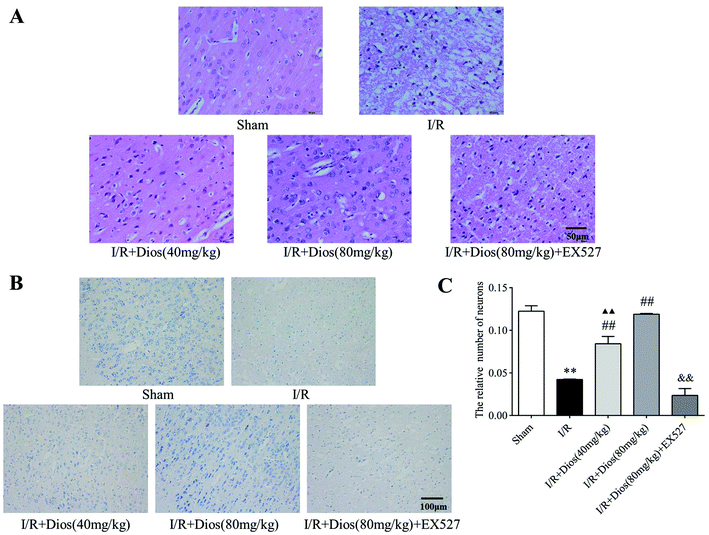 |
| Fig. 3 Effects of diosmetin on pathological lesions. (A) Representative photographs of HE-stained brain sections (400×) (scale bar = 50 μm). (B) Representative photographs of Nissl-stained brain sections (200×) (scale bar = 100 μm). (C) Nissl body number analysis. Values are expressed as mean ± SEM (n = 3). **P < 0.01 vs. sham group. ##P < 0.01 vs. I/R group. ▲▲P < 0.01 vs. sham group. &&P < 0.01 vs. I/R + diosmetin (80 mg kg−1) group. | |
3.3 Diosmetin pretreatment attenuated oxidative stress in cerebral I/R rats
To examine whether the neuroprotective effects of diosmetin are associated with its antioxidative activity, SOD and CAT activity and GSH and MDA content in the cortical brain tissues of cerebral I/R rats were determined. As shown in Fig. 4, a significant decrease in SOD and CAT activity and the GSH content, and a marked increase in the MDA content were observed in I/R-treated rats compared with the sham group (P < 0.01). However, the SOD and CAT activity and the GSH content were markedly increased upon pretreatment with diosmetin at 40 mg kg−1 and 80 mg kg−1 (P < 0.05 and P < 0.01 respectively) compared with the I/R group, and there was no difference between the diosmetin-treated I/R group (80 mg kg−1) and the sham group. Additionally, relative to cerebral I/R rats, the administration of diosmetin at 40 mg kg−1 and 80 mg kg−1 also attenuated the increase in the MDA level in cortical brain tissues (P < 0.05 and P < 0.01, respectively), and there was no statistically significant difference between the I/R + diosmetin (80 mg kg−1) group and the sham group, while EX527 considerably reversed the activity of SOD, CAT, GSH, and MDA compared with the diosmetin group (80 mg kg−1, P < 0.01). Collectively, these results showed that diosmetin improved antioxidant defenses and suppressed oxidative stress in a dose-dependent manner in cerebral I/R rats.
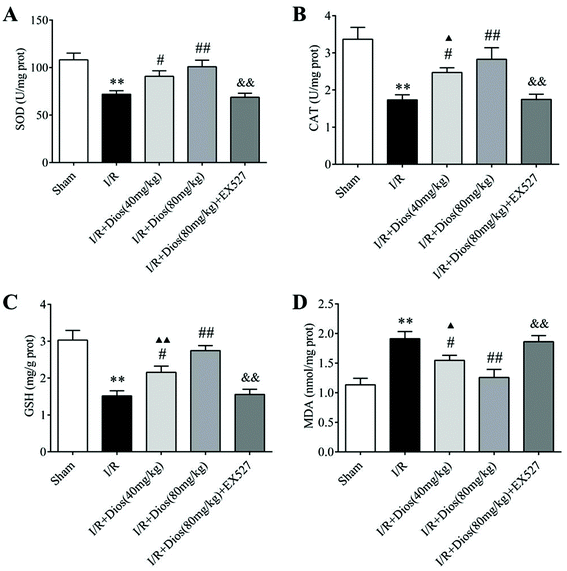 |
| Fig. 4 Effects of diosmetin on I/R-induced oxidative stress. (A) SOD activity. (B) CAT activity. (C) GSH content. (D) MDA content. Values are expressed as mean ± SEM (n = 3). **P < 0.01 vs. sham group. #P < 0.05 vs. I/R group. ##P < 0.01 vs. I/R group. ▲P < 0.05 vs. sham group. ▲▲P < 0.01 vs. sham group. &P < 0.05 vs. I/R + diosmetin (80 mg kg−1) group. &&P < 0.01 vs. I/R + diosmetin (80 mg kg−1) group. | |
3.4 Diosmetin pretreatment promoted the activation of the SIRT1/Nrf2 signaling pathway in cerebral I/R rats
To determine whether the SIRT1/Nrf2 signaling pathway plays an essential role in cerebral I/R-induced oxidative injury, the preliminary cellular location of Nrf2 and the protein expression levels of SIRT1, Nrf2, NQO1 and HO-1 were evaluated. As shown in Fig. 5, cerebral I/R significantly reduced the protein expressions of SIRT1, T-Nrf2, N-Nrf2, NQO1 and HO-1 compared with the sham group (P < 0.01). However, pretreatment with diosmetin at 40 mg kg−1 and 80 mg kg−1 both reversed the inhibition of SIRT1, T-Nrf2, N-Nrf2, NQO1 and HO-1 induced by cerebral I/R in a dose-dependent manner compared to the I/R group (P < 0.05, P < 0.01). Meanwhile, the elevated expressions of SIRT1, T-Nrf2, N-Nrf2, NQO1 and HO-1 induced by diosmetin were inhibited by EX527 (P < 0.01). Collectively, these results demonstrated that diosmetin showed protective effects against cerebral I/R injury by activating the SIRT1/Nrf2 signaling pathway, promoting Nrf2 nuclear translocation, and upregulating the protein expressions of its downstream targets NQO1 and HO-1.
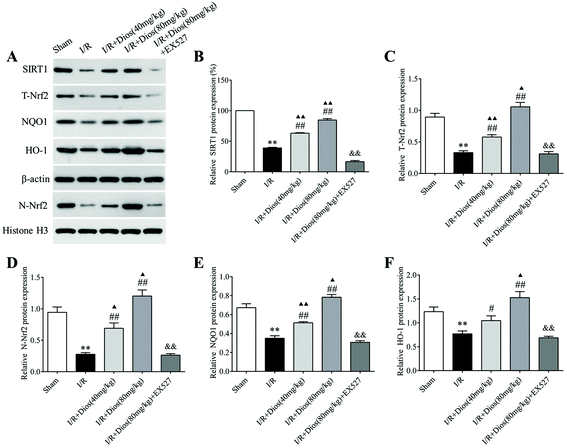 |
| Fig. 5 Effects of diosmetin on the SIRT1/Nrf2 signaling pathway. (A) Representative western blot bands of SIRT1, T-Nrf2, N-Nrf2, NQO1 and HO-1. (B–F) Relative protein expression of SIRT1, T-Nrf2, N-Nrf2, NQO1 and HO-1, respectively. Values are expressed as mean ± SEM (n = 3). **P < 0.01 vs. sham group. #P < 0.05 vs. I/R group. ##P < 0.01 vs. I/R group. ▲P < 0.05 vs. sham group. ▲▲P < 0.01 vs. sham group. &&P < 0.01 vs. I/R + diosmetin (80 mg kg−1) group. | |
3.5 Diosmetin reduced OGD/R-induced PC12 cell injury
The effects of diosmetin on OGD/R-induced PC12 cell injury were detected through morphological observation, the MTT assay which reveals cell viability, and the LDH assay which detects damaged cells. PC12 cells exposed to OGD for 2 h and reoxygenation for 24 h exhibited a marked decrease in the cell number, with most cells losing their neurites and showing a round shape. However, cellular morphology appeared much better in the groups pretreated with diosmetin (20–160 nM) (Fig. 6A), which indicated the protective effect of diosmetin against OGD/R.
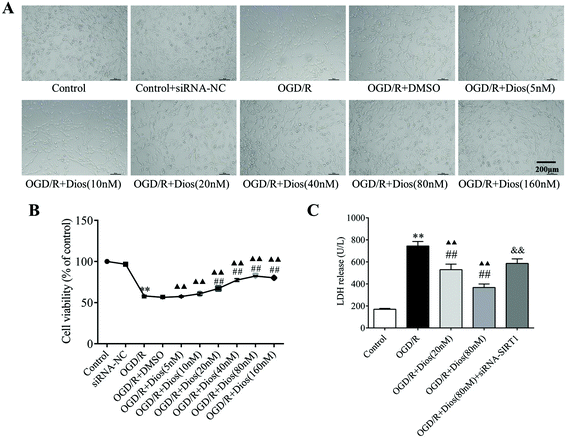 |
| Fig. 6 Effects of diosmetin on OGD/R-induced PC12 cell injury. (A) Morphology of PC12 cells. (B) Cell viability analysis. (C) LDH release analysis. Values are expressed as mean ± SEM (n = 3). **P < 0.01 vs. control group. ##P < 0.01 vs. ODG/R group. ▲▲P < 0.01 vs. control group. &&P < 0.01 vs. OGD/R + diosmetin (80 nM) group. | |
To identify the appropriate drug concentrations, OGD/R-induced PC12 cells were treated with different concentrations of diosmetin (5, 10, 20, 40, 80, and 160 nM). As shown in Fig. 6B, cell viability showed a significant decrease in the OGD/R group compared with the control group (P < 0.01), while treatment with diosmetin (20–160 nM) markedly increased cell viability compared with the OGD/R group (P < 0.01), and the cell viability was highest in the OGD/R + diosmetin (80 nM) group. Therefore, these results suggested that diosmetin conferred protective effects on PC12 cells after OGD/R, and doses of 20 nM and 80 nM were identified as the optimal drug doses for further experiments.
As shown in Fig. 6C, LDH release was significantly increased in the supernatants of PC12 cells in the OGD/R group compared with the control group (P < 0.01), which was indicative of cellular membrane damage caused by OGD/R. Compared with the OGD/R group, LDH release was significantly reduced in an obvious dose-dependent manner in the supernatants of those groups in which PC12 cells were treated with different concentrations of diosmetin (20 and 80 nM) (P < 0.01), while LDH release was significantly upregulated in the OGD/R + diosmetin (20 and 80 nM) group compared with the control group (P < 0.01). Additionally, siRNA-SIRT1 transfection reversed this reduction compared with the OGD/R + diosmetin (80 nM) group (P < 0.01). These results showed that diosmetin could attenuate cellular injury mediated by OGD/R in a dose-dependent manner, and the neuroprotective effects of diosmetin were associated with SIRT1.
3.6 Diosmetin inhibited the production of ROS in OGD/R-induced PC12 cells
To confirm that diosmetin contributes to the inhibition of ROS in OGD/R-induced PC12 cells, the production of ROS in PC12 cells was monitored by the fluorescent molecule DCFH-DA. As shown in Fig. 7, the ROS level was significantly increased in PC12 cells subjected to OGD/R compared with the control group (P < 0.01). However, the ROS levels were dose-dependently decreased after treatment with 20 nM and 80 nM diosmetin compared with the OGD/R group (P < 0.01). The level of ROS was markedly higher in the OGD/R + diosmetin group (20 and 80 nM) compared to the control group (P < 0.01). To confirm the role of SIRT1 in the regulation of ROS, cells were treated with siRNA-SIRT1, and the results showed that the ROS level was markedly increased in siRNA-SIRT1 treated cells compared with diosmetin (80 nM) treated cells (P < 0.01). These data indicated that diosmetin could dose-dependently inhibit the production of ROS in OGD/R-induced PC12 cells, which was partially mediated by SIRT1.
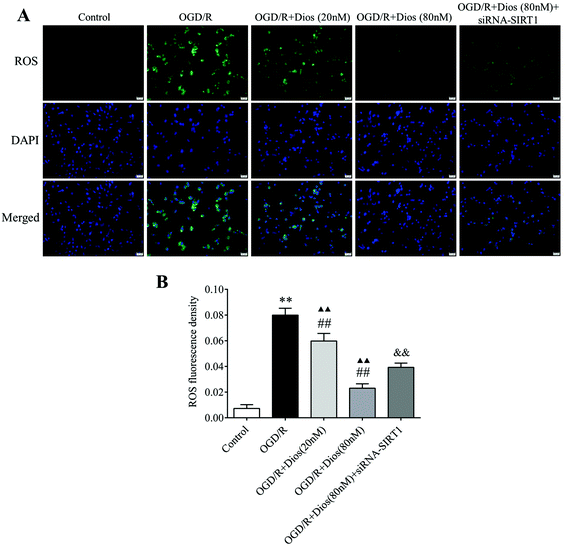 |
| Fig. 7 Effect of diosmetin on the ROS level. (A) Representative immunofluorescence photographs of ROS (scale bar = 20 μm). (B) ROS level analysis. Values are expressed as mean ± SEM (n = 3). **P < 0.01 vs. control group. ##P < 0.01 vs. OGD/R group. ▲▲P < 0.01 vs. control group. &&P < 0.01 vs. OGD/R + diosmetin (80 nM) group. | |
3.7 Diosmetin attenuated oxidative stress in OGD/R-induced PC12 cells
To elucidate the antioxidative capacity of diosmetin on OGD/R-induced PC12 cells, the activity of SOD and CAT and the content of GSH and MDA were measured. As shown in Fig. 8, remarkable decreases in the SOD and CAT activity and GSH content were observed in the OGD/R group, and the level of MDA was significantly increased in the OGD/R group compared to the control group (P < 0.01). However, pretreatment with 20 nM and 80 nM of diosmetin could predominantly increase the antioxidative capacity of PC12 cells by restoring the SOD, CAT and GSH activity and decreasing the MDA content compared to the OGD/R group (P < 0.05, P < 0.01), and there was no difference in the activity of SOD between the ODG/R + diosmetin group (20 and 80 nM) and the control group. SIRT1 silencing reversed the protective effect of diosmetin on the SOD, CAT, GSH, and MDA levels compared with that in the OGD/R + diosmetin (80 nM) group (P < 0.01). These findings indicated that diosmetin possessed antioxidative capacity in OGD/R, which was mediated via SIRT1.
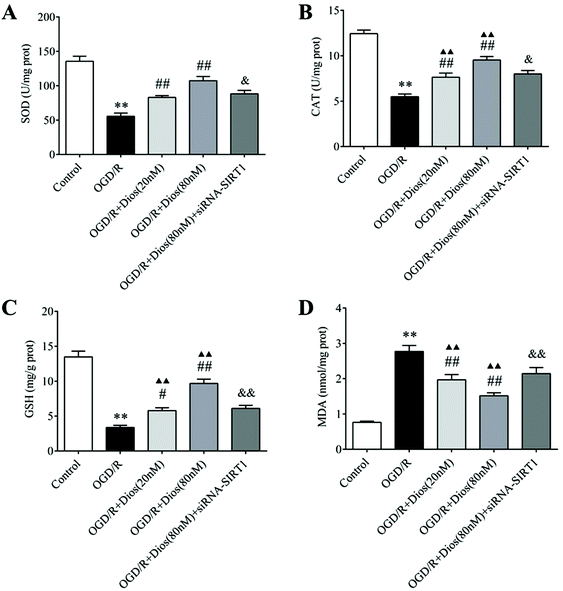 |
| Fig. 8 Effects of diosmetin on OGD/R-induced oxidative stress. (A) SOD activity. (B) CAT activity. (C) GSH content. (D) MDA content. Values are expressed as mean ± SEM (n = 3). **P < 0.01 vs. control group. #P < 0.05 vs. OGD/R group. ##P < 0.01 vs. OGD/R group. ▲▲P < 0.01 vs. control group. &P < 0.05 vs. OGD/R + diosmetin (80 nM) group. &&P < 0.01 vs. OGD/R + diosmetin (80 nM) group. | |
3.8 Diosmetin promoted SIRT1/Nrf2 signaling pathway activation in OGD/R-induced PC12 cells
To further elucidate the mechanisms of protection of PC12 cells by diosmetin against OGD/R, the SIRT1/Nrf2 signaling pathway was investigated. As shown in Fig. 9, western blot analysis showed that the protein expressions of SIRT1, T-Nrf2 and N-Nrf2 and its downstream targets NQO1 and HO-1 were significantly reduced in the OGD/R group compared with the control group (P < 0.01). However, diosmetin administration markedly enhanced the protein expressions of SIRT1, T-Nrf2, and N-Nrf2, as well as the downstream targets NQO1 and HO-1 in OGD/R-induced PC12 cells in a dose-dependent manner (P < 0.01). There was no difference in the expression of T-Nrf2, N-Nrf2, NQO1, and HO-1 between the OGD/R + diosmetin (80 nM) group and the control group. When the cells were pretreated with diosmetin (20 nM), no difference exists in the expression of T-Nrf2, NQO1, and HO-1 compared to the control group as well. Furthermore, the elevated expressions of SIRT1, T-Nrf2, N-Nrf2, NQO1, and HO-1 induced by diosmetin were inhibited by siRNA-SIRT1 administration (P < 0.01). These data demonstrated that diosmetin exerts protective effects by activating the SIRT1/Nrf2 signaling pathway, promoting Nrf2 translocation from the cytoplasm to the nucleus, and enhancing the downstream proteins NQO1 and HO-1.
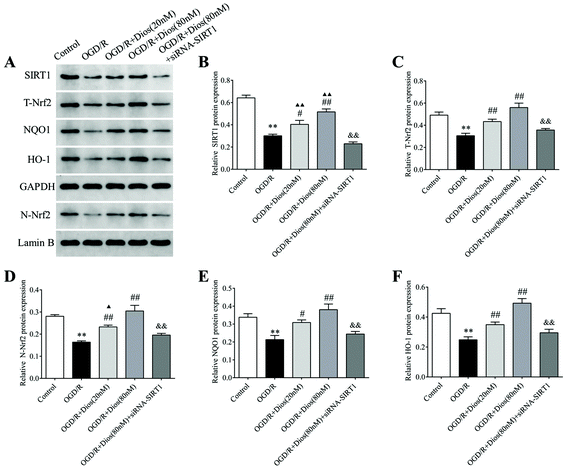 |
| Fig. 9 Effects of diosmetin on the SIRT1/Nrf2 signaling pathway. (A) Representative western blot bands of SIRT1, T-Nrf2, N-Nrf2, NQO1 and HO-1. (B–F) Relative protein expression of SIRT1, T-Nrf2, N-Nrf2, NQO1 and HO-1, respectively. Values are expressed as mean ± SEM (n = 3). **P < 0.01 vs. control group. ##P < 0.01 vs. OGD/R group. ▲P < 0.05 vs. control group. ▲▲P < 0.01 vs. control group. &P < 0.05 vs. OGD/R + diosmetin (80 nM) group. &&P < 0.01 vs. OGD/R + diosmetin (80 nM) group. | |
4. Discussion
With the increase in the aging population and the prevalence of unhealthy lifestyles, the incidence of ischemic stroke has increased, which imposes an enormous economic burden on society. At present, re-establishing blood circulation is the best strategy for ischemic stroke, but it can lead to I/R injury. In the present study, MCAO and OGD/R were used respectively as in vivo and in vitro models to mimic cerebral I/R. The neuroprotective effects as well as the underlying mechanisms of diosmetin were explored. We found that diosmetin could improve neurobehavioral function and reduce the cerebral infarct volume and cortical pathological lesions. Besides, diosmetin could also promote PC12 cell survival, increase cell viability, and reduce LDH release and ROS level. Furthermore, diosmetin also increased the SOD and CAT activity and GSH content and decreased the MDA level both in vivo and in vitro. Additionally, diosmetin upregulated the expressions of SIRT1, T-Nrf2 and N-Nrf2, promoted Nrf2 translocation into the nucleus, and increased the protein expressions of its downstream anti-oxidases NQO1 and HO-1. In contrast, all the beneficial effects of diosmetin were eliminated by administering the SIRT1 specific inhibitor EX527 or siRNA-SIRT1. These results provided convincing evidence that diosmetin could attenuate cerebral I/R injury by mitigating oxidative stress, which might be related to the activation of the SIRT1/Nrf2 signaling pathway.
Cerebral I/R injury is a complex pathological process with multiple mechanisms, and accumulating evidence suggests that oxidative stress induced by the overproduction of ROS is a crucial mechanism of cerebral I/R injury.21 The enormous generation of ROS following I/R could disrupt the balance between oxidative and antioxidative defense systems, and brain cells are susceptible to the damage induced by ROS. Importantly, oxidative stress could also induce cell apoptosis and inflammation through a series of pathways, such as the mitochondrial apoptosis pathway,22 endoplasmic reticulum stress pathway,23 and NF-κB pathway,24,25 resulting in more severe brain damage after cerebral I/R. Thus, enhancing the endogenous antioxidation by increasing antioxidant expression can elicit a certain neuroprotective effect. In the present study, the DCFH-DA method was used to detect the level of intracellular ROS in vitro. We found that diosmetin treatment perceptibly reversed the OGD/R-induced increase of ROS. Additionally, SOD, CAT, and GSH are important parts of antioxidant defense systems.26 SOD is an endogenous antioxidant enzyme, which detoxifies superoxide radicals to hydrogen peroxide specifically and protects organisms from oxidative damage. CAT plays a key role in capturing biological free radicals and catalyzing the decomposition of hydrogen peroxide into water and oxygen. GSH, as the main antioxidant, plays an irreplaceable role in maintaining redox homeostasis in cellular self-regulation. MDA is the final decomposition product of lipid peroxide oxidation, and its level is a measure for the extent of lipid peroxidation in the cytomembrane. Thus, the activity of SOD and CAT and the content of GSH and MDA could reflect the severity of oxidative stress.26,27 In our study, we found that the activity of SOD and CAT and the level of GSH were decreased, and the MDA level was increased both in rats subjected to MCAO/reperfusion and in PC12 cells exposed to OGD/R, which was dramatically reversed by diosmetin administration in a dose dependent manner. These results proved that the neuroprotective effects of diosmetin in cerebral I/R injury may be associated with its ability to alleviate oxidative stress.
Considerable interest has been focused on natural compounds such as polyphenols and bioflavonoids because of their extensive pharmacological effects against I/R injury.28,29 Polyphenols are reported to possess anti-inflammatory properties in experimental liver I/R injury, as shown by the downregulation of the main effector of inflammatory response, nuclear factor-κB.29 Bioflavonoids have also been found to show pleiotropic activities, including anti-thrombosis, anti-inflammation, and anti-oxidative effects in I/R injury.19,28 As a bioflavonoid that widely exists in citrus fruits, diosmetin has been reported to possess anti-oxidative, anti-inflammatory, anti-apoptotic, and anti-cancer properties, and plays a positive role in impeding the development of many diseases, such as ovarian cancer,30 nonalcoholic steatohepatitis,31 and rheumatoid arthritis.32 Additionally, it has been found that pretreatment with diosmetin could ameliorate myocardial ischemia injury by decreasing oxidative stress in a dose-dependent manner.33 Importantly, diosmetin has been revealed to alleviate cerebral I/R injury in rats, which may be associated with its anti-oxidative activity, ability to inhibit apoptosis, and anti-inflammatory activity.34 However, the precise mechanism of the antioxidative activity of diosmetin in cerebral I/R has not been fully elucidated. The results of our present study revealed that diosmetin dramatically protected nerve function and reduced the cerebral infarct volume and pathological lesions in vivo, which are similar to the previous report.34Through in vitro experiments, we found that diosmetin could promote cell survival, increase cell vitality and reduce LDH release in OGD/R-induced PC12 cells. These results showed that diosmetin could effectively mitigate cerebral I/R injury. In addition, previous research also proved that diosmetin possessed antioxidative efficacy via the SIRT1 signaling pathway.21 Therefore, we further studied whether diosmetin could exert protective effects against cerebral I/R injury by attenuating oxidative stress by regulating the SIRT1 signaling pathway.
SIRT1, a class III histone deacetylase that is abundantly expressed in the brain, plays an important role in neuroprotection in a variety of neurological diseases, such as ischemia stroke,35 Alzheimer's disease36 and Parkinson's disease.37 SIRT1 can deacetylate protein substrates in various signal transduction pathways to regulate gene expression, cell apoptosis and senescence, and participate in the process of neuroprotection, energy metabolism, inflammation and oxidative stress.38 The activation of SIRT1 has been found to improve neurological dysfunction and reduce the cerebral infarct volume, while the inhibition of SIRT1 by EX527 could aggravate cerebral I/R injury.39,40 Importantly, SIRT1 can also regulate the key transcription factor of oxidative stress, Nrf2, which is activated by oxidative stress and involved in the regulation of the cellular redox balance and subsequently induces the coding of a series of antioxidant protective proteins, including NQO1 and HO-1.41 Additionally, it has been demonstrated that SIRT1 could upregulate Nrf2 expression and transcriptional activity and heighten the downstream anti-oxidase expression,10 whereas the downregulation of SIRT1 inhibits Nrf2 expression and increases the susceptibility of cells to oxidative stress.42 Therefore, the SIRT1/Nrf2 signaling pathway might play a vital role in the inhibition of oxidative stress.11 Both polyphenols and bioflavonoids have been considered as potent antioxidants in natural compounds. Hydroxytyrosol, a polyphenol mainly present in extra virgin olive oil, is regarded as an effective ROS scavenger that works by activating Nrf2 and decreasing oxidative stress-related biomarkers.43,44 In addition, the natural flavonoid diosmetin has been shown to improve cell viability and restrict I/R-induced oxidative stress via activating Nrf2,18,19 which is in accordance with our results. Our results showed that the nuclear location of Nrf2 was increased and the expressions of SIRT1, N-Nrf2, and the downstream proteins NQO1 and HO-1 were upregulated both in rats and in PC12 cells that were pretreated with diosmetin in a dose-dependent manner. In addition, to further elucidate the neuroprotective mechanisms of diosmetin, the SIRT1 inhibitor EX527 and siRNA-SIRT1 were used to inhibit SIRT1 expression. The results showed that the neuroprotective and antioxidative effects of diosmetin were eliminated in the EX527 administered group. EX527 downregulated the expression of Nrf2 and attenuated Nrf2 nuclear translocation and the levels of its downstream anti-oxidases NQO1 and HO-1. Furthermore, transfection with siRNA-SIRT1 was performed for the knockdown of SIRT1 in PC12 cells. Similar results were shown by in vitro experiments, and both the cytoprotective and antioxidative effects of diosmetin were reversed, and Nrf2 expression, nuclear translocation, and the levels of the downstream anti-oxidases NQO1 and HO-1 were dramatically suppressed. These results indicated that the protective effects of diosmetin (40 mg kg−1 and 80 mg kg−1) in cerebral I/R may be achieved by attenuating oxidative stress via the SIRT1/Nrf2 signaling pathway (Fig. 10). According to the guide for dose conversion between animals and humans,45 the human equivalent dose is 6.09 mg kg−1 and 12.18 mg kg−1 respectively. It has been reported that flavonoids are the major active ingredients in Trollius chinensis Bge, and 1.32 mg of diosmetin could be extracted from 1 g of Trollius chinensis Bge after conversion; thus a 60 kg adult should consume about 276.8 g of Trollius chinensis Bge to achieve the requirement of 6.09 mg kg−1 per day.46 In addition, Menthae Haplocalycis herba has been widely used as a food and as a medicinal compound for thousands of years in China. Pharmacological investigations of Menthae Haplocalycis herba have led to the extraction of flavonoids, such as diosmetin.12 HPLC fingerprint and determination of the components in Menthae Haplocalycis herba revealed that 0.07 mg diosmetin could be extracted from one gram of Menthae Haplocalycis herba;47 therefore, 5220 g of Menthae Haplocalycis herba is required for an adult to achieve the same effect that we observed in our animal experiments. Pharmacokinetic investigations revealed that diosmetin was absorbed and rapidly distributed throughout the body with a long plasma elimination half-life ranging from 24 to 43 hours. Diosmetin was degraded to phenolic acids or their glycine-conjugated derivatives and eliminated through urine, and not feces.48 However, further toxicology research and investigation of the minimum pharmacological activity of diosmetin in humans are required.
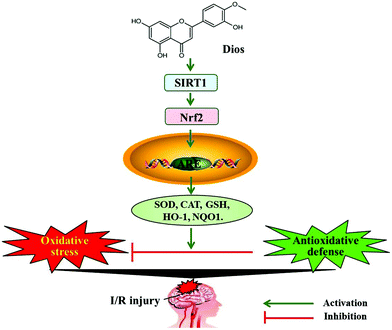 |
| Fig. 10 Possible mechanism of action of diosmetin in cerebral I/R injury. | |
5. Conclusion
In conclusion, our study indicated that diosmetin possessed neuroprotective effects against cerebral I/R both in vivo and in vitro, which were mediated by inhibiting oxidative stress via regulating the SIRT1/Nrf2 signaling pathway. These results suggest that diosmetin may serve as a potential therapeutic drug and dietary supplement for the treatment of cerebral I/R injury and provide experimental evidence for the clinical usage of diosmetin in the future.
Conflicts of interest
The authors declare that they have no conflicts of interest.
Acknowledgements
This work was supported by the Science and Technology Project of Yichang, Hubei (No. A18-302-a12); the Research Fund for Excellent Dissertation of China Three Gorges University (No. 2019SSPY111); the fund for Youth Top Talent Project of Hubei Provincial Health and Family Planning Commission (EWT-2019-48), and the Key Project of Hunan Province Education Department (20A366).
References
- E. J. Benjamin, S. S. Virani, C. W. Callaway, A. M. Chamberlain, A. R. Chang, S. Cheng, S. E. Chiuve, M. Cushman, F. N. Delling and R. Deo,
et al., Heart Disease and Stroke Statistics-2018 Update: A Report From the American Heart Association, Circulation, 2018, 137, e67–e492 CrossRef PubMed.
- M. Goyal, A. Y. Yu, B. K. Menon, D. W. J. Dippel, W. Hacke, S. M. Davis, M. Fisher, D. R. Yavagal, F. Turjman, J. Ross, S. Yoshimura, Z. Miao, R. Bhatia, M. Almekhlafi, Y. Murayama, S. Sohn, J. L. Saver, A. M. Demchuk and M. D. Hill, Endovascular Therapy in Acute Ischemic Stroke: Challenges and Transition from Trials to Bedside, Stroke, 2016, 47, 548–553 CrossRef PubMed.
- T. Leng and Z. G. Xiong, Treatment for ischemic stroke: From thrombolysis to thrombectomy and remaining challenges, Brain Circ., 2019, 5, 8–11 CrossRef PubMed.
- B. L. Ya, Q. Liu, H. F. Li, H. J. Cheng, T. Yu, L. Chen, Y. Wang, L. L. Yuan, W. J. Li and B. Bai, Uric Acid Protects against Focal Cerebral Ischemia/Reperfusion-Induced Oxidative Stress via Activating Nrf2 and Regulating Neurotrophic Factor Expression, Oxid. Med. Cell. Longevity, 2018, 2018, 6069150 Search PubMed.
- T. Kalogeris, C. P. Baines, M. Krenz and R. J. Korthuis, Ischemia/Reperfusion, Compr. Physiol., 2016, 7, 113–170 Search PubMed.
- M. Liu, H. Li, L. Zhang, Z. Xu, Y. Song, X. Wang, R. Chu, Y. Xiao, M. Sun, Y. Ma and W. Mi, Cottonseed Oil Alleviates Ischemic Stroke-Induced Oxidative Stress Injury via Activating the Nrf2 Signaling Pathway, Mol. Neurobiol., 2021, 58, 2494–2507 CrossRef CAS PubMed.
- P. Xu, Q. Liu, Y. Xie, X. Shi, Y. Li, M. Peng, H. Guo, R. Sun, J. Li, Y. Hong, X. Liu and G. Xu, Breast cancer susceptibility protein 1 (BRCA1) rescues neurons from cerebral ischemia/reperfusion injury through NRF2-mediated antioxidant pathway, Redox Biol., 2018, 18, 158–172 CrossRef CAS PubMed.
- P. Liu, J. Li, M. Liu, M. Zhang, Y. Xue, Y. Zhang, X. Han, X. Jing and L. Chu, Hesperetin modulates the Sirt1/Nrf2 signaling pathway in counteracting myocardial ischemia through suppression of oxidative stress, inflammation, and apoptosis, Biomed. Pharmacother., 2021, 139, 111552 CrossRef CAS PubMed.
- Y. M. Zhang, X. Y. Qu, L. N. Tao, J. H. Zhai, H. Gao, Y. Q. Song and S. X. Zhang, XingNaoJing injection ameliorates cerebral ischaemia/reperfusion injury via SIRT1-mediated inflammatory response inhibition, Pharm. Biol., 2020, 58, 16–24 CrossRef CAS PubMed.
- L. Chen, J. Cao, D. Cao, M. Wang, H. Xiang, Y. Yang, T. Ying and H. Cong, Protective effect of dexmedetomidine against diabetic hyperglycemia-exacerbated cerebral ischemia/reperfusion injury: An in vivo and in vitro study, Life Sci., 2019, 235, 116553 CrossRef CAS PubMed.
- J. Li, C. Yang and Y. Wang, miR-126 overexpression attenuates oxygen-glucose deprivation/reperfusion injury by inhibiting oxidative stress and inflammatory response via the activation of SIRT1/Nrf2 signaling pathway in human umbilical vein endothelial cells, Mol. Med. Rep., 2021, 23, 165 CrossRef CAS PubMed.
- W. Zhang, L. L. Xu, X. Yu, Y. Y. Jiang, J. Y. Zhang and B. Liu, Characterization and identification of the metabolites of Menthae Haplocalycis Herba water extracts in rat plasma, urine, and feces by ultra-high performance liquid chromatography with linear ion trap-Orbitrap mass spectrometry, J. Sep. Sci., 2020, 43, 1051–1062 CrossRef CAS PubMed.
- C. Wang, Y. Liao, S. Wang, D. Wang, N. Wu, Q. Xu, W. Jiang, M. Qiu and C. Liu, Cytoprotective effects of diosmetin against hydrogen peroxide-induced L02 cell oxidative damage via activation of the Nrf2-ARE signaling pathway, Mol. Med. Rep., 2018, 17, 7331–7338 CAS.
- D. H. Lee, J. K. Park, J. Choi, H. Jang and J. W. Seol, Anti-inflammatory effects of natural flavonoid diosmetin in IL-4 and LPS-induced macrophage activation and atopic dermatitis model, Int. Immunopharmacol., 2020, 89, 107046 CrossRef CAS PubMed.
- Q. Si, Y. Shi, D. Huang and N. Zhang, Diosmetin alleviates hypoxia-induced myocardial apoptosis by inducing autophagy through AMPK activation, Mol. Med. Rep., 2020, 22, 1335–1341 CrossRef CAS PubMed.
- S. Koosha, Z. Mohamed, A. Sinniah and M. A. Alshawsh, Investigation into the Molecular Mechanisms underlying the Anti-proliferative and Anti-tumorigenesis activities of Diosmetin against HCT-116 Human Colorectal Cancer, Sci. Rep., 2019, 9, 5148 CrossRef PubMed.
- W. Liao, Z. Ning, L. Chen, Q. Wei, E. Yuan, J. Yang and J. Ren, Intracellular antioxidant detoxifying effects of diosmetin on 2,2-azobis(2-amidinopropane) dihydrochloride (AAPH)-induced oxidative stress through inhibition of reactive oxygen species generation, J. Agric. Food Chem., 2014, 62, 8648–8654 CrossRef CAS PubMed.
- L. Luan, L. Cao, L. Zhu and J. Sun, Diosmetin ameliorates cerebral ischemia/reperfusion injury in pc12 cells through nuclear factor-kB (NF-kB) and nuclear factor erythroid 2-related factor/heme oxygenase-1 (Nrf2/HO-1) Pathway., Curr. Top. Nutraceutical Res., 2019, 17, 322–328 Search PubMed.
- K. Yang, W. F. Li, J. F. Yu, C. Yi and W. F. Huang, Diosmetin protects against ischemia/reperfusion-induced acute kidney injury in mice, J. Surg. Res., 2017, 214, 69–78 CrossRef CAS PubMed.
- E. Z. Longa, P. R. Weinstein, S. Carlson and R. Cummins, Reversible middle cerebral artery occlusion without craniectomy in rats, Stroke, 1989, 20, 84–91 CrossRef CAS PubMed.
- Z. Wen, W. Hou, W. Wu, Y. Zhao, X. Dong, X. Bai, L. Peng and L. Song, 6′-O-Galloylpaeoniflorin Attenuates Cerebral Ischemia Reperfusion-Induced Neuroinflammation and Oxidative Stress via PI3K/Akt/Nrf2 Activation, Oxid. Med. Cell. Longevity, 2018, 2018, 8678267 Search PubMed.
- C. Gu, L. Li, Y. Huang, D. Qian, W. Liu, C. Zhang, Y. Luo, Z. Zhou, F. Kong, X. Zhao, H. Liu, P. Gao, J. Chen and G. Yin, Salidroside Ameliorates Mitochondria-Dependent Neuronal Apoptosis after Spinal Cord Ischemia-Reperfusion Injury Partially through Inhibiting Oxidative Stress and Promoting Mitophagy, Oxid. Med. Cell. Longevity, 2020, 2020, 3549704 Search PubMed.
- C. Y. Huang, J. S. Deng, W. C. Huang, W. P. Jiang and G. J. Huang, Attenuation of Lipopolysaccharide-Induced Acute Lung Injury by Hispolon in Mice, Through Regulating the TLR4/PI3K/Akt/mTOR and Keap1/Nrf2/HO-1 Pathways, and Suppressing Oxidative Stress-Mediated ER Stress-Induced Apoptosis and Autophagy, Nutrients, 2020, 12, 1742 CrossRef CAS PubMed.
- M. Wang, L. Xia and G. Fu, Lipopolysaccharide pretreatment inhibits oxidative stress-induced endothelial progenitor cell apoptosis via a TLR4-mediated PI3K/Akt/NF-κB p65 signaling pathway, Cell. Mol. Biol., 2019, 65, 101–106 CrossRef.
- M. Mardones, R. Valenzuela, P. Romanque, N. Covarrubias, F. Anghileri, V. Fernandez, L. A. Videla and G. Tapia, Prevention of liver ischemia reperfusion injury by a combined thyroid hormone and fish oil protocol, J. Nutr. Biochem., 2012, 23, 1113–1120 CrossRef CAS PubMed.
- S. Samarghandian, M. Azimi-Nezhad, T. Farkhondeh and F. Samini, Anti-oxidative effects of curcumin on immobilization-induced oxidative stress in rat brain, liver and kidney, Biomed. Pharmacother., 2017, 87, 223–229 CrossRef CAS PubMed.
- H. Yu, Z. Wu, X. Wang, C. Gao, R. Liu, F. Kang and M. Dai, Protective effects of combined treatment with mild hypothermia and edaravone against cerebral ischemia/reperfusion injury via oxidative stress and Nrf2 pathway regulation, Int. J. Oncol., 2020, 57, 500–508 CrossRef CAS PubMed.
- T. Wang, F. Wang, L. Yu and Z. Li, Nobiletin alleviates cerebral ischemic-reperfusion injury via MAPK signaling pathway, Am. J. Transl. Res., 2019, 11, 5967–5977 CAS.
- C. G. Dossi, R. G. Vargas, R. Valenzuela and L. A. Videla, Beneficial effects of natural compounds on experimental liver ischemia-reperfusion injury, Food Funct., 2021, 12, 3787–3798 RSC.
- F. Zhao, X. Hong, D. Li, Z. Wei, X. Ci and S. Zhang, Diosmetin induces apoptosis in ovarian cancer cells by activating reactive oxygen species and inhibiting the Nrf2 pathway, Med. Oncol., 2021, 38, 54 CrossRef CAS PubMed.
- N. Luo, C. Yang, Y. Zhu, Q. Chen and B. Zhang, Diosmetin Ameliorates Nonalcoholic Steatohepatitis through Modulating Lipogenesis and Inflammatory Response in a STAT1/CXCL10-Dependent Manner, J. Agric. Food Chem., 2021, 69, 655–667 CrossRef CAS PubMed.
- Y. Chen, Y. Wang, M. Liu, B. Zhou and G. Yang, Diosmetin exhibits anti-proliferative and anti-inflammatory effects on TNF-α-stimulated human rheumatoid arthritis fibroblast-like synoviocytes through regulating the Akt and NF-κB signaling pathways, Phytother. Res., 2020, 34, 1310–1319 CrossRef CAS PubMed.
- G. Mo, Y. He, X. Zhang, X. Lei and Q. Luo, Diosmetin exerts cardioprotective effect on myocardial ischaemia injury in neonatal rats by decreasing oxidative stress and myocardial apoptosis, Clin. Exp. Pharmacol. Physiol., 2020, 47, 1713–1722 CAS.
- N. Zhang, X. Y. Qi, S. P. Pan, X. L. Zheng and Z. Z. Li, Protective effects of diosmetin on cerebral ischemia reperfusion injury in rats, Chin. J. Immunol., 2019, 035, 2996–3000 Search PubMed , 3007.
- J. F. Zhang, Y. L. Zhang and Y. C. Wu, The Role of Sirt1 in Ischemic Stroke: Pathogenesis and Therapeutic Strategies, Front. Neurosci., 2018, 12, 833 CrossRef PubMed.
- L. Zhu, F. Lu, X. Jia, Q. Yan, X. Zhang and P. Mu, Amyloid-β (25–35) regulates neuronal damage and memory loss via SIRT1/Nrf2 in the cortex of mice, J. Chem. Neuroanat., 2021, 114, 101945 CrossRef CAS PubMed.
- X. Li, Y. Feng, X. X. Wang, D. Truong and Y. C. Wu, The Critical Role of SIRT1 in Parkinson's Disease: Mechanism and Therapeutic Considerations, Aging Dis., 2020, 11, 1608–1622 CrossRef PubMed.
- Z. Ren, H. He, Z. Zuo, Z. Xu, Z. Wei and J. Deng, The role of different SIRT1-mediated signaling pathways in toxic injury, Cell. Mol. Biol. Lett., 2019, 24, 36 CrossRef PubMed.
- Z. G. Mei, Y. G. Huang, Z. T. Feng, Y. N. Luo, S. B. Yang, L. P. Du, K. Jiang, X. L. Liu, X. Y. Fu, Y. H. Deng and H. J. Zhou, Electroacupuncture ameliorates cerebral ischemia/reperfusion injury by suppressing autophagy via the SIRT1-FOXO1 signaling pathway, Aging, 2020, 12, 13187–13205 CrossRef CAS PubMed.
- L. Tan, X. Zhang, Z. Mei, J. Wang, X. Li, W. Huang and S. Yang, Fermented Chinese Formula Shuan-Tong-Ling Protects Brain Microvascular Endothelial Cells against Oxidative Stress Injury, J. Evidence-Based Complementary Altern. Med., 2016, 2016, 5154290 Search PubMed.
- T. H. Rushmore, M. R. Morton and C. B. Pickett, The antioxidant responsive element. Activation by oxidative stress and identification of the DNA consensus sequence required for functional activity, J. Biol. Chem., 1991, 266, 11632–11639 CrossRef CAS.
- M. T. Do, H. G. Kim, J. H. Choi and H. G. Jeong, Metformin induces microRNA-34a to downregulate the Sirt1/Pgc-1α/Nrf2 pathway, leading to increased susceptibility of wild-type p53 cancer cells to oxidative stress and therapeutic agents, Free Radicals Biol. Med., 2014, 74, 21–34 CrossRef PubMed.
- R. Valenzuela, P. Illesca, F. Echeverría, A. Espinosa, M. A. Rincon-Cervera, M. Ortiz, M. C. Hernandez-Rodas, A. Valenzuela and L. A. Videla, Molecular adaptations underlying the beneficial effects of hydroxytyrosol in the pathogenic alterations induced by a high-fat diet in mouse liver: PPAR-α and Nrf2 activation, and NF-κB down-regulation, Food Funct., 2017, 8, 1526–1537 RSC.
- P. Illesca, R. Valenzuela, A. Espinosa, F. Echeverría, S. Soto-Alarcon, M. Ortiz and L. A. Videla, Hydroxytyrosol supplementation ameliorates the metabolic disturbances in white adipose tissue from mice fed a high-fat diet through recovery of transcription factors Nrf2, SREBP-1c, PPAR-γ and NF-κB, Biomed. Pharmacother., 2019, 109, 2472–2481 CrossRef CAS PubMed.
- A. B. Nair and S. Jacob, A simple practice guide for dose conversion between animals and human, J. Basic Clin. Pharm., 2016, 7, 27–31 CrossRef PubMed.
- H. Y. Cai, D. T. Han, X. D. Yang, B. H. Zhang, G. H. Zheng, Z. L. Zhan and J. J. Hu, Determination of five flavone components in Trollius chinensis Bge by quantitative analysis of muti-components with a single-marker, J. Chin. Med. Mater., 2021, 44, 2157–2161 Search PubMed.
- W. Tian, S. S. Fan, Y. Q. Zhen, L. Gao, M. Wang and L. Y. Niu, Comparative Study on Non-volatile Ingredients of Menthae Haplocalycis Herba and Menthae Spicatae Herba Based on HPLC Fingerprints and Multi-components Content Determination, Chin. J. Inf. Tradit. Chin. Med., 2021, 28, 76–82 Search PubMed.
- D. Cova, L. De Angelis, F. Giavarini, G. Palladini and R. Perego, Pharmacokinetics and metabolism of oral diosmin in healthy volunteers, Int. J. Clin. Pharmacol., Ther. Toxicol., 1992, 30, 29–33 CAS.
Footnote |
† These authors contributed equally to this work. |
|
This journal is © The Royal Society of Chemistry 2022 |