DOI:
10.1039/D1FO03058B
(Paper)
Food Funct., 2022,
13, 186-197
Potential effects of mung bean protein and a mung bean protein–polyphenol complex on oxidative stress levels and intestinal microflora in aging mice
Received
13th September 2021
, Accepted 27th November 2021
First published on 1st December 2021
Abstract
This study investigated the effects of mung bean protein (MPI) and a MPI-polyphenol complex on oxidative stress levels and intestinal microflora in a D-galactose-induced aging mouse model. MPI and MPI-polyphenol complex intervention significantly increased activity of superoxide dismutase (SOD) and catalase and other antioxidant enzymes, improved the abundance and diversity of intestinal flora, and decreased the Firmicutes to Bacteroidetes ratio. Among them, the complex was more conducive to the improvement of the activity of antioxidant enzymes. The addition of MPI and the MPI-polyphenol complex can help the proliferation of Bacteroidetes, Bifidobacterium and Roseburia in the intestinal tract of aging mice, and inhibit the growth of Firmicutes and Ruminococcus, and the proliferation effect of the complex on Bifidobacterium was better than that of MPI. MPI significantly upregulated five pathways related to lipid and energy metabolism. Roseburia and Muribaculaceae were negatively correlated with malondialdehyde levels and positively correlated with SOD and other antioxidant enzyme indices. Our findings showed that MPI and MPI-polyphenol complexes can delay aging in mice by reducing oxidative damage and regulating intestinal flora. We also found a strong relationship between the abundance of intestinal flora and the levels of oxidative stress-related enzymes. This study provides theoretical support for the health and anti-aging benefits of mung bean food products.
1. Introduction
Senescence is an inevitable process of growth and a complex natural phenomenon that manifests as structural degeneration and functional decline.1 The oxygen free radical theory is the most widely studied and recognised hypothesis of the aging mechanism2 and posits that aging disturbs the balance of free radicals in the body. Aging can lead to the loss of physiological functions, including a weakened immune response. Multiple studies have found strong relationships between oxidative stress3 and the pathogenesis of age-induced inflammation and disease.4,5 The aging process is irreversible; therefore, delayed aging, or anti-aging, has become a major focus in many fields of research, including food and medicine. Research indicates that the ecology of intestinal flora is closely related to aging, and that intestinal ecological disorders could lead to accelerated aging and a shortened lifespan.6,7 However, Felice et al.8 found that the re-establishment of intestinal bacteria by transplantation of faecal bacteria can effectively reduce age-related diseases. Thus, maintaining a stable intestinal microecology is a potential strategy to mitigate the adverse effects related to aging. Abnormal oxidative stress levels can lead to notable fluctuations in intestinal flora, and pathological alterations of the body.9 Oxidative stress levels can be regulated through natural active ingredients, endogenous hormones, and new functional carrier materials, among which the regulation effects of proteins,10 polyphenols,11,12 peptides,13 and polysaccharides14 and other plant-derived antioxidants have become popular research topics. These substances can effectively reduce free radicals in the body by promoting antioxidant enzyme activity, and have significant effects on maintaining intestinal microecology and regulating the species composition of intestinal flora.15,16 It is therefore considered to be particularly important that natural plant-based antioxidants be included in the daily diet to prevent advanced aging.
Mung bean is a commonly used plant-grain with homology of medicine and food, and contains a high protein (mung bean protein or MPI) and active polyphenol content,17 has antioxidant effects,18 inhibits metabolic diseases, and regulates intestinal flora.19 A study found that vitexin, the main polyphenol substance in mung bean, could improve the activity and expression of antioxidant enzymes and reduce the prevalence of inflammation as well as the invasion of pathogenic bacteria.20 Additionally, MPI has been shown to repair oxidative damage and improve the community composition of intestinal flora.19 The interaction between proteins and polyphenols can improve the overall antioxidant capacity, increase the bioavailability of polyphenols, and promote the digestion and absorption of proteins.21,22 Current knowledge indicates that polyphenols and small molecule proteins have synergistic effects on the inhibition of aging23 and the regulation of intestinal bacteria. Research is needed into the effects of protein–polyphenol complexes on oxidative stress levels and intestinal microflora. Moreover, the functional role of intestinal microflora in the regulation of oxidative stress pathways and aging is even less clear.
Therefore, the effects of MPI and the MPI-polyphenol complex on the activity of antioxidant enzymes in the body were determined by measuring total antioxidant capacity (T-AOC), superoxide dismutase (SOD), glutathione peroxidase (GSH-Px), catalase (CAT), and malondialdehyde (MDA) indices in serum and liver tissue. Through rRNA sequencing, we analysed the effects of MPI and the MPI-polyphenol complex on abundance and diversity of intestinal flora, and described the statistical relationship between intestinal flora, antioxidant activity, and anti-aging. This study provides a novel evaluation of the function of MPI and the MPI-polyphenol complex for the development of foods with anti-aging properties.
2. Materials and methods
2.1 Materials and reagents
MPI and MPI-polyphenol complex were prepared according to the method described by our team.24 The MPI samples obtained by alkali extraction and acid precipitation samples were dispersed in deionised water and stirred for 2 h to fully hydrate the protein. The protein solution was diluted with a phosphate buffer to a concentration of 1 g per 100 mL. The mung bean polyphenol solution was mixed with protein solution, and the final polyphenol concentrations were 480 μmol per g pro. Because of its pronounced antioxidant activity in vitro, the proportion of the complex was 480 μmol per g protein. After reacting for 2 h at the specified temperature, the pH was adjusted to 4.6 with 5% trichloroacetic acid and centrifuged at 4000g for 15 min after kept still 30 min, took the precipitate for later used. (The preparation of MPI-polyphenol complex mainly relied on hydrophobicity to connect the two, and there were three binding sites. In the secondary structure of the complex, the deformation vibration of phenol-OH and the bending vibration outside the C–H plane of the benzene ring were observed, and the content of irregular crimping decreased. Compared with MPI, the ζ-potential absolute value increased, and the surface hydrophobicity decreased.)24D-Gal was obtained from Beijing Solarbio Science & Technology Co., Ltd (Beijing, China). Biochemical assay kit for T-AOC, CAT, GSH-Px, MDA, SOD, and protein were purchased from Jiancheng Institute of Biotechnology (Nanjing, China). Water-soluble vitamin E was provided by Tianjin Kemio Chemical Reagent Co., Ltd (Tianjin, China).
2.2 Animals and experimental design
All experiments were designed in strict accordance with Regulations for the Administration of Laboratory Animals and approved by the Ethics Committee of Animal Experiments of Heilongjiang Bayi Agricultural University, China. Thirty male KM mice (age 5–6 weeks) were purchased from Shanghai Lingchang Biological Technology Co., Ltd (Shanghai, China) (laboratory animal production license SCXK (Hu) 2018-0003; laboratory animal use licenseSYXK (Su) 2017-0040). Mice were reared in transparent cp-4 cages (L × W × H: 290 mm × 178 mm × 160 mm). The mice were acclimated for 3 days (12 h of alternating light and dark, ambient temperature ranging from 20 °C to 26 °C, and relative humidity ranging from 40% to 70%) and provided with standard feed (Beijing Keao Feed Co., Ltd) (feed production enterprise license SCXK (Beijing) 2019-0003) and water ad libitum.
After acclimation, six mice were randomly selected as the control group (Con). The remaining mice were subcutaneously injected with D-galactose (300 mg per kg body weight) to establish an aging model, and randomly divided into groups according to nutritional intervention: model group (M, D-galactose treatment only), mung bean protein intervention group (Pro, 250 mg d−1), mung bean protein–polyphenol complex intervention group (Complex, 250 mg d−1), and vitamin E (VE, 4 mg d−1). The protein dosage of the protein and complex intervention groups was increased to the maximum daily intake (84 g d−1) for adults (weight 60 g). The optimal intake of adult mice was calculated as 84 × 9.01/60 × 0.02 = 252 mg d−1.25,26 All treatments were administered intragastrically once a day for 42 days, and the control and model groups were administered saline of the same amount. During the experiment, mice in each group were monitored weekly to determine general condition, including hair colour, mental state, appetite, and activity pattern.
Determination of mouse aging model. The successful establishment of mice aging model was mainly determined by biochemical and intestinal bacteria tests. At the initial stage, the aging model was evaluated by observing the body weight, hair color, mental state and activity of mice during the experiment.27
2.3 Collection of serum, liver tissue and faecal samples
Blood was collected from the mouse eyeball at the end of the treatment period. After half an hour, the serum was separated at 4 °C and 12
000g for 20 min. The supernatant was collected and stored separately at −80 °C.
The mice were euthanized by dislocation of the spine, and the liver tissues were quickly removed and rinsed with chilled saline. Liver tissue samples were homogenised by adding a certain amount of PBS buffer (pH = 7.4) in an ice bath and centrifuged for 20 min at 3000 rpm. The supernatant was collected and stored at −80 °C.
Following the study period, fresh faeces (approximately 0.5 g) was collected from the end of the colon of each mouse and stored in a sterilised centrifuge tube at −80 °C.
2.4 Biochemical assays
The levels of serum and liver oxidative stress biomarkers, which superoxide dismutase (SOD), glutathione peroxidase (GSH-Px), catalase (CAT), and malondialdehyde (MDA) as well as total antioxidant capacity (T-AOC) were measured using the manufacturer's instructions (Jiancheng Institute of Biotechnology). The measured values were calculated according to the protein concentration of the sample, expressed using the BCA kit.
2.5 Analysis of intestinal flora
The Qubit dsDNA Assay Kit (Thermo Fisher Scientific, Waltham, MA, USA) was used to extract DNA from the faecal samples. The extracted DNA was used as the template, and the V3–V4 region of 16S rDNA was amplified using specific primers with barcodes determined by the sequencing region. The MiSeq platform was used for sequencing (Illumina, San Diego, CA, USA). The primers used were CCTAYGGGRBGCASCAG and GGACTACNNGGGTATCTAAT.
The original image data file obtained by high-throughput sequencing was transformed into the original sequence reads using Illumina bcl2fastq software for base calling. The original sequence number was obtained by analysis with fastq-join (version 1.3.1, https://code.google.com/p/ea-utils/) and PEAR software (version 0.9.11).28 Cutadapt (version 1.18)29 was then used to cut the number of optimised sequences for filtering. UCLUST software (version 11.0.667, http://www.drive5.com/usearch/) was used to qualify 97% of the sequence similarity clustering (operational taxonomic unit, OTU). Using the RDP Classifier algorithm (default confidence threshold of 0.8), OTU representative sequences with 97% similarity level were classified. QIIME software (http://qiime.org/) was used to generate abundance information tables at different classification levels.30 The RStudio was used to compare community structure maps at different taxonomic levels between samples, and the differences in community composition and key microflora species between intervention groups were analysed using UniFrac.
2.6 Statistical analysis
The results are expressed as the mean ± standard deviation (X ± S). The Tukey HSD test or Kruskal-Wallis test was used to analyse the differences in species diversity between groups. The PCA, PCoA and NMDS were used to analyze the distribution of possible dispersion and aggregation among different samples. SPSS software was used for univariate analysis of variance (ANOVA), and differences between groups were compared using the t-test. A significant difference between groups was indicated by p < 0.05.
3. Results and discussion
3.1 Preliminary evaluation of mice aging model
After 42 days of modeling, the physiological states of the model group and the control group were preliminarily determined. As can be seen from Fig. 1, mice in control group acted with dynamic, with hair smooth and good mental state. Aging model group of mice action slower, hair became white and fell off, poor mental state. It was also found that the weight increment of mice in the control group was more than control group 28 days before modeling. However, after 28 days, the final body weight of mice in the model group was lower than the control group, indicating that subcutaneously injected of D-galactose affected the normal growth and development of mice and the modeling was successful. After the intervention of MIP and MIP-polyphenol complex, the body weight of mice was slightly lower than that of normal mice, but there was no significant weight fluctuation, and in a state of continuous growth.
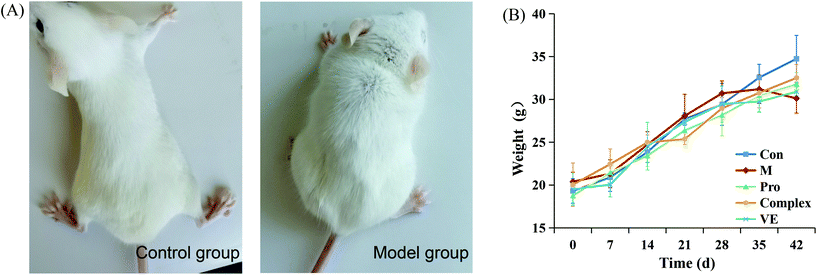 |
| Fig. 1 Physiological status indexes of mice. (A) Pictures of control mice and model mice 42 days after modeling. (B) Body weight of mice in five groups at different periods. | |
3.2 Effects of MPI and MPI-polyphenol complex on oxidative stress levels in aging mice
The effects of MPI and MPI-polyphenol complex on oxidative stress markers in the D-gal-induced aging mouse model were determined by measuring serum total protein, T-AOC, SOD, CAT, GSH-Px, and MDA. Compared with the control group, the serum total protein content in the model group was reduced (p < 0.05), and T-AOC levels decreased (p < 0.01) (Fig. 2). The activities of major antioxidant enzymes in the serum and liver decreased significantly (p < 0.01), while MDA levels increased (p < 0.01) (Fig. 2 and 3). Multiple studies have successfully induced aging in a mouse model. Similar to the present study, El-Tantawy et al.31 found that D-galactose could reduce the activities of T-AOC, SOD, and GSH-Px, and increase the level of MDA to induce oxidative damage.
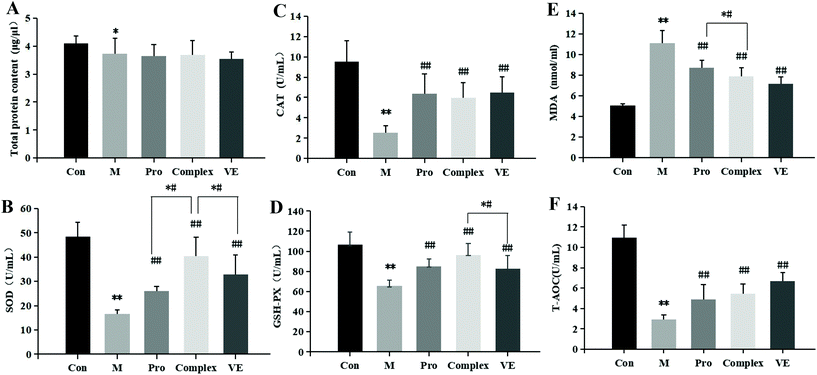 |
| Fig. 2 Effects of MPI and MPI-polyphenol complex intervention on the total protein content (A); SOD (B), CAT (C), GSH-Px (D), and T-AOC (F) activity levels; and MDA (E) levels in blood serum of aging mice. Note: * indicates a significant difference between the model group and the control group, and ** indicates an extremely significant difference; # indicates a significant difference between the model group and the intervention group, and ## indicates an extremely significant difference; *# indicates significant differences between intervention groups. | |
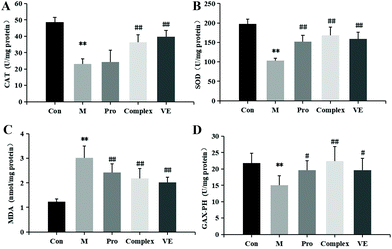 |
| Fig. 3 Effect of mung bean nutrient components intervention on the CAT (A), SOD (B), GSH-Px (D) activity levels; MDA (C) level in liver homogenate of aging mice. Note: * indicates a significant difference between the model group and the control group, and ** indicates an extremely significant difference; # indicates a significant difference between the model group and the intervention group, and ## indicates an extremely significant difference; *# indicates significant differences between intervention groups. | |
Compared with the model group, MPI, MPI-polyphenol complex, and VE intervention groups experienced increased activity of serum T-AOC, and the activities of SOD, CAT, and GSH-Px in serum and liver tissue were increased, with MDA content decreased to different degrees. However, total protein content in the serum was not significantly affected. The three intervention groups showed significantly improved activity of antioxidant enzymes and reduced accumulation of MDA, but the activity of antioxidant enzymes was still lower than that of normal group. Yu et al.32 found that the regulation of antioxidant enzyme activity could prevent endogenous oxidative stress and aging. Our results indicate that the MPI and MPI-polyphenol complex can regulate the level of oxidative stress in the body and can promote the repair of D-gal-induced oxidative damage.
As seen in Fig. 3B, 2D and E, subsequent to MPI and MPI-polyphenol complex treatments, SOD in serum respectively increased by 57.33% and 144.67%, GSH-Px activity levels respectively increased by 29.76% and 47.27%, and MDA content was respectively reduced to 8.73 nmol mL−1 and 7.88 nmol mL−1. Compared to a single protein component, the MPI-polyphenol complex group had a more obvious effect on oxidative stress injury. In addition, the MPI-polyphenol complex was more effective at regulating oxidative stress levels than VE, and VE was more effective than MPI. Exogenous antioxidants may carry phenolic hydroxyl groups, remove excess free radicals, maintain the balance of oxygen metabolism in cells, and improve the expression of endogenous antioxidant enzymes. Compared to the other interventions, the antioxidant capacity of the protein–polyphenol complex was significantly increased, and possibly because protein protection improves the bioavailability and stability of polyphenols.21 Polyphenols can pass through the upper digestive tract to the colon and are more easily absorbed and transported by the monolayer. Antioxidant defence levels are evidently stronger in the protein–polyphenol complex.33,34
3.3 Effects of MPI and MPI-polyphenol complex intake on intestinal microflora of aging mice
3.3.1 Analysis of species diversity.
The Sobs, Ace, and Chao1 indices were used to estimate abundance of intestinal microflora. The Simpson and Shannon indices were used to calculate species diversity.35 As shown in Table 1, compared with the control group, the abundance of intestinal flora was significantly decreased in the model group, and there was no significant change in diversity. In the MPI group, Shannon indices indicated significantly higher diversity than in the control group, though the Simpson indices did not reflect this result. Sobs, Ace, Chao1, and Shannon indices increased with the MPI-polyphenol complex and VE interventions, and the Simpson index decreased, though not significantly so. The Venn diagram and OTU distribution diagram (Fig. 4D) compare species richness (total of 438) between intervention groups, including 138 non-exclusive species and 19 endemic species at the OTU level. The number of species in the model group was significantly lower than that in the control group, and increased after administration of any treatments, especially in the MPI intervention group. Moreover, MPI group were more strains of the same bacteria with the control group. The MPI group, MPI-polyphenol complex group, and VE group had five, four, and two unique OTUs, respectively. The three groups produced their own unique flora after each intervention, indicating that nutrients had an important influence on intestinal flora colonisation. According to the above results, both MPI and MPI-polyphenol complex could improve the abundance and diversityof intestinal bacteria in an aging mouse model.
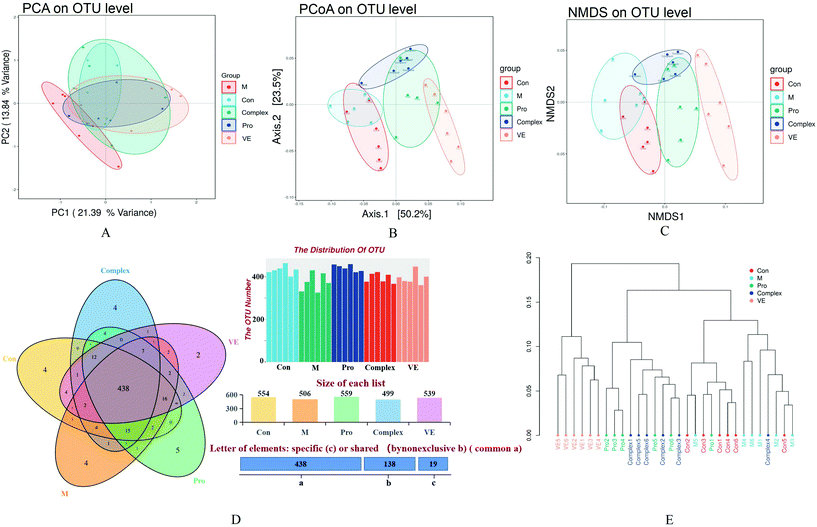 |
| Fig. 4 Intestinal microflora species assessments and study group comparisons. (A) PCA plot for the OTU level of each group, (B) PCoA plot for the OTU level of each group, (C) NMDS analysis, (D) Venn diagrams for the OTU level of each group, and (E) hierarchical clustering analysis. Groups Con, M, Pro, Complex, and VE represented the control, aging, mung bean protein, mung bean protein–polyphenol complex, and vitamin E study groups, respectively. | |
Table 1 Comparison of microbial alpha diversity index values between the different intervention groups
Group |
Sobs index |
Ace index |
Chao1 index |
Shannon index |
Simpson index |
Con |
417.83 ± 20.47 |
464.15 ± 27.89 |
468.03 ± 30.82 |
4.04 ± 0.35 |
0.06 ± 0.03 |
M |
364.17 ± 42.61** |
409.77 ± 30.64* |
411.76 ± 33.05** |
3.71 ± 0.64 |
0.07 ± 0.04 |
Pro |
430.83 ± 16.20## |
472.51 ± 16.95## |
481.09 ± 19.48## |
4.29 ± 0.23## |
0.04 ± 0.02 |
Complex |
376.50 ± 25.68 |
423.32 ± 19.90 |
436.17 ± 16.14 |
3.95 ± 0.31 |
0.06 ± 0.03 |
VE |
380.17 ± 25.83 |
421.07 ± 24.51 |
418.69 ± 24.31 |
3.82 ± 0.33 |
0.06 ± 0.02 |
The combined analysis of faecal samples by principal component analysis (PCA), PCA of unweighted Unifrac (PCoA), and non-metric multidimensional scaling (NMDS) plots showed that the intestinal flora of the control and model groups were almost separated, the model group was completely separated from the intervention group, and the bacterial community structure was significantly different (Fig. 4A–C). There was aggregation between the MPI group and the MPI-polyphenol complex group, but also some degree of separation, and the VE group was separated significantly. These analyses suggest significant differences in the species abundance of faecal flora among the five groups. According to the statistical results of the differences of intestinal flora samples in each group, hierarchical cluster analysis was conducted on the samples (Fig. 4E). The VE group formed a separate cluster, and the MPI and MPI-polyphenol complex groups were similarly clustered, with little variation. The clusters of the intervention and control groups were significantly separated from the model group. These results confirmed that there were differences in the intestinal microflora between the control and model groups. This difference appeared to be reduced under the intervention of MPI and MPI-polyphenol complex.
3.3.2 Species composition analysis.
Faecal samples were analysed using high-throughput sequencing, and the species composition of intestinal microflora was compared based on the classification results of each sample at the levels of phylum, class, order, family, genus, and species (Fig. 5). The community composition of microflora was analysed at the phylum level. A total of 14 phyla were identified, including (in order of relative abundance) Bacteroidetes, Firmicutes, Campilobacterota, Proteobacteria, Patescibacteria, Desulfobacterota, Actinobacteriota, Armatimonadota, Bdellovibrionota, Cyanobacteria, Deferribacterota, Gemmatimonadota, and Verrucomicrobiota, Unclassified, the first seven were the main flora, of which Bacteroidetes and Firmicutes had the highest relative abundance, accounting for more than 80% of intestinal microflora in all faecal samples, both of which were the dominant flora of intestinal flora. In the model group, the abundance of Firmicutes increased, and the abundance of Bacteroidetes decreased (Fig. 5A, 6A and B). Studies have shown that senescence reduces the abundance of essential core species and increases the abundance of subdominant species, which is consistent with the results reported in the literature.36–38 However, the F/B ratio decreased following MPI and MPI-polyphenol complex intervention (the abundance of Firmicutes decreased and that of Bacteroidetes increased) (Fig. 6A–C). These results suggested that MPI and MPI-polyphenol complexes could promote intestinal health by regulating the abundance of the two dominant bacteria.
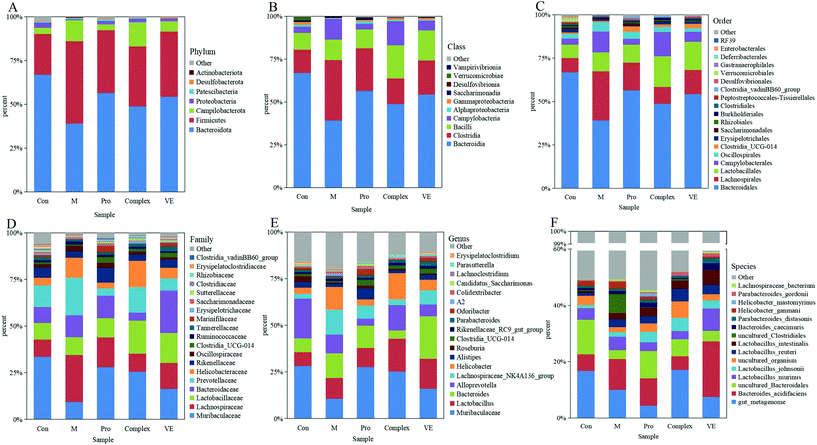 |
| Fig. 5 The relative abundance of intestinal microbiota in mice. The level of Phylum (A), Class (B), Order (C), family (D), genus (E), and species (F). | |
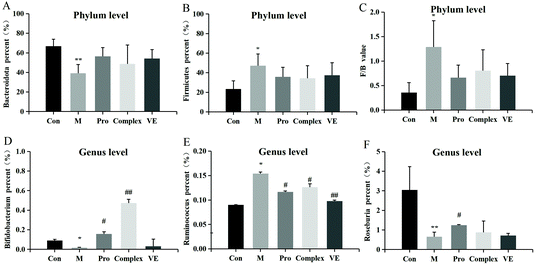 |
| Fig. 6 The relative changes in abundance at phylum and genus level of intestinal microflora in an aging mouse model. The enclosed figures (A), (B) and (C) represent phylum level, and (D), (E) and (F) represent genus level. Note: * indicates a significant difference between the model group and the control group, and ** indicates an extremely significant difference; # indicates a significant difference between the model group and the intervention group, and ## indicates an extremely significant difference. | |
Intestinal microflora were subsequently analysed at genus level to describe niche changes (Fig. 5E). The results showed that the abundance of Muribaculaceae was significantly different between the model group and the intervention groups. This bacterium belongs to the Bacteroidetes phylum and is common in the gut microbiome. The abundance of Muribaculaceae was low in the model group and increased significantly in the MPI intervention group, where it reached a comparable level of abundance with the control group. Its prevalence might be due to the expression of many active enzymes in its genome, promoting the effective degradation of macromolecular nutrients.39 The abundance of Muribaculaceae in the MPI-polyphenol complex group and VE group was relatively low, possibly because absorption of polyphenols in the gut required microbial degradation.40 The abundance of Lactobacillus was also increased in the MPI-polyphenol complex and VE groups after intervention. Recent studies have demonstrated similar trends under low oxidative stress levels induced by a high antioxidant diet.41 The abundance of Bacteroides and Alloprevotella changed in the model group, and these two genera were closely related to intestinal type changes.42 In addition, the fluctuation of F/B value could also lead to changes in the intestinal type, and the results were consistent with those at the phyla level. There were also significant differences in the community composition at class, order, family, and species level between the model group, the control group, and the intervention groups (Fig. 5B–D and F). These results indicated that aging had a strong interference effect on the ecology of intestinal flora, whereas MPI and MPI-polyphenol complex had a significant regulatory effect.
Compared with the control group, Bifidobacterium and Roseburia were significantly decreased in the model group, Ruminococcus was significantly increased, while the occurrence of this above phenomenon was reduced in the intervention groups (Fig. 6D–F). The abundance of three intestinal bacteria was associated with aging, and similar trends in abundance for these three intestinal bacteria have been reported in previous studies of aging.43,44 In addition, linear discriminant analysis (LDA) confirmed that the count of Bifidobacterium was significantly altered by intervention of the MPI-polyphenol complex (Fig. 7). We found that the anti-aging effects of MPI and the MPI-polyphenol complex were closely related to the changes in Ruminococcus, Bifidobacterium, and Roseburia.
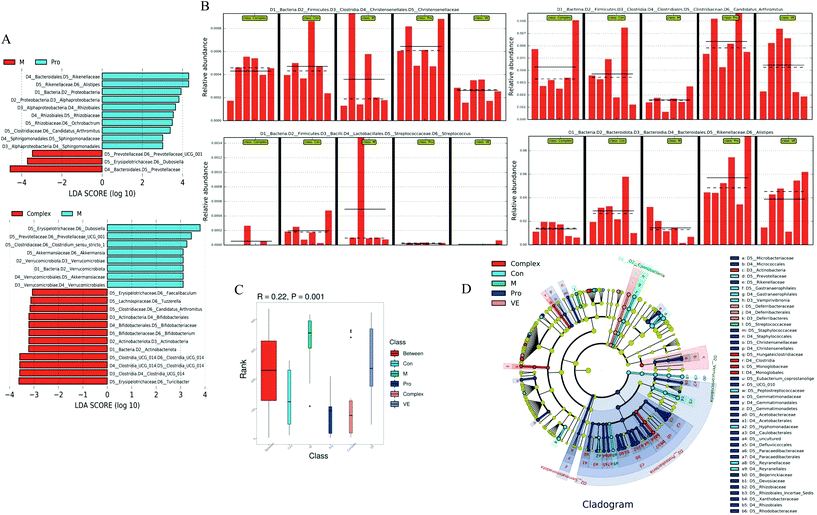 |
| Fig. 7 Analysis of characteristic differences of intestinal flora species in mice. (A) Histogram of LDA value distribution. (B) Histogram of horizontal relative abundance between groups. (C) Anosim boxplot. (D) Analysis of evolutionary branching. Anosim similarity analysis R-value >0, p < 0.05. The LDA Score was 3.0. | |
3.3.3 Analysis of characteristic differences of species.
The analysis of similarity (ANOSIM) indicated that species composition was significant different between groups and the differences between groups were significantly greater than within groups (R-value = 0.22, p < 0.05) (Fig. 7C). LEfSe analysis showed species with an LDA score greater than 3.0 (Kruskall-Wallis, p < 0.05). This analysis could be used to compare species abundance between multiple groups. Fig. 7D shows that 8, 1, 26, 5, and 3 bacterial branches were detected in five groups (the differences were statistically significant and biologically consistent), and the abundance of Streptococcaceae was significantly different in the model group compared to the control and intervention groups. The relative abundance of streptococci in the intestinal flora of mice in the model group was significantly increased (p < 0.05) (Fig. 7B). Research has found that Streptococcus is dominant in atherosclerotic plaques, a common age-induced disease,45 and the change in Streptococcus might increase the risk of disease in older adults. Compared with the model group, the relative abundance of Streptococcus was decreased in the three intervention groups, while the relative abundance of Alistipes, Candidatus-Arthromitus, and Christensenellaceae was significantly increased (Fig. 7B). Christensenellaceae, Candidatus-Arthromitus and Alistipes were negatively correlated with BMI, inflammation and other metabolic diseases,46 and are therefore considered to be important in immune regulation and host health.47
3.3.4 Enrichment analysis of pathways.
PICRUSt software was used to evaluate gene sequencing data to identify functional gene families and compare changes in metabolic pathways between the microbial communities specific to each study group.48 The predictive results, based on the Kyoto Encyclopaedia of Genes and Genomes (KEGG) database, showed that a total of 17 functional gene families were involved (Fig. 8). Functional pathway analysis of intestinal flora revealed that the naphthalene degradation pathway was significantly downregulated after D-gal-induced senescence, and downregulation of this pathway might lead to liver and kidney damage as well as carcinogenesis.49 The functional pathways that were upregulated in the control and MPI intervention groups were related to limonene and pinene degradation, linoleic acid metabolism, bisphenol degradation, xenobiotic metabolism by cytochrome P450, and photosynthesis. MPI-polyphenol complex group only significantly upregulated linoleic acid metabolic pathway.
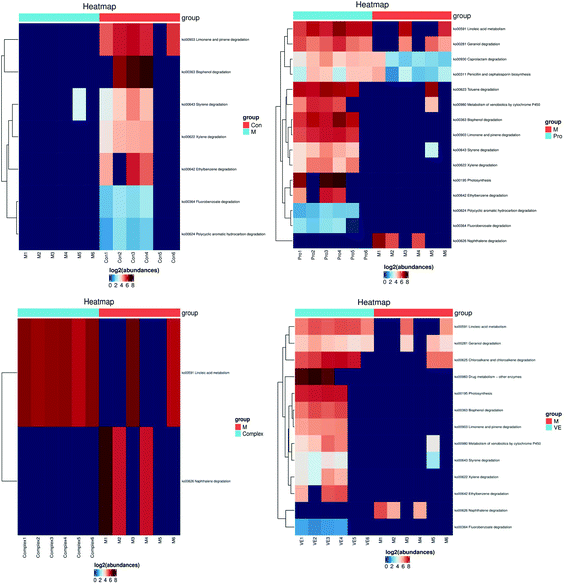 |
| Fig. 8 Metabolic pathway analysis of mung bean nutrient components in aging mice. The KEGG Pathway clustering heat map illustrates high levels of abundance as red and low levels of abundance as blue. | |
The linoleic acid metabolism pathway is closely related to D-gal-induced aging.50 Limonene and pinene degradation and photosynthesis metabolism pathways are related to body fat metabolism and energy metabolism.51 With an increase in aging, the activity of enzymes involved in fat metabolism decreases, leading to surplus fat synthesis over metabolism. However, the MPI and VE intervention could upregulate this pathway, which might have an antagonistic effect on body fat accumulation. In conclusion, both MPI and MPI-polyphenol complex can significantly regulate metabolic pathways closely related to aging, and MPI could also significantly relieve aging symptoms by regulating fat and energy metabolism.
3.4 Relationship between antioxidant index and intestinal flora
Pearson's correlation coefficient was used to describe the relationship between bacterial abundance and the oxidative stress index (Fig. 9). At the genus level, the Lachnospiraceae NK4A136 group was negatively correlated with CAT in serum and liver tissue, and negatively correlated with SOD and GSH-Px. Ruminococcus was positively correlated with MDA levels. However, the biochemical pathways related to the Lachnospiraceae NK4A136 group and Ruminococcus, and the relationship between their functions and oxidative stress, require further investigation. Fig. 9 shows that Roseburia and Muribaculaceae were negatively correlated with MDA and positively correlated with other antioxidant enzyme indices. Roseburia in the gut is sensitive to oxidative stress as it lacks the usual antioxidant enzyme defence system, which weakened ability to reduce free radicals led to a significant decline in abundance.52 Therefore, Lachnospiraceae NK4A136 group, Ruminococcus, Roseburia, and Muribaculaceae might be the key bacteria species involved in regulating oxidative stress and delaying aging.
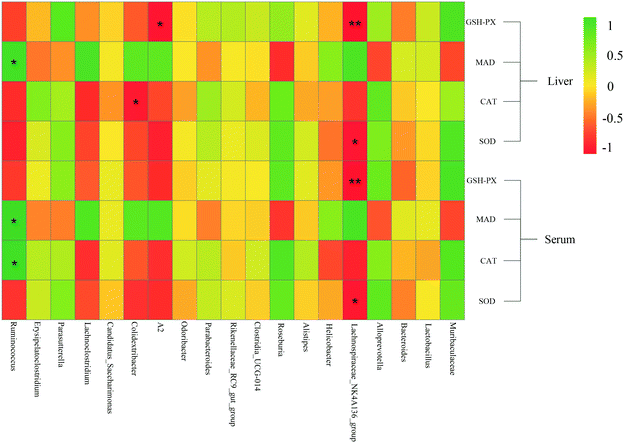 |
| Fig. 9 Heat map of the correlation between mung bean nutritional components intervention on dominant intestinal flora and antioxidant indices in aging mice. Red represents a negative correlation, green represents a positive correlation, * represents p < 0.05, and ** represents p < 0.01. | |
4. Conclusion
In the D-gal-induced aging mouse model, MPI and MPI-polyphenol complex could regulate oxidative stress levels by increasing the levels of antioxidant enzymes and MDA secretion, and the advantage of MPI-polyphenol complex regulating enzyme activity was more significant. At the same time, MPI and MPI-polyphenol complexes could effectively regulate the abundance and diversity of intestinal flora, improve the F/B ratio, significantly inhibit the growth of potential pathogens, and promote the proliferation of beneficial bacteria. This regulation is closely related to lipid and energy metabolism. In addition, the abundance of Roseburia and Muribaculaceae in mice was significantly correlated with enzymes related to the regulation of oxidative stress. This study suggested that MPI and MPI-polyphenol complexes could play an anti-aging role by regulating the level of oxidative stress and promoting balance in the microecology of intestinal flora.
Compliance with ethics requirements
This article does not contain any studies with human participants or performed by any of the authors.
Author contributions
Changyuan Wang: conceptualization (lead); project administration (supporting); funding acquisition (lead). Dongjie Zhang: conceptualization (supporting); methodology (lead); resources (supporting). Shu Zhang: formal analysis (lead); writing original draft (lead); investigation (lead); writing – review & editing (lead). Yantao Ma: visualization (lead); data curation (lead); investigation (lead). Yuchao Feng: methodology (supporting); writing – review & editing (supporting).
Conflicts of interest
The authors declare no competing financial interest.
Acknowledgements
We would like to thank Editage for English language editing. This work was supported by National Key Research and development programs (Grant Number: 2018YFE0206300), (Grant Number: SQ2018YFE020070), and Heilongjiang Province engineering science and technology major special project of China (Grant Number: 2021ZX12B06).
References
- C. Franceschi, P. Garagnani, P. Parini, C. Giuliani and A. Santoro, Inflammaging: A new immune-metabolic viewpoint for age-related diseases, Nat. Rev. Endocrinol., 2018, 14, 576–590 CrossRef CAS PubMed.
- D. Harman, Aging: a theory based on free radical and radiation chemistry, J. Gerontol., 1956, 11, 298–300 CrossRef CAS PubMed.
- F. Kleefeldt, U. Rueckschloss and S. Ergün, CEACAM1 promotes vascular aging processes, Aging, 2020, 12, 3121–3123 CrossRef PubMed.
- J. Teixeira, M. Feio and M. L. Figueira, The role of oxidative stress in aging and dementia, PsiLogos, 2014, 12, 43–57 Search PubMed.
- Y. Yoshitaka, M. Tomonori, O. Kazuhiko and S. Tazuko, Relationship between hyposalivation and oxidative stress in aging mice, J. Clin. Biochem. Nutr., 2017, 61, 40–46 CrossRef PubMed.
- S. Kim and S. M. Jazwinski, The gut microbiota and healthy aging: A mini-review, Gerontology, 2018, 64, 1–8 CrossRef PubMed.
- J. Qin, R. Li and J. Raes, A human gut microbial gene catalogue established by metagenomic sequencing, Nature, 2010, 464, 59–65 CrossRef CAS PubMed.
- V. D. Felice, E. M. Quigley, A. M. Sullivan, G. W. O'Keeffe and S. M. O'Mahony, Microbiota-gut-brain signalling in parkinson's disease: implications for non-motor symptoms, Parkinsonism Relat. Disord., 2016, 27, 1–8 CrossRef.
- X. Y. Liu, C. X. Wu, D. Han, J. Liu and Z. Q. Jiang, Partially hydrolyzed guar gum attenuates D-galactose-induced oxidative stress and restores gut microbiota in rats, Int. J. Mol. Sci., 2019, 20, 4 Search PubMed.
- X. C. Yu, Z. Li, X. R. Liu, J. N. Hu, R. Liu, N. Zhu and Y. Li, The antioxidant effects of whey protein peptide on learning and memory improvement in aging mice models, Nutrients, 2021, 13, 2100 CrossRef CAS PubMed.
- G. Petruk, R. D. Giudice, M. M. Rigano, D. M. Monti and L. Bravo, Antioxidants from plants protect against skin photoaging, Oxid. Med. Cell. Longevity, 2018, 2018, 1–11, DOI:10.1155/2018/1454936.
- L. Zhao, H. Q. Yang, M. L. Xu, X. Wan, C. T. Wang, Y. H. Lian, A. Mehmood and H. C. Dai, Stevia residue extract ameliorates oxidative stress in D-galactose-induced aging mice via Akt/Nrf2/HO-1 pathway, Nutraceuticals Funct. Med. Foods, 2019, 52, 587–595 CrossRef CAS.
-
P. Y. Wang, Study on preparation of pumpkin seed peptides and their anti-aging effect, Master thesis, Jiangnan University, China, 2020 Search PubMed.
- W. Xia, Z. H. Xiu, L. Zhe, Y. Ran and H. J. Zeng, Investigations on the anti-aging activity of polysaccharides from Chinese yam and their regulation on klotho gene expression in mice, J. Mol. Sruct., 2020, 1208, 127895 CrossRef.
-
T. T. Liang, The regulatory mechanism of lipid metabolism in plant proteins based on improvement of intestinal microorganism, Master thesis, Shanxi University of Science and Technology, China, 2018 Search PubMed.
- T. T. Liang, L. T. Tong, D. H. Geng, L. L. Wang, X. R. Zhou, H. Y. Pu, W. Jia, Q. P. Wu and J. R. Huang, Wheat gluten regulates cholesterol metabolism by modulating gut microbiota in hamsters with hyperlipidemia, J. Oleo Sci., 2019, 68, 909–922 CrossRef CAS PubMed.
- N. Gupta, N. Srivastava and S. S. Bhagyawant, Vicilin-A major storage protein of mung bean exhibits antioxidative potential, antiproliferative effects and ace inhibitory activity, PLoS One, 2018, 13, e0191265 CrossRef.
- M. Kohno, H. Sugano, Y. Shigihara, Y. Shiraishi and T. Motoyama, Improvement of glucose and lipid metabolism via mung bean protein consumption: clinical trials of GLUCODIA™ isolated mung bean protein in the USA and Canada, J. Nutr. Sci. Vitaminol., 2018, 7, e21–e11 Search PubMed.
- A. Nakatani, X. Li, J. Miyamoto, M. Igarashi, H. Watanabe, A. Sutou, K. Watanabe, T. Motoyama, N. Tachibana, M. Kohno, H. Inoue and I. Kimura, Dietary mung bean protein reduces high-fat diet-induced weight gain by modulating host bile acid metabolism in a gut microbiota-dependent manner, Biochem. Biophys. Res. Commun., 2018, 501, 955–961 CrossRef CAS PubMed.
- S. Li, T. Liang, Y. Zhang, K. Huang, S. Y. Yang, H. Y. Lv, Y. Chen, C. H. Zhang and X. Guan, Vitexin alleviates high-fat diet induced brain oxidative stress and inflammation via anti-oxidant, anti-inflammatory and gut microbiota modulating properties, Free Radical Biol. Med., 2021, 171, 332–344 CrossRef CAS PubMed.
- S. Rohn, H. M. Rawel and J. Kroll, Antioxidant activity of protein-bound quercetin, J. Agric. Food Chem., 2004, 52, 4725 CrossRef CAS PubMed.
- Z. L. Wan, J. M. Wang, L. Y. Wang, Y. Yuan and X. Q. Yang, Complexation of resveratrol with soy protein and its improvement on oxidative stability of corn oil/water emulsions, Food Chem., 2014, 161, 324–331 CrossRef CAS PubMed.
- J. Xiao, B. Liu and Y. Zhuang, Effects of rambutan (Nephelium lappaceum) peel phenolics and Leu-Ser-Gly-Tyr-Gly-Pro on hairless mice skin photoaging induced by ultraviolet irradiation, Food Chem. Toxicol., 2019, 129, 30–37 CrossRef CAS PubMed.
- S. Zhang, Y. N. Sheng, Y. C. Feng, J. J. Diao, C. Y. Wang and D. J. Zhang, Changes in structural and functional properties of globulin-polyphenol complexes in mung beans: exploration under different interaction ratios and heat treatment conditions, Int. J. Food Sci. Technol., 2021 DOI:10.1111/ijfs.15180.
- J. X. Luo and S. W. Xu, Study on protein intake of china's residents, Agric. Outlook, 2017, 13, 71–76 Search PubMed.
- J. H. Huang, X. H. Huang, Z. Y. Chen, Q. S. Zheng and R. Sun, Dose conversion among different animals and healthy volunteers in pharmacological study, Chin. J. Clin. Pharmacol. Ther., 2004, 9, 1069–1072 Search PubMed.
- A. Shc, Y. Dan, A. Ypw, Y. B. Ran, B. Lq and A. Hjz, Effect of resveratrol on the repair of kidney and brain injuries and its regulation on klotho gene in d-galactose-induced aging mice, Bioorg. Med. Chem. Lett., 2021, 40, 127913 CrossRef.
- J. J. Zhang, K. Kobert, T. Flouri and A. Stamatakis, PEAR: a fast and accurate Illumina Paired-End reAd mergeR, Bioinformatics, 2014, 30, 614–620 CrossRef CAS PubMed.
- M. Martin, Cutadapt removes adapter sequences from high-throughput sequencing reads, EMBnet. J., 2011, 17, 10–12 CrossRef.
- J. G. Caporaso, J. Kuczynski and J. Stombaugh, QIIME allows analysis of high-throughput community sequencing data, Nat. Methods, 2010, 7, 335–336 CrossRef CAS.
- W. H. El-Tantawy, Antioxidant effects of spirulina supplement against lead acetate-induced hepatic injury in rats. African Journal of Traditional, BMC Complementary Altern. Med., 2016, 6, 327–331 Search PubMed.
- X. C. Yu, Z. Li, X. R. Liu, J. N. Hu, R. Liu, N. Zhu and Y. Li, The antioxidant effects of whey protein peptide on learning and memory improvement in aging mice models, Nutrients, 2021, 13, 2100 CrossRef CAS.
- M. J. T. J. Arts, G. R. M. M. Haenen, H. P. Voss and A. Bast, Masking of antioxidant capacity by the interaction of flavonoids with protein, Food Chem. Toxicol., 2001, 39, 787–791 CrossRef CAS PubMed.
- Y. F. Wang and X. Y. Wang, Binding, stability, and antioxidant activity of quercetin with soy protein isolate particles, Food Chem., 2015, 188, 24–29 CrossRef CAS PubMed.
-
L. Hu, Effect of EGCG on gut microbiota diversity and fat deposition in KM mice, Master thesis, South China Agricultural University, China, 2018 Search PubMed.
- S. H. Piao, Z. Q. Zhu, S. Y. Tan, H. X. Zhan, X. L. Rong and J. Guo, An integrated fecal microbiome and metabolome in the aged mice reveal anti-aging effects from the intestines and biochemical mechanism of FuFang zhenshu TiaoZhi(FTZ), Biomed. Pharmacother., 2019, 121, 109421–109421 Search PubMed.
-
Z. T. Yin, Protective effect of Procyanidin B2 on metabolomics and gut microbiota of aging model induced by D-galactose, Master thesis, Shanghai Institute of Technology, China, 2018 Search PubMed.
- V. Ravichandra, S. Tanvi, G. Rohit, G. Shakuntla, K. Avinash, B. David, M. Christopher and E. Rajaraman, Lactobacillus acidophilus DDS-1 modulates the gut microbiota and improves metabolic profiles in aging mice, Nutrients, 2018, 10, 1255 CrossRef PubMed.
- I. Lagkouvardos, T. R. Lesker and T. C. A. Hitch, Sequence and cultivation study of Muribaculaceae reveals novel species, host preference, and functional potential of this yet undescribed family, Microbiome, 2019, 7, 28–43 CrossRef PubMed.
- P. Dey, Gut microbiota in phytopharmacology: A comprehensive overview of concepts, reciprocal interactions, biotransformations and mode of actions, Pharmacol. Res., 2019, 147, 104367 CrossRef CAS.
- S. Singh, R. K. Sharma, S. Malhotra, R. Pothuraju and U. K. Shandilya, Lactobacillus rhamnosus NCDC17 ameliorates type-2 diabetes by improving gut function, oxidative stress and inflammation in high-fat-diet fed and streptozotocintreated rats, Benef. Microbes, 2017, 8, 243–255 CrossRef CAS PubMed.
- P. I. Costea, F. Hildebrand and M. Arumugam, Enterotypes in the landscape of gut microbial community composition, Nat. Microbiol., 2017, 3, 8–16 CrossRef PubMed.
- S. Kim and S. M. Jazwinski, The gut microbiota and healthy aging: a mini-review, Gerontology, 2018, 64, 513–520 CrossRef CAS PubMed.
- M. J. Claesson, I. B. Jeffery and S. Conde, Gut microbiota composition correlates with diet and health in the elderly, Nature, 2012, 488, 178–184 CrossRef CAS PubMed.
- C. Y. W. Patrick, L. L. T. Jade, K. P. L. Susanna and K. Y. Yuen, Clinical, phenotypic, and genotypic evidence for Streptococcus sinensis as the common ancestor of anginosus and mitis groups of streptococci, Med. Hypotheses, 2006, 66, 345–351 CrossRef PubMed.
- J. L. Waters and R. E. Ley, The human gut bacteria Christensenellaceae are widespread, heritable, and associated with health, BMC Biol., 2019, 17, 641–648 CrossRef.
- K. Pawan, M. Leticia and C. Patricia, Intestinal interleukin-17 receptor signaling mediates reciprocal control of the gut microbiota and autoimmune inflammation, Immunity, 2016, 44, 659–671 CrossRef PubMed.
- M. G. Langille, J. Zaneveld and J. G. Caporaso, Predictive functional profiling of microbial communities using 16S rRNA marker gene sequences, Nat. Biotechnol., 2013, 31, 814–821 CrossRef CAS PubMed.
- D. Kartik, S. R. Subashchandrabose, V. Kadiyala, K. Kannan and M. Mallavarapu, Anaerobic microbial degradation of polycyclic aromatic hydrocarbons: a comprehensive review, Rev. Environ. Contam. Toxicol., 2020, 251, 25–108 Search PubMed.
-
S. C. Wang, Anti-aging effect and mechanism of camellia oleifera oil, Master thesis, Shanghai Jiao Tong University, China, 2019 Search PubMed.
- S. Greenblum, P. J. Turnbaugh and E. Borenstein, Metagenomic systems biology of the human gut microbiome reveals topological shifts associated with obesity and inflammatory bowel disease, Proc. Natl. Acad. Sci. U. S. A., 2011, 109, 594–599 CrossRef PubMed.
-
Y. Qiao, The effect of oxidative stress induced by high fat diet on changes of gut microbiota and inflammation in mice, PhD thesis, Jiangnan University, China, 2014 Search PubMed.
|
This journal is © The Royal Society of Chemistry 2022 |