DOI:
10.1039/D0FO02794D
(Paper)
Food Funct., 2021,
12, 373-386
Lactobacillus plantarum Y44 alleviates oxidative stress by regulating gut microbiota and colonic barrier function in Balb/C mice with subcutaneous D-galactose injection†
Received
26th October 2020
, Accepted 19th November 2020
First published on 21st November 2020
Abstract
Probiotics have been proved to ameliorate the symptoms of the host induced by oxidative stress. In this study, the protective effects of Lactobacillus plantarum Y44 on Balb/C mice injured by D-galactose (D-gal)-injection were examined. Six weeks of continuous subcutaneous D-gal injection caused liver and colon injury of the Balb/C mice. L. plantarum Y44 administration significantly reversed the injury by modulating hepatic protein expressions related to the Nrf-2/Keap-1 pathway, and enhancing expressions of colonic tight junction proteins. L. plantarum Y44 administration restored the D-gal injection-induced gut microbiota imbalance by manipulating the ratio of Firmicutes/Bacteroidetes (F/B) and Proteobacteria relative abundance at the phylum level, and manipulating relative abundances of Lactobacillaceae, Muribaculaceae, Ruminococcaceae, Desulfovibrionaceae, and Prevotellaceae at the family level. Moreover, the D-gal injection-induced glycerophospholipid metabolism disorder was ameliorated, evidenced by the decline of phosphatidyl ethanolamine (PE), phosphatidylcholine (PC), phosphatidyl serine (PS), and lysophosphatidyl choline (LysoPC) levels in the serum of the mice after the L. plantarum Y44 administration. Spearman correlation analysis revealed a significant correlation between changes in gut microbiota composition, glycerophospholipid levels, and oxidative stress-related indicators. In summary, L. plantarum Y44 administration ameliorated D-gal injection-induced oxidative stress in Balb/C mice by manipulating gut microbiota and intestinal barrier function, and further influenced the glycerophospholipid metabolism and hepatic Nrf-2/Keap-1 pathway-related protein expressions.
1. Introduction
Oxidative stress is usually caused by an imbalance between accumulated reactive oxygen species (ROS) and intracellular antioxidant defense systems, which results in various chronic human diseases, such as aging, inflammation, Alzheimer's disease, Parkinson's disease, and even cancer.1–3 The intracellular antioxidant defense systems are insufficient to relieve oxidative stress in some circumstances such as alcohol consumption, smoking, and high-fat diet.4 Therefore, consuming some antioxidants to prevent oxidative stress is vital for health.
Numerous studies have paid attention to probiotics, commonly lactobacilli strains, having the potential to act as an antioxidant to protect the host from oxidative stress.5,6 Some lactobacilli strains were found to quench oxygen free radicals by using a chemical antioxidant method. Lactobacillus plantarum (L. plantarum) Ln4 was found to have higher 1-diphenyl-2-picrylhydrazyl radical (DPPH) scavenging and β-carotene oxidation-inhibitory activities.7 It was also reported that some lactobacilli strains could protect cell models, such as HepG2 cells, and Caco-2 cells, against oxidative stress.8,9 In addition, L. plantarum MA2 was found to colonize in the gastrointestinal tract and exert a protective effect on mice against D-galactose (D-gal)-induced oxidative stress.10 However, the animal model antioxidant method was reliable in elucidating the antioxidant activity of lactobacilli strains after chemical and cellular model assays.11,12
The development of high-throughput microbiome and metabolomics approaches had led to a deep understanding of the relationship between the host's health, gut microbiota, and metabolite level.13,14 Previous studies proved that hyperglycemia,15 colitis,16 and type 2 diabetes17 were correlated with the imbalance of the gut microbiota and metabolite level. The oxidative stress is associated with the alteration of the gut microbiota, and metabolite level.18–20 Several lactobacilli strains were surmised to attenuate oxidative stress of the host by manipulating gut microbiota, or regulating the metabolite level.11,12,21
In the present study, the ameliorative effect of L. plantarum Y44 administration on D-gal-induced oxidative stress of the Balb/C mice was investigated by examining the hepatic antioxidant activities, Nrf-2/Keap-1 pathway and colon tight junction (TJ) protein expressions of the Balb/C mice. Moreover, the gut microbiota diversity and composition, and serum metabolite levels were analyzed by high-throughput approaches to investigate the possible correlation between the gut microbiota, metabolite level, and antioxidant activity in the Balb/C mice.
2. Materials and methods
2.1 Bacterial strains and growth conditions
L. plantarum Y44 was stored in de Man, Rogosa, and Sharp (MRS) broth medium (Land Bridge Technology, Beijing, China) containing 25% glycerol at −80 °C at Dalian Probiotics Function Research Key Laboratory. L. plantarum Y44 was cultured in MRS broth (Land Bridge Technology) at 37 °C for 18 h twice prior to use. The bacterial cells were harvested by centrifugation at 2010g for 10 min, 4 °C (Eppendorf, Hamburg, Germany). Cell pellets were washed twice with normal saline and re-suspended in the normal saline. Cell numbers were adjusted to approximately 1.0 × 108 CFU mL−1 and 1 × 109 CFU mL−1 for the oral supplement to Balb/C in different groups, respectively.
2.2 Animal administration and experimental design
All animal experiments were approved in accordance with the Guidelines of the Dalian Polytechnic University Experimental Animal Ethics Committee under the approved protocol number (Liao) SYXK2017-0005. Fifty eight-week-old male SPF grade Balb/c mice were purchased from Liaoning Changsheng Biotechnology Company (Benxi, Liaoning, China) and kept under environmentally controlled conditions at the Animal Center of Dalian Polytechnic University (Dalian, Liaoning, China) at a temperature of 22 ± 2 °C, a relative humidity of 50% ± 10%, and 12/12 h of automatic lighting. All the mice were permitted free access to drinking water and a standard diet purchased from Dalian Medical University (Dalian, Liaoning, China).
After a one-week adaptation, the mice were randomly divided into five groups (10 mice per group). Groups were named and treated for 6 weeks as follows. (1) Control (CK) group, 0.85% normal saline injection + 0.85% normal saline gavage once daily; (2) D-galactose (D-gal, DG) group, D-gal (800 mg per kg body weight, Sigma-Aldrich, St Louis, MO) injection + 0.85% normal saline gavage once daily; (3) High Concentration (HC) group, D-gal (800 mg per kg body weight) injection + 109 CFU mL−1L. plantarum Y44 gavage; (4) Low Concentration (LC) group, D-gal (800 mg per kg body weight) injection + 108 CFU mL−1L. plantarum Y44 gavage; and (5) ascorbic acid (VC) group, D-gal (800 mg per kg body weight) injection + ascorbic acid (mg per kg body weight, Sigma-Aldrich) gavage once daily. The injection volume and gavage volume were 200 μL for each mouse. The ascorbic acid (VC) group was used as the positive control.
The body weight of the mice was measured weekly. After 6 weeks of continuous administration, the mice were deprived of diet and water for 24 h and then were weighed, anesthetized, and sacrificed. The liver and colon tissue were collected and immediately frozen in liquid nitrogen and stored at −80 °C.
2.3 Histological analysis of liver and colon
Fresh liver and colon of the mice in all groups were collected and immediately fixed with 4% paraformaldehyde for 48 h. Then the tissues were dehydrated and embedded in paraffin wax, and made into 5–8 μm slices. After dewaxing, tissue samples were observed by hematoxylin and eosin (H&E) staining. Histological images were photographed under an optical microscope (Olympus ix73, Japan).
2.4 Hepatic antioxidant enzyme activities and malondialdehyde (MDA) level
The liver tissue samples in all groups were homogenized in 9-fold cold 0.85% normal saline to obtain 10% (w/v) homogenates. Then the homogenate was centrifuged at 2010g at 4 °C for 10 min, and the supernatant was obtained for further assay. The superoxide dismutase (SOD), glutathione peroxidase (GPx), and catalase (CAT) activities and MDA levels in the hepatic homogenates were determined using the detection kit provided by Nanjing Jiancheng Bioengineering Institute (Nanjing, Jiangsu, China) following the manufacturer's instructions. Protein concentration was determined using the BCA protein assay kit (Solarbio Life Science, Beijing, China).
2.5 Western blotting analysis
The nuclear and cytoplasmic protein of the liver tissue was extracted using a nuclear and cytoplasmic protein extraction kit (Solarbio Life Science) following the manufacturer's instructions. The total protein of colon tissue was extracted using a total protein extraction kit (Solarbio Life Science) following the manufacturer's instructions. Protein concentrations were determined using the BCA protein assay kit (Solarbio Life Science). Equal amounts of protein from each sample were subjected to 12% (wt/vol) SDS-PAGE and transferred to polyvinylidene difluoride (PVDF) membranes. The membranes were blocked with TBS containing 0.05% Tween-20 and 5% skimmed milk at room temperature for 2 h. Membranes were incubated overnight at 4 °C with primary antibodies against occludin (1:500, abclonal, Wuhan, Hubei, China), Claudin-1 (1:500, ABclonal), nuclear factor E2-related factor 2 (Nrf-2, 1:1000, ABclonal), Kelch-like ECH associated protein1 (Keap-1, 1:1000, ABclonal), heme oxygenase-1, (HO-1, 1:1000, ABclonal), β-actin (1:5000, Biosynthesis Biotechnology, Beijing, China) and lamin-B (1:1000, Biosynthesis Biotechnology). The target proteins were incubated using the corresponding horseradish peroxidase-labeled secondary antibodies (Beyotime Institute of Biotechnology, Shanghai, China, 1:2000) for 1 h, followed by detection using a BeyoECL Star Kit (Beyotime Institute of Biotechnology) following the manufacturer's instructions. The band intensity was then quantified using ImageJ software (National Institutes of Health, Bethesda, MD, U.S.A) and normalized to the expression of human β-actin or human lamin-B.
2.6 Gut microbiota analysis
All instruments for collecting feces were sterilized at 121 °C for 20 minutes. Feces of the mice in all groups were collected before the end of the experiment and stored at −80 °C. Microbial DNA from the feces was extracted using the E.Z.N.A.® soil DNA Kit (Omega Biotek, Norcross, GA, U.S.A) according to the manufacturer's instruction. The final DNA concentration and purification were determined using a NanoDrop 2000 UV-vis spectrophotometer (Thermo Scientific, Wilmington, USA), and DNA quality was checked by 1% agarose gel electrophoresis. The hypervariable region V3–V4 of the bacterial 16S rRNA gene was amplified with primer pairs 338F (5′-ACTCCTACGGGAGGCAGCAG-3′) and 806R (5′-GGACTACHVGGGTWTCTAAT-3′) by an ABI GeneAmp® 9700 PCR thermocycler (ABI, CA, USA). Purified 16S rRNA gene amplicons were pooled in equimolar quantities and paired-end sequenced on an Illumina MiSeq platform (Illumina, San Diego, USA), according to the standard protocols by Majorbio Bio-Pharm Technology Co. Ltd (Shanghai, China).
2.7 Serum metabolomics analysis
Serum samples were obtained from blood samples of the mice in all groups through centrifugation at 2010g for 10 min at 4 °C and stored at −80 °C after being frozen in liquid nitrogen. 100 μL serum was accurately weighed, and the metabolites were extracted using a 400 μL acetonitrile
:
methanol (1
:
1, v/v) solution. The mixture was vortexed for 30 s and ultrasound was performed at 40 kHz for 30 min at 5 °C. The samples were placed at −20 °C for 30 min to precipitate proteins. After centrifugation (13
000g, 4 °C, 15 min) the supernatant was dried with nitrogen. The dry powder was re-suspended in 120 μL acetonitrile
:
water (1
:
1, v/v) solution, then followed by ultrasound at 40 kHz for 5 min at 5 °C. After centrifuging at 13
000g at 4 °C for 15 min, the supernatant was carefully transferred to sample vials for LC-MS/MS analysis. As a part of the system conditioning and quality control process, a pooled quality control sample (QC) was prepared by mixing equal volumes of all samples.
After preparations, the mixtures were analyzed on an ExionLCTMAD UPLC system (AB Sciex, USA) coupled to a quadrupole-time-of-flight mass spectrometer (Triple TOF, TM5600+, AB Sciex, USA) equipped with an electrospray ionization (ESI) source operating in positive mode and negative mode. The raw data were imported into the Progenesis QI 2.3 (Nonlinear Dynamics, Waters, USA) for peak detection and alignment. The internal standard was used for data QC (reproducibility). The metabolites with the features in relative standard deviation (RSD) of QC > 30% were discarded. Following normalization procedures and imputation, statistical analysis was performed on log-transformed data to identify significant differences in metabolite levels between comparable groups. Mass spectra of these metabolic features were identified by using the accurate mass, MS/MS fragment spectra, and isotope ratio difference with searching in reliable biochemical databases such as the Human metabolome database (HMDB) (http://www.hmdb.ca/). In concrete terms, the mass tolerance between the measured m/z values and the exact mass of the components of interest was ±10 ppm. For metabolites having an MS/MS confirmation, only the ones with the MS/MS fragment score above 30 were considered as confidently identified. Otherwise, metabolites had only tentative assignments. The metabolites with variable importance in projection (VIP) values >1 in the OPLS-DA model and p < 0.05 were regarded as differential metabolites. The relevant pathways were performed using metabolomics pathway analysis (MetPA, http://www.metaboanalyst.ca) based on the Kyoto Encyclopedia of Genes and Genomes (KEGG, http://www.genome.jp/kegg). In this study, the Mus musculus (mouse) pathway library was selected to search for the possible metabolic pathways.
2.8 Statistical analysis
All data were expressed as mean ± standard deviation (SD). One-way ANOVA was assessed with SPSS (version 20.0, SPSS Inc., Chicago, IL, U.S.A.) to verify significant differences between samples. The results were considered significant when p < 0.05.
3. Results
3.1 Effect of L. plantarum Y44 administration on body weight and tissue histopathology of the D-gal-injected Balb/C mice
As shown in Fig. 1A, body weights of mice in all groups were increased during the 6 weeks (Fig. 1A). The weight gain of the DG group was significantly lower than those in other groups (p < 0.05, Fig. 1B), although body weights of the mice in all groups were not significantly different (p > 0.05).
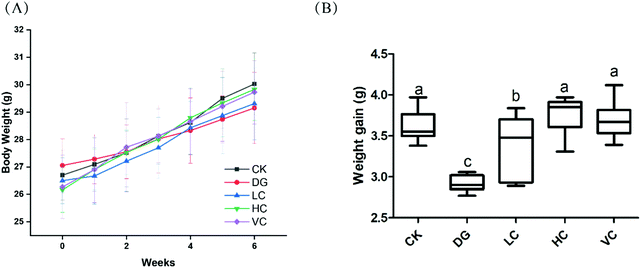 |
| Fig. 1 Effects of L. plantarum Y44 administration on body weights of Balb/C mice. (A) Body weight of Balb/C mice and (B) weight gain of Balb/C mice. The results are expressed as means ± SD. Different lowercase letters (a–c) show significant difference between groups (p < 0.05). CK, Control group; DG, D-galactose group; HC, high concentration group; LC, low concentration group; and VC, ascorbic acid group. | |
The hepatic H&E staining in all groups is presented in Fig. 2. In the CK group, the liver of the mice showed a normal structure with well-preserved cell morphology and a prominent nucleus. After D-gal injection, symptoms such as swollen hepatocytes, cytoplasmic vacuolization, fat vacuoles, and karyopyknosis were found in the liver of the mice in the DG group. However, the histological changes were reversed by L. plantarum Y44 and ascorbic acid administrations. The ameliorative effect of 109 CFU mL−1L. plantarum Y44 administration was more effective, evidenced by a loss of swollen hepatocytes, cytoplasmic vacuolization, fat vacuoles, and karyopyknosis, which was similar to that observed in the VC group (Fig. 2).
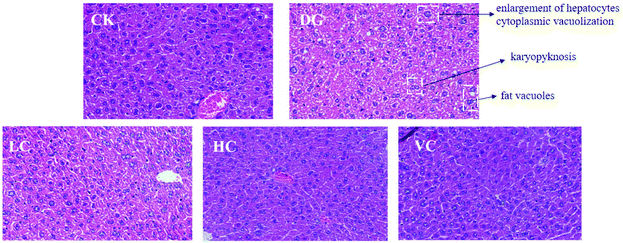 |
| Fig. 2 Histopathological changes of the liver stained with H&E of the Balb/C mice in different groups (original magnification of 400×). CK, Control group; DG, D-galactose group; HC, high concentration group; LC, low concentration group; and VC, ascorbic acid group. | |
The colonic H&E staining results of the mice in all groups are presented in Fig. 3. The colon structure of the mice in the CK group exhibited regular colon crypts, complete mucosal epithelium, and abundance of goblet cells. After D-gal injection, the colonic structure of the mice in the DG group showed crypt structure atrophy, mucosal epithelium impairment, and decreased goblet cells. In contrast, colonic structures of the mice in the HC group and VC group were obviously improved, with restored crypt structure and increased goblet cells (Fig. 3).
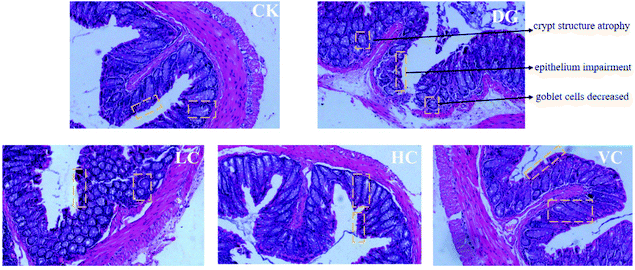 |
| Fig. 3 Histopathological changes of the colon stained with H&E of the Balb/C mice in different groups (original magnification of 200×). CK, Control group; DG, D-galactose group; HC, high concentration group; LC, low concentration group; and VC, ascorbic acid group. | |
3.2 Effect of L. plantarum Y44 administration on the liver oxidative status of the D-gal-injected Balb/C mice
The hepatic antioxidant enzyme activities and MDA level of the mice in all groups are shown in Fig. 4. Compared to the CK group, D-gal injection significantly increased the hepatic MDA level of the mice in the DG group (p < 0.05). After L. plantarum Y44 and ascorbic acid administrations, the MDA levels significantly decreased compared to those in the DG group (p < 0.05). However, the activities of hepatic superoxide dismutase (SOD), glutathione peroxidase (GPx), and catalase (CAT) in the DG group were significantly lower than those in the CK group (p < 0.05, Fig. 4B–D), while the administrations of 109 CFU mL−1L. plantarum Y44 and ascorbic acid reversed the declines in the SOD, GPx, and CAT activities of the mice injected with D-gal (p < 0.05, Fig. 4B–D), respectively.
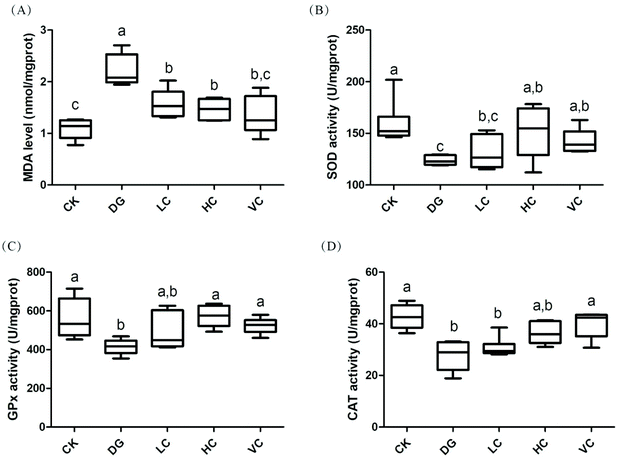 |
| Fig. 4 Effect of L. plantarum Y44 administration on hepatic antioxidant enzyme activities and MDA level of the Balb/C mice. (A) MDA level, (B) superoxide dismutase (SOD) activity, (C) glutathione peroxidase (GPx) activity, and (D) catalase (CAT) activity. The results are expressed as means ± SD. Different lowercase letters (a–c) show a significant difference between groups (p < 0.05). CK, Control group; DG, D-galactose group; HC, high concentration group; LC, low concentration group; and VC, ascorbic acid group. | |
3.3 Effect of L. plantarum Y44 administration on the Nrf-2/Keap-1 signal pathway related protein expression of the D-gal-injected Balb/C mice
The nuclear factor erythroid-2 related factor 2 (Nrf2)/Kelch like ECH associated protein1 (Keap-1) pathway plays an important role in regulating the defense against oxidative stress, which could activate the expression of the anti-oxidative response element (ARE).22 The hepatic Nrf-2, Keap-1, and HO-1 protein expressions of the D-gal-injected Balb/C mice were determined to identify whether the Nrf-2/Keap-1 pathway was activated by L. plantarum Y44 administration. D-Gal injection significantly inhibited Nrf-2 translocation in the nucleus of the mice in the DG group, compared to that in the CK group (p < 0.05, Fig. 5A). The inhibited Nrf-2 translocation was significantly reversed after 109 CFU ml−1L. plantarum Y44 and ascorbic acid administrations (p < 0.05, Fig. 5A). In addition, L. plantarum Y44 and ascorbic acid administrations significantly promoted Keap-1 degradation in the cytoplasm (p < 0.05, Fig. 5B).
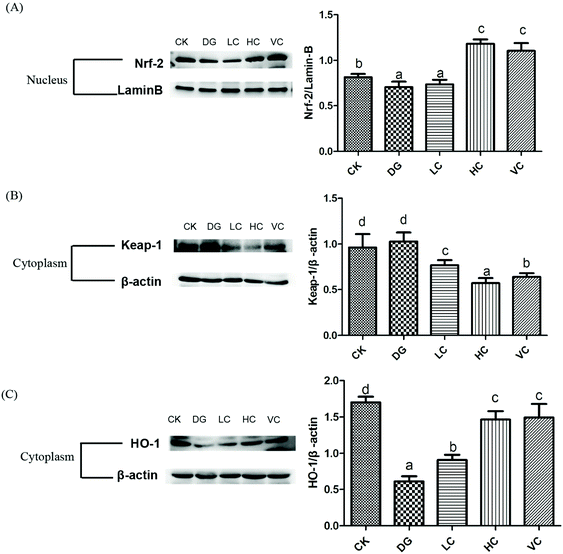 |
| Fig. 5 Effect of L. plantarum Y44 administration on Nrf-2 protein expression in hepatic cell nuclear (A), and Keap-1 (B), HO-1 (C) protein expression in hepatic cell cytoplasm of the Balb/C mice. The results are expressed as means ± SD. Different lowercase letters (a–d) show a significant difference between groups (p < 0.05). CK, Control group; DG, D-galactose group; HC, high concentration group; LC, low concentration group; and VC, ascorbic acid group. | |
HO-1 expression was also determined to confirm whether the downstream target gene of the Nrf-2/Keap-1 pathway was activated. As shown in Fig. 5C, the HO-1 expression of the mice in the DG group was significantly inhibited by D-gal (p < 0.05), and this down-regulation was reversed by L. plantarum Y44 administration (p < 0.05, Fig. 5C). The results indicated that L. plantarum Y44 administration could activate the hepatic Nrf-2/Keap-1 pathway against D-gal injection-induced oxidative stress of the mice.
3.4 Effect of L. plantarum Y44 administration on the intestinal barrier function of the D-gal-injected Balb/C mice
Tight junction protein (TJ) is a junctional complex including many types of epithelial and endothelial cells, which act as a barrier function regulating the permeability of solutes between adjacent cells.23–25
In our study, D-gal injection caused crypt structure atrophy, mucosal epithelium impairment, and decreased goblet cells of the mice in the DG group (Fig. 3), indicating that intestinal barrier function of the mice might be spoiled. As shown in Fig. 6, compared to the CK group, Claudin-1 and Occludin protein expressions in the colon of the DG group significantly decreased (p < 0.05). L. plantarum Y44 administration significantly reversed the downregulation of claudin-1 and occludin protein expressions (p < 0.05, Fig. 6). The expressions of TJ proteins in the colon of the HC group were higher than those of other groups (p < 0.05, Fig. 6), indicating that L. plantarum Y44 administration enhanced the intestinal barrier function of mice through increasing TJ protein expression. Based on the results mentioned above, the HC group was selected for further experiments, with the VC group used as a positive control.
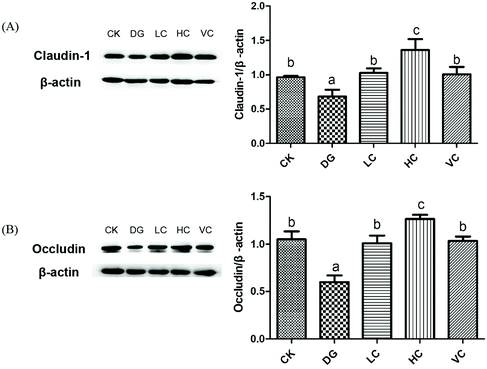 |
| Fig. 6 Effect of L. plantarum Y44 administration on the tight junction protein expression of the Balb/C mice. (A) Claudin-1, and (B) occauldin. The results are expressed as means ± SD. Different lowercase letters (a–d) show a significant difference between groups (p < 0.05). CK, Control group; DG, D-galactose group; HC, high concentration group; LC, low concentration group; and VC, ascorbic acid group. | |
3.5 Effect of L. plantarum Y44 administration on gut microbiota of the D-gal-injected Balb/C mice
A total of 949
850 16S rDNA raw gene sequences from 20 samples (5 samples in each group) were obtained through Illumina MiSeq sequencing, including 223
132 sequences in the CK group, 216
157 sequences in the DG group, 252
571 sequences in the HC group, and 257
990 sequences in the VC group. As shown in Fig. S1A and S1B,† Shannon, and Simpson indices of the HC group were significantly different from those of the DG group (p < 0.05), without no difference with those of the VC group (p > 0.05). As shown in Fig. S1C,† 540 OTUs were shared by all groups, which suggests a strong core gut microbiota. There were 6, 11, 11, and 10 OTUs regarded as unique taxa in CK, DG, HC, and VC groups, respectively (Fig. S1C†). Furthermore, the principal coordinate analysis (PCoA) of unweighted UniFrac distance and partial least squares discrimination analysis (PLS-DA) were employed to illustrate the difference among all groups on gut microbiota composition. As shown in Fig. S1D,† the CK, HC, and VC group were grouped together, and the DG group was drastically dropped and farther than other groups. The PLS-DA result showed that the HC and VC groups were clustered together (Fig. S1E†). These findings indicated that L. plantarum Y44 administration altered the diversity of gut microbiota undermined by D-gal injection, which displayed a similar effect with ascorbic acid administration.
The difference in gut microbiota composition at the phylum level is shown in Fig. 7A and B. The most abundant phyla in all groups were Bacteroidetes, Firmicutes, Proteobacteria, and Actinobacteria. Compared to the CK group, the relative abundance of Bacteroidetes and Proteobacteria increased from 43.58% and 0.87% to 51.08% and 2.06%, respectively, after D-gal injection (p < 0.05, Fig. 7B). In addition, the relative abundance of Firmicutes and Actinobacteria decreased from 48.60% and 0.95% to 37.54% and 0.65% (p < 0.05, Fig. 7B), respectively. After L. plantarum Y44 and ascorbic acid administration, the relative abundance of Bacteroidetes, Firmicutes, and Proteobacteria in the HC group and the VC group was significantly mitigated compared to those of the DG group (p < 0.05, Fig. 7B). As shown in Fig. 7C, the Firmicutes/Bacteroidetes (F/B) ratio of the DG group decreased compared to that of the CK group (p < 0.05). L. plantarum Y44 and ascorbic acid administration significantly reversed D-gal injection-induced F/B ratio reduction in the HC and the VC group, respectively (p < 0.05, Fig. 7C).
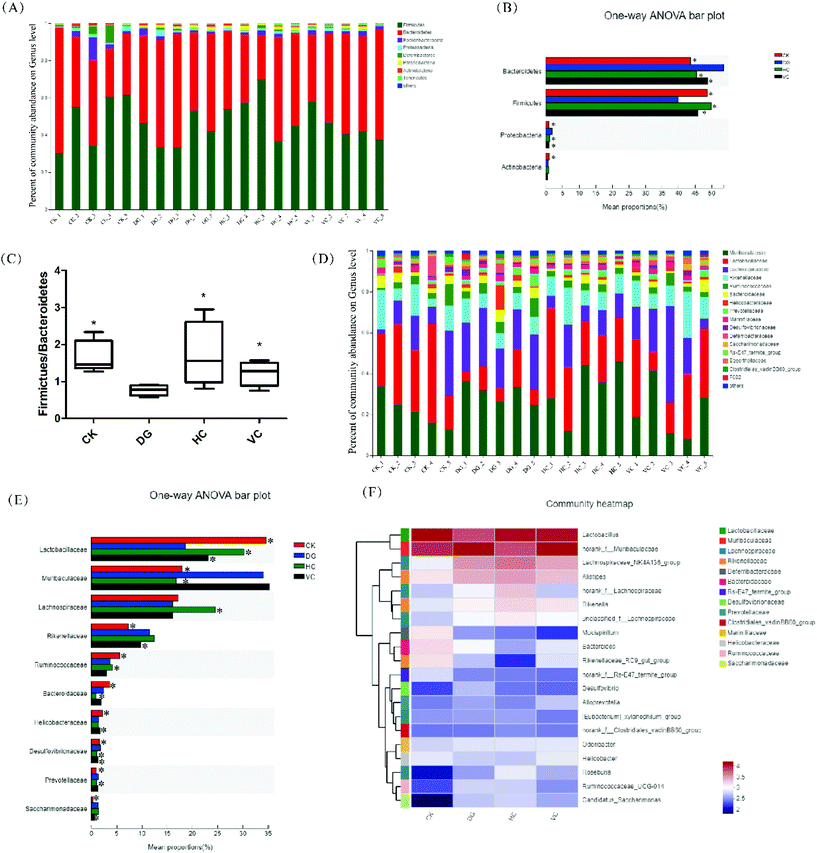 |
| Fig. 7 Effect of L. plantarum Y44 administration on the gut microbiota composition of the Balb/C mice. (A) Bar picture of the gut microbiota at the phylum level, (B) relative abundance of gut microbiota at the phylum level, (C) the ratio of Firmicutes to Bacteroidetes (F/B), (D) bar picture of the gut microbiota at the family level, (E) relative abundance of gut microbiota at the family level, and (F) heatmap of the gut microbiota at the genus level. The results are expressed as means ± SD (B, C and E). * indicates a significant difference compared to the DG group (p < 0.05, B, C and E). CK, Control group; DG, D-galactose group; HC, high concentration group; and VC, ascorbic acid group. | |
The difference in the gut microbiota composition at the family level is shown in Fig. 7D and E. The relative abundance of Lactobacillaceae in the DG group drastically dropped and the relative abundance of Muribaculaceae drastically elevated compared to those of the CK group (p < 0.05, Fig. 7E). After L. plantarum Y44 administration, the relative abundance of Lactobacillaceae and Muribaculaceae was remarkably restored, compared to those of the DG group (p < 0.05, Fig. 7D). However, ascorbic acid administration only restored the relative abundance of Lactobacillaceae in the VC group, and had no effect on the relative abundance of the Muribaculaceae (p > 0.05, Fig. 7E), compared to those of the DG group (p < 0.05, Fig. 7E). Additionally, the relative abundance of Lachnospiraceae in the HC group was higher than that of other groups (p < 0.05, Fig. 7E). L. plantarum Y44 administration could restore D-gal injection-induced Ruminococcaeae, Prevotellaceae, Desulfovbrionaceae variations (p < 0.05, Fig. 7E). However, ascorbic acid administration could restore D-gal injection-induced Rikenellaceae, Helicobacteraceae, Desulfovbrionaceae, and Sacchairmonadaceae variation (p < 0.05, Fig. 7E). In addition, at the genus level, the relative abundance of Desulfovibrio, Helicobacter, Bacteroides, Mucispirillum, and Odoribacter were various in all groups, as shown in Fig. 7F.
3.6 Effect of L. plantarum Y44 administration on the serum metabolomics profile of the D-gal-injected Balb/C mice
The serum metabolomics profile was analyzed by the UPLC-ESI-Triple TOF/MS method. The principal component analysis (PCA) and orthogonal partial least-squares discrimination analysis (OPLS-DA) were carried out to discern the differences of metabolite fingerprints in the serum of the Balb/C mice among CK, DG, HC, and VC groups. PCA score plots demonstrated that there was a separation trend in the HC and VC group with other groups (Fig. S2†). OPLS-DA score plots showed that samples exhibited good clustering compared within groups, and separation compared among groups (Fig. S3†). In all comparable groups, the R2Y were close to 1, and Q2Y were all more than 0.7, respectively, indicating that the quality of the OPLS-DA model was reliable. The metabolites with variable importance in projection (VIP) values >1 in the OPLS-DA model and p < 0.05 were regarded as differential metabolites. A total of 103 differential metabolites in the CK/DG comparison group, 155 differential metabolites in the HC/DG comparison group, and 177 differential metabolites in the VC/DG comparison group (Fig. S4†).
To further identify the possible biochemical pathways that were affected by D-gal injection, MetPA was performed to reveal the most relevant pathways. Pathways with the criterion of pathway impact >0.2, and p-value < 0.05 were selected as the potential target differential metabolic pathway. As shown in Fig. 8, the potential target metabolic pathway of the mice was glycerophospholipid metabolism. As shown in Table 1, levels of glycerophospholipid and derivatives, such as phosphatidyl ethanolamines (PE), phosphatidylcholine (PC), phosphatidyl serines (PS), lysophosphatidyl cholines (LysoPC) and lysophosphatidic acid (LysoPA) in serum of the mice varied in different experimental groups. The PE (22:5/24:1), PS (18:4/22:0), PC (20:5/22:6), LysoPC (20:0), and LysoPC (20:5) levels were elevated significantly (p < 0.05, Table 1), and the LysoPA level (0:0/16:0) was dropped significantly (p < 0.05, Table 1) after D-gal injection. L. plantarum Y44 administration significantly reversed the decline of PE (22:5/24:1), PS (18:0/20:0), PS (18:4/22:0), LysoPC (20:0) and LysoPC (20:0/0:0) levels (p < 0.05, Table 1) in the serum of the mice. However, neither L. plantarum Y44 nor ascorbic acid administration could relieve the D-gal injection-induced LysoPA level decline in the serum of the mice (Table 1).
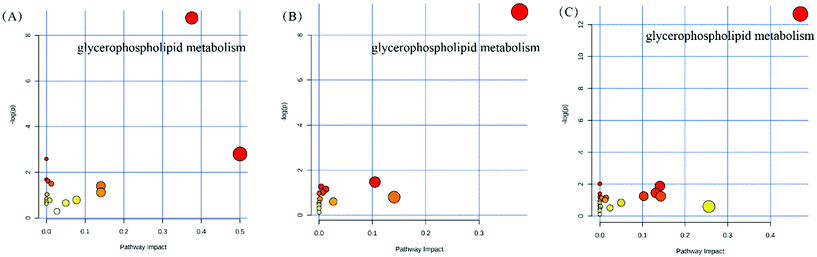 |
| Fig. 8 Metabolomics pathway analysis (MetPA) of differential metabolites in the comparison of the Balb/C mice in different groups. (A) DG/CK group, (B) HC/DG group, and (C) VC/DG group. CK, Control group; DG, D-galactose group; HC, high concentration group; and VC, ascorbic acid group. | |
Table 1 Differential metabolites of glycerophospholipid metabolism
Metabolite |
m/z |
Formula |
FC (DG/CK) |
FC (HC/DG) |
FC (HC/CK) |
FC (VC/DG) |
FC (VC/CK) |
Increased levels are indicated by arrows (↑); decreased levels are indicated by arrows (↓); * indicated variable importance in projection (VIP) values >1 in the OPLS-DA model and p < 0.05. |
PE (22:5/24:1) |
917.68 |
C51H90NO8P |
↑* |
↓* |
↓ |
↓ |
↑ |
PS (18:0/20:0) |
802.59 |
C44H86NO10P |
↑ |
↓* |
↓ |
↓ |
↑ |
PS (18:4/22:0) |
822.56 |
C46H82NO10P |
↑* |
↓* |
↓ |
↓ |
↑ |
PC (20:5/22:6) |
896.55 |
C50H78NO8P |
↑* |
↑ |
↑* |
↓ |
↑* |
LysoPC (20:0) |
552.40 |
C28H58NO7P |
↑* |
↓* |
↓ |
↓* |
↓ |
LysoPC (20:0/0:0) |
574.39 |
C28H58NO7P |
↑ |
↓* |
↓ |
↓* |
↓* |
LysoPC (20:5) |
586.31 |
C28H48NO7P |
↑* |
↑* |
↑* |
↑* |
↑* |
LysoPA (0:0/18:2) |
433.24 |
C21H39O7P |
↓ |
↓* |
↓* |
↓* |
↓* |
LysoPA (0:0/16:0) |
393.24 |
C19H39O7P |
↓* |
↓* |
↓* |
↓* |
↓* |
3.7 The correlation between gut microbiome, serum metabolome, liver antioxidant enzyme expressions/activities and tight junction proteins of the D-gal-injected Balb/C mice
The correlations of the gut microbiota, differential metabolites, antioxidant enzyme expressions/activities, and TJ proteins were determined using Spearman correlation analysis. As shown in Fig. 9A, the relative abundances of Proteobacteria, Muribaculaceae, and Prevotellaceae were negatively correlated with SOD and GPx activities, as well as Bacteroidetes’ relative abundance with GPx activity (p < 0.05). The relative abundance of Lactobacillacea and F/B ratio were positively correlated with SOD and GPx activities, as well as Firmicutes’ relative abundance with GPx activity (p < 0.05). HO-1 expression was positively correlated with the relative abundances of Lactobacillacea, Firmicutes, Actinobacteria, and F/B ratio and negatively correlated with relative abundances of Proteobacteria, Bacteroidetes, Prevotellaceae, Sacchairmonadaceae, Rikenellacea and Desulfovbrionaceae (p < 0.05). As shown in Fig. 9B, PS (18:4/22:0), PE (22:5/24:1), and LysoPC (20:0) levels were negatively correlated with SOD and GPx activities and HO-1 expression (p > 0.05). LysoPC (20:0/0:0) level was negatively correlated with SOD and GPx activities as well as Nrf-2, Occludin, and Claudin-1 expression (p < 0.05). As shown in Fig. 9C, PS (18:4/22:0) and LysoPC (20:0) levels were negatively correlated with F/B ratio and Lactobacillacea and Firmicutes’ relative abundances; and positively correlated with Proteobacteria, Muribaculaceae, Prevotellaceae and Bacteroidetes’ relative abundances (p < 0.05).
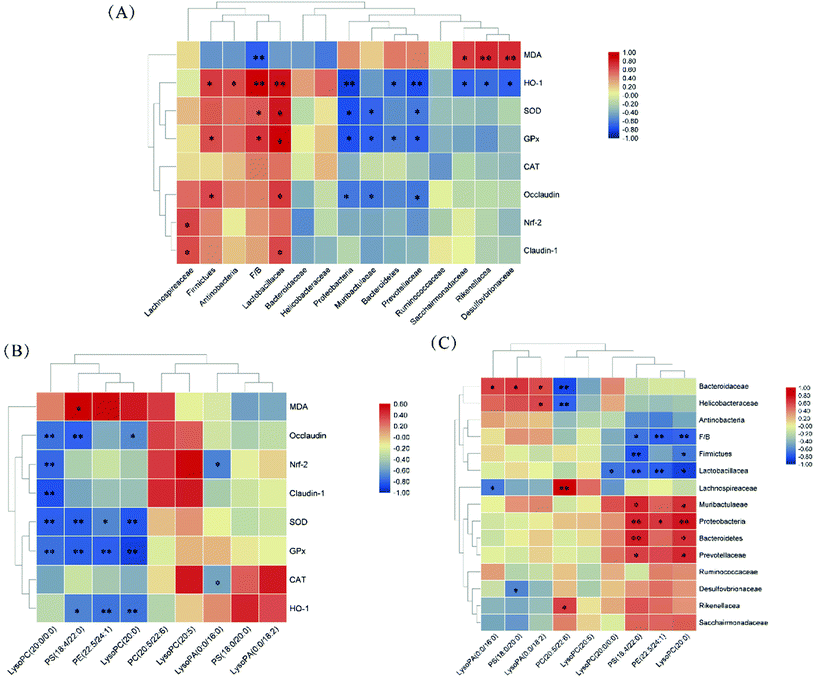 |
| Fig. 9 Correlation analysis (A) between gut microbiota and antioxidant enzyme expressions/activities and tight junction proteins, (B) between differential metabolites and antioxidant enzymes expressions/activities and tight junction proteins, (C) between gut microbiota and differential metabolites. * indicated p < 0.05 (two-tailed), ** indicated p < 0.01 (two-tailed). | |
4. Discussion
It was demonstrated that some lactobacilli strains improved the health of hosts after they colonized and propagated in the human gastrointestinal tract.10,26 In our previous study, L. plantarum Y44 exhibited abilities to scavenge free radicals and protect Caco-2 and HT-29 cell lines from oxidative stress in vitro.27–29 However, in vitro assays react directly and rapidly with ROS, which did not consider biological activity. Furthermore, L. plantarum Y44 is a live organism, whose antioxidant activity mechanism may be more complicated and differ from other antioxidants. In this study, the ameliorative effect and underlying mechanism of L. plantarum Y44 administration on the D-gal injection-induced oxidative stress in the Balb/C mice were examined in vivo.
D-Gal subcutaneous injection for mice can induce the ROS generation in the experimental animal cells, which caused injury to the cells and organs of the animals.30,31 It was reported that some lactobacilli strains exerted an antioxidative effect on mice exposed to D-gal.12,26 In this study, 6 weeks of continuous subcutaneous D-gal injection damaged the liver and colon, disturbed the colon microbiota balance, and led to glycerophospholipid metabolism disorder of Balb/C mice, while L. plantarum Y44 administration reversed the adverse physiological changes of the mice. The results were consistent with other reports.26,30–32
Previous studies suggested that gut microbiota composition generally revealed the health status of the host.15,33 In our study, D-gal injection led to the decline of the F/B ratio of the mice in the DG group. Similar changes induced by D-gal injection in aging mice were found by He et al.34 and Li et al.11 Obesity is associated with changes in the relative abundance of Bacteroidetes and Firmicutes.35 The reduction of Firmicutes proportion was found in the gut microbiota of Alzheimer's disease and Parkinson's disease patients, which were generally induced by oxidative stress.36 The administrations of L. plantarum Y44 and ascorbic acid reversed the changes in the gut microbiota through increasing Firmicutes’ relative abundance and decreasing Bacteroidetes’ relative abundance. In addition, L. plantarum Y44 administration reversed D-gal injection-induced decline of Lactobacillus’ relative abundance. Lactobacillus belongs to Lactobacillaceae, which could produce short-chain fatty acids (SCFAs), lower the intestinal pH, restrain pathogenic bacteria, and keep the balance of gut microbiota.21 The increased relative abundance of Lactobacillus in the HC group might attribute to survival and colonization of L. plantarum Y44 in the colon. The proportion of Lachnospiraceae and Ruminococcaceae was enriched in the HC group. Lachnospiraceae and Ruminococcaceae, belonging to Firmicutes, were SCFA-producing bacteria, which could prevent pathogen colonization and induce regulatory T cells against inflammation.16,37,38 Previous studies demonstrated that antioxidants alleviated oxidative stress by manipulating gut microbiota composition.11,12,39 In this study, a clear correlation between gut microbiota composition and antioxidant activity is shown in Fig. 9A, indicating that L. plantarum Y44 had the potential to alleviate oxidative stress by modulating the gut microbiota composition.
Desulfovibrionaceae and Proteobacteria were enriched in the mice of the DG group compared to those of other groups. Desulfovibrionaceae belongs to Proteobacteria, which was harmful to the immune system and associated with the pathogenesis of inflammatory bowel disease (IBD).40,41 The major outer membrane component of Proteobacteria and Desulfovibrionaceae are lipopolysaccharides (LPS), which leads to impairment of intestinal barrier function of the host.42–44Desulfovibrionaceae could interact with intestinal epithelial cells to weaken barrier function.44 The loss of colon integrity of the mice in DG group was attributed to the gut microbiota disturbance, such as Desulfovibrionaceae and Proteobacteria increase. Previous studies demonstrated that disordered gut microbiota composition undermined intestinal gut barrier function.45,46 TJ proteins can be connected to the actin skeleton and play an important role in the intestinal barrier function.47L. plantarum Y44 relieved TJ proteins impairment and maintained barrier function in Caco-2 cells under oxidative stress in our previous study.23 Claudin-1 and occludin are significant TJ proteins, and act as important factors for maintaining intestinal barrier function.48,49 In this study, Lactobacillaceae’ relative abundance in gut microbiota showed a significant correlation with claudin-1 and occludin expression (Fig. 9A), and L. plantarum Y44 administration enhanced intestinal barrier function by restoring gut microbiota composition. Some lactobacilli strains were reported to maintain intestinal barrier function through regulating the expression of TJ protein.45,50,51
Accumulating pieces of evidence have indicated that the gut microbiota acted as an additional metabolic organ to influence the host's metabolism.52–54 In addition, impaired intestinal barrier function lead to the pathogenesis of metabolic disorders.55,56 In this study, PE, PS and PC levels were correlated with the gut microbiota composition and TJ protein expressions (Fig. 9B and C). Glycerophospholipids are main lipid constituents of cell membranes and have a wide range of biological functions in cell proliferation, differentiation, and apoptosis.57–59 In the present study, glycerophospholipid metabolism disturbance was observed in the mice with subcutaneous D-gal injection, evidenced by elevated PE (22:5/24:1), PS (18:0/20:0), PS (18:4/22:0), LysoPC (20:0) and LysoPC (20:0/0:0) levels in the mice serum, which was in correspondence with other studies.60–62 Glycerophospholipid metabolism was identified as a significant metabolic pathway relevant to normal aging, and LysoPCs were regarded as the products of fatty acid oxidation.62–64 In addition, the PCs, PSs, and PEs are the main components of the cell membranes, which were involved in cell apoptosis and lipid signaling pathways.61–63 Liu et al.61 found that cell membrane biosynthesis was prevented by the oxidative stress, which resulted in elevated PE, PS and PC levels. In this study, the levels of the predominant glycerophospholipids in the serum of the mice were declined after L. plantarum Y44 administration, demonstrating that L. plantarum Y44 administration might alleviate fatty acid oxidation and facilitate cell membrane biosynthesis regularly. However, L. plantarum Y44 or ascorbic acid administration did not reverse the D-gal-injection-induced LysoPA level defect in this study.
The liver plays a role in maintaining metabolism and detoxification, which is easily exposed to ROS due to its high respiratory rate.30 In addition, gut microbiota disturbance and metabolic disorder could lead to liver impairment.26,65 In this study, D-gal injection facilitated liver injury evidenced by histopathology analysis, while L. plantarum Y44 administration reversed hepatic oxidative injury through activating the protein expression of the Nrf-2/Keap-1 pathway and promoting the activity of SOD and GPx. The Nrf-2/Keap-1 pathway was reported to participate in regulating a battery of genes against oxidative stress.66 Some lactobacilli strains activated the hepatic Nrf-2/Keap-1 pathway to promote liver antioxidant enzyme expression.11,67 In our previous study, L. plantarum Y44 protected Caco-2 cells from oxidative stress by activating the Nrf-2/Keap-1 pathway and elevating CAT expression.23 SOD, GPx, and CAT reflect antioxidant status universally. SOD is a necessary antioxidant enzyme, which could decompose superoxide through a disproportionation reaction.68 GPx specifically catalyze the reaction of glutathione with H2O2, thereby protecting the structural integrity of the cell membrane, and CAT also has the ability to directly remove peroxide.66 In this study L. plantarum Y44 administration activated the protein expression of hepatic Nrf-2/Keap-1 pathway and increased SOD, GPx and CAT activities to alleviate D-gal injection-induced oxidative stress in the mice.
5. Conclusion
In our previous studies, L. plantarum Y44 was clarified to scavenge free radicals and protect Caco-2 and HT-29 cell lines against oxidative stress in vitro. In this study, L. plantarum Y44 administration was found to ameliorate oxidative stress induced by D-gal. Furthermore, the underlying mechanism of L. plantarum Y44 administration on D-gal injection-induced oxidative stress in Balb/C mice should be attributed to the recovery of the disordered gut microbiota and intestinal barrier function of the Balb/C mice, and further influenced the glycerophospholipid metabolism and hepatic Nrf-2/Keap-1 pathway related protein expressions.
Author contribution
Yuan Gao: Conceptualization, methodology, validation, formal analysis, writing – original draft, and data curation; Yujun Liu: conceptualization, methodology, validation, formal analysis, and data curation; Fenglian Ma: conceptualization, and methodology; Mengying Sun: validation and formal analysis; Yinglong Song: validation and formal analysis; Dongxue Xu: validation and formal analysis; Guangqing Mu: conceptualization, project administration, funding acquisition, and supervision; Yanfeng Tuo: conceptualization, writing – review and editing, visualization, and supervision.
Conflicts of interest
The authors declare no competing financial interest.
Acknowledgements
This study was funded by the National Natural Science Foundation of China (No. 31671828).
References
- K. R. Elfahri, T. Vasiljevic, T. Yeager and O. N. Donkor, Anti-colon cancer and antioxidant activities of bovine skim milk fermented by selected Lactobacillus helveticus strains, J. Dairy Sci., 2015, 99(1), 31–40 CrossRef
.
- S. González-Manzano, A. M. González-Paramás, L. Delgado, S. Patianna, F. Surco-Laos, M. Dueñas and C. Santos-Buelga, Oxidative status of stressed Caenorhabditis elegans treated with epicatechin, J. Agric. Food Chem., 2012, 60(36), 8911–8916 CrossRef
.
- M. Valko, D. Leibfritz, J. Moncol, M. T. Cronin, M. Mazur and J. Telser, Free radicals and antioxidants in normal physiological functions and human disease, Int. J. Biochem. Cell Biol., 2007, 39(1), 44–84 CrossRef CAS PubMed
.
- T. Wei, Z. Xing, H. Wei, L. Chao, J. Wang and Y. Wang, Antioxidative effects in vivo and colonization of Lactobacillus plantarum MA2 in the murine intestinal tract, Appl. Microbiol. Biotechnol., 2016, 100(16), 7193–7202 CrossRef
.
- V. Mishra, C. Shah, N. Mokashe, R. Chavan, H. Yadav and J. Prajapati, Probiotics as potential antioxidants: a systematic review, J. Agric. Food Chem., 2015, 63(14), 3615–3626 CrossRef CAS PubMed
.
- Y. Wang, Y. Wu, Y. Wang, H. Xu, X. Mei, D. Yu, Y. Wang and W. Li, Antioxidant Properties of Probiotic Bacteria, Nutrients, 2017, 9(5), 521 CrossRef
.
- S.-H. Son, H.-L. Jeon, E. B. Jeon, N.-K. Lee, Y.-S. Park, D.-K. Kang and H.-D. Paik, Potential probiotic Lactobacillus plantarum Ln4 from kimchi: Evaluation of β-galactosidase and antioxidant activities, LWT–Food Sci. Technol., 2017, 85, 181–186 CrossRef CAS
.
- J. Xing, G. Wang, Z. Gu, X. Liu, Q. Zhang, J. Zhao, H. Zhang, Y. Q. Chen and W. Chen, Cellular model to assess the antioxidant activity of lactobacilli, RSC Adv., 2015, 5(47), 37626–37634 RSC
.
- J. Xing, G. Wang, Q. Zhang, X. Liu, B. Yin, D. Fang, J. Zhao, H. Zhang, Y. Q. Chen and W. Chen, Determining antioxidant activities of lactobacilli by cellular antioxidant assay in mammal cells, J. Funct. Foods, 2015, 19, 554–562 CrossRef CAS
.
- W. Tang, Z. Xing, W. Hu, C. Li, J. Wang and Y. Wang, Antioxidative effects in vivo and colonization of Lactobacillus plantarum MA2 in the murine intestinal tract, Appl. Microbiol. Biotechnol., 2016, 100(16), 7193–7202 CrossRef CAS
.
- B. Li, S. E. Evivie, J. Lu, Y. Jiao, C. Wang, Z. Li, F. Liu and G. Huo, Lactobacillus helveticus KLDS1. 8701 alleviates D-galactose-induced aging by regulating Nrf-2 and gut microbiota in mice, Food Funct., 2018, 9(12), 6586–6598 RSC
.
- J. Zhao, F. Tian, S. Yan, Q. Zhai, H. Zhang and W. Chen, Lactobacillus plantarum CCFM10 alleviating oxidative stress and restoring the gut microbiota in d-galactose-induced aging mice, Food Funct., 2018, 9(12), 917–924 RSC
.
- E. B.-M. Daliri, S. Wei, D. H. Oh and B. H. Lee, The human microbiome and metabolomics: current concepts and applications, Crit. Rev. Food Sci. Nutr., 2017, 57(16), 3565–3576 CrossRef CAS
.
- S. Lamichhane, P. Sen, A. M. Dickens, M. Orešič and H. C. Bertram, Gut metabolome meets microbiome: A methodological perspective to understand the relationship between host and microbe, Methods, 2018, 149, 3–12 CrossRef CAS
.
- Y. Shao, D. Huo, Q. Peng, Y. Pan, S. Jiang, B. Liu and J. Zhang, Lactobacillus plantarum HNU082-derived improvements in the intestinal microbiome prevent the development of hyperlipidaemia, Food Funct., 2017, 8(12), 4508–4516 RSC
.
- Y. Li, Z. Xie, T. Gao, L. Li, Y. Chen, D. Xiao, W. Liu, B. Zou, B. Lu, X. Tian, B. Han, Y. Guo, S. Zhang, L. Lin, M. Wang, P. Li and Q. Liao, A holistic view of gallic acid-induced attenuation in colitis based on microbiome-metabolomics analysis, Food Funct., 2019, 10(7), 4046–4061 RSC
.
- M. Chen, D. Xiao, W. Liu, Y. Song, B. Zou, L. Li, P. Li, Y. Cai, Q. Liao and Z. Xie, Intake of Ganoderma lucidum polysaccharides reverses the disturbed gut microbiota and metabolism in type 2 diabetic rats, Int. J. Biol. Macromol., 2020, 155, 890–902 CrossRef CAS
.
- Y. Li, P. Wang, J. Yin, S. Jin, W. Su, J. Tian, T. Li and K. Yao, Effects of ornithine α-ketoglutarate on growth performance and gut microbiota in a chronic oxidative stress pig model induced by D-galactose, Food Funct., 2020, 472–482 RSC
.
- F. Zhao, L. Gao, X. Qin, G. Du and Y. Zhou, The intervention effect of licorice in d-galactose induced aging rats by regulating the taurine metabolic pathway, Food Funct., 2018, 9(9), 4814–4821 RSC
.
- S. Mei, X. Song, Y. Wang, J. Wang, S. Su, J. Zhu and Y. Geng, Studies on Protection of Astaxanthin from Oxidative Damage Induced by H2O2 in RAW 264.7 Cells Based on 1H NMR Metabolomics, J. Agric. Food Chem., 2019, 67(49), 13568–13576 CrossRef CAS
.
- Y.-Y. Hor, L.-C. Lew, M. H. Jaafar, A. S.-Y. Lau, J.-S. Ong, T. Kato, Y. Nakanishi, G. Azzam, A. Azlan and H. Ohno, Lactobacillus sp. improved microbiota and metabolite profiles of aging rats, Pharmacol. Res., 2019, 146, 104312 CrossRef PubMed
.
- M. Wu, Y. Jiang, M. Liu, Y. Shang and J. An, Amino-PAHs activated Nrf2/ARE anti-oxidative defense system and promoted inflammatory responses: the regulation of PI3 K/Akt pathway, Toxicol. Res., 2018, 7(3), 465–472 CrossRef CAS PubMed
.
- Q. Wang, L. Lv, H. Jiang, K. Wang, R. Yan, Y. Li, J. Ye, J. Wu, Q. Wang and X. Bian, Lactobacillus helveticus R0052 alleviates liver injury by modulating gut microbiome and metabolome in d-galactosamine-treated rats, Appl. Microbiol. Biotechnol., 2019, 103(23–24), 9673–9686 CrossRef CAS
.
- L. Gonzalez-Mariscal, A. Betanzos, P. Nava and B. E. Jaramillo, Tight junction proteins, Prog. Biophys. Mol. Biol., 2003, 81(1), 1–44 CrossRef CAS
.
- E. A. Runkle and D. Mu, Tight junction proteins: from barrier to tumorigenesis, Cancer Lett., 2013, 337(1), 41–48 CrossRef CAS
.
- D. Ren, C. Li, Y. Qin, R. Yin, S. Du, F. Ye, C. Liu, H. Liu, M. Wang and Y. Li, In vitro evaluation of the probiotic and functional potential of Lactobacillus strains isolated from fermented food and human intestine, Anaerobe, 2014, 30, 1–10 CrossRef CAS
.
- G. Mu, Y. Gao, Y. Tuo, H. Li, Y. Zhang, F. Qian and S. Jiang, Assessing and comparing antioxidant activities of lactobacilli strains by using different chemical and cellular antioxidant methods, J. Dairy Sci., 2018, 101(12), 10792–10806 CrossRef CAS
.
- G. Mu, H. Li, Y. Tuo, Y. Gao and Y. Zhang, Antioxidative effect of Lactobacillus plantarum Y44 on 2, 2′-azobis (2-methylpropionamidine) dihydrochloride (ABAP)-damaged Caco-2 cells, J. Dairy Sci., 2019, 102(8), 6863–6875 CrossRef CAS PubMed
.
- Y. Gao, Y. Liu, M. Sun, H. Zhang, G. Mu and Y. Tuo, Physiological function analysis of Lactobacillus plantarum Y44 based on genotypic and phenotypic characteristics, J. Dairy Sci., 2020, 103(7), 5916–5930 CrossRef CAS
.
- S.-C. Ho, J.-H. Liu and R.-Y. Wu, Establishment of the mimetic aging effect in mice caused by D-galactose, Biogerontology, 2003, 4(1), 15–18 CrossRef CAS
.
- Z. Qu, J. Zhang, H. Yang, L. Huo and W. Gao, Protective effect of tetrahydropalmatine against D-galactose induced memory impairment in rat, Physiol. Behav., 2015, 154(11), 114–125 Search PubMed
.
- C. Duan, Y. Zhao, C. Huang, Z. Zhao, L. Gao, C. Niu, C. Wang, X. Liu, C. Zhang and S. Li, Hepatoprotective effects of Lactobacillus plantarum, C88 on LPS/D-GalN–induced acute liver injury in mice, J. Funct. Foods, 2018, 43, 146–153 CrossRef CAS
.
- L. Qu, J. Ren, L. Huang, B. Pang, X. Liu, X. Liu, B. Li and Y. Shan, Antidiabetic Effects of Lactobacillus casei Fermented Yogurt through Reshaping Gut Microbiota Structure in Type 2 Diabetic Rats, J. Agric. Food Chem., 2018, 66(48), 12696–12705 CrossRef CAS PubMed
.
- S. He, Z. Zhang, H. Sun, Y. Zhu, X. Cao, Y. Ye, J. Wang and Y. Cao, Potential effects of rapeseed peptide Maillard reaction products on aging-related disorder attenuation and gut microbiota modulation in d-galactose induced aging mice, Food Funct., 2019, 10(7), 4291–4303 RSC
.
- P. J. Turnbaugh, R. E. Ley, M. A. Mahowald, V. Magrini, E. R. Mardis and J. I. Gordon, An obesity-associated gut microbiome with increased capacity for energy harvest, Nature, 2006, 444(7122), 1027 CrossRef PubMed
.
- S. Manoharan, G. J. Guillemin, R. S. Abiramasundari, M. M. Essa, M. Akbar and M. D. Akbar, The role of reactive oxygen species in the pathogenesis of Alzheimer’s disease, Parkinson’s disease, and Huntington’s disease: a mini review, Oxid. Med. Cell. Longevity, 2016, 2016, 8590578 Search PubMed
.
- M. F. Neurath, Host–microbiota interactions in inflammatory bowel disease, Nat. Rev. Gastroenterol. Hepatol., 2020, 17(2), 76–77 CrossRef
.
- Q. Shang, X. Shan, C. Cai, J. Hao, G. Li and G. Yu, Dietary fucoidan modulates the gut microbiota in mice by increasing the abundance of Lactobacillus and Ruminococcaceae, Food Funct., 2016, 7(7), 3224–3232 RSC
.
- Y. Qiao, J. Sun, Y. Ding, G. Le and Y. Shi, Alterations of the gut microbiota in high-fat diet mice is strongly linked to oxidative stress, Appl. Microbiol. Biotechnol., 2013, 97(4), 1689–1697 CrossRef CAS
.
- I. Mukhopadhya, R. Hansen, E. M. El-Omar and G. L. Hold, IBD—what role do Proteobacteria play?, Nat. Rev. Gastroenterol. Hepatol., 2012, 9(4), 219 CrossRef CAS
.
- F. Rowan, N. G. Docherty, M. Murphy, B. Murphy, J. C. Coffey and P. R. O‘Connell, Desulfovibrio bacterial species are increased in ulcerative colitis, Dis. Colon Rectum, 2010, 53(11), 1530–1536 CrossRef
.
- J. P. Pearson and I. A. Brownlee, The interaction of large bowel microflora with the colonic mucus barrier, Int. J. Inflammation, 2010, 2010, 321426 Search PubMed
.
- K. Madsen, A. Cornish, P. Soper, C. McKaigney, H. Jijon, C. Yachimec, J. Doyle, L. Jewell and C. De Simone, Probiotic bacteria enhance murine and human intestinal epithelial barrier function, Gastroenterology, 2001, 121(3), 580–591 CrossRef CAS PubMed
.
- V. R. Figliuolo, R. Coutinho-Silva and C. M. L. M. Coutinho, Contribution of sulfate-reducing bacteria to homeostasis disruption during intestinal inflammation, Life Sci., 2018, 215, 145–151 CrossRef CAS
.
- J. Wang, H. Ji, S. Wang, H. Liu, W. Zhang, D. Zhang and Y. Wang, Probiotic Lactobacillus plantarum Promotes Intestinal Barrier Function by Strengthening the Epithelium and Modulating Gut Microbiota, Front. Microbiol., 2018, 9, 1953 CrossRef
.
- P. D. Cani, Crosstalk between the gut microbiota and the endocannabinoid system: impact on the gut barrier function and the adipose tissue, Clin. Microbiol. Infect., 2012, 18, 50–53 CrossRef CAS PubMed
.
- J. Karczewski,
et al., Regulation of human epithelial tight junction proteins by Lactobacillus plantarum in vivo and protective effects on the epithelial barrier, Am. J. Physiol.: Gastrointest. Liver Physiol., 2010, 298(6), G851–G859 CrossRef CAS PubMed
.
- M. Furuse, M. Hata, K. Furuse, Y. Yoshida, A. Haratake, Y. Sugitani, T. Noda, A. Kubo and S. Tsukita, Claudin-based tight junctions are crucial for the mammalian epidermal barrier a lesson from claudin-1–deficient mice, J. Cell Biol., 2002, 156(6), 1099–1111 CrossRef CAS PubMed
.
- G. J. Feldman, J. M. Mullin and M. P. Ryan, Occludin: structure, function and regulation, Adv. Drug Delivery Rev., 2005, 57(6), 883–917 CrossRef CAS PubMed
.
- Y. Qiu, Z. Jiang, S. Hu, L. Wang, X. Ma and X. Yang, Lactobacillus plantarum Enhanced IL-22 Production in Natural Killer (NK) Cells That Protect the Integrity of Intestinal Epithelial Cell Barrier Damaged by Enterotoxigenic Escherichia coli, Int. J. Mol. Sci., 2017, 18(11), 2409 CrossRef
.
- N. Valeur, P. Engel, N. Carbajal, E. Connolly and K. Ladefoged, Colonization and Immunomodulation by Lactobacillus reuteri ATCC 55730 in the Human Gastrointestinal Tract, Appl. Environ. Microbiol., 2004, 70(2), 1176–1181 CrossRef CAS
.
- F. Bäckhed, R. E. Ley, J. L. Sonnenburg, D. A. Peterson and J. I. Gordon, Host-bacterial mutualism in the human intestine, Science, 2005, 307(5717), 1915–1920 CrossRef
.
- A. Tripathi, J. Debelius, D. A. Brenner, M. Karin, R. Loomba, B. Schnabl and R. Knight, The gut–liver axis and the intersection with the microbiome, Nat. Rev. Gastroenterol. Hepatol., 2018, 15(7), 397–411 CrossRef CAS
.
- Y. Zeng, H. Zhang, R. Tsao and Y. Mine, Lactobacillus pentosus S-PT84 Prevents Low-Grade Chronic Inflammation-Associated Metabolic Disorders in a Lipopolysaccharide and High-Fat Diet C57/BL6J Mouse Model, J. Agric. Food Chem., 2020, 4374–4386 CrossRef CAS PubMed
.
- B. G. Postal, S. Ghezzal, D. Aguanno, S. André, K. Garbin, L. Genser, E. Brot-Laroche, C. Poitou, H. Soula and A. Leturque, AhR activation defends gut barrier integrity against damage occurring in obesity, Mol. Metab., 2020, 101007 CrossRef CAS
.
- H. Liang, Y. Ma, R. Li and A. Ma, Effect of Aplysin on Intestinal Barrier and Lipid Metabolism of Rats Exposed by Ethanol and Iron, FASEB J., 2017, 31(1_supplement), lb409 Search PubMed
.
- L. Zong, J. Xing, S. Liu, Z. Liu and F. Song, Cell metabolomics reveals the neurotoxicity mechanism of cadmium in PC12 cells, Ecotoxicol. Environ. Saf., 2018, 147, 26–33 CrossRef CAS PubMed
.
- G. Van Meer, D. R. Voelker and G. W. Feigenson, Membrane lipids: where they are and how they behave, Nat. Rev. Mol. Cell Biol., 2008, 9(2), 112–124 CrossRef CAS PubMed
.
- X. Wang, Y. Xu, X. Song, Q. Jia, X. Zhang, Y. Qian and J. Qiu, Analysis of glycerophospholipid metabolism after exposure to PCB153 in PC12 cells through targeted lipidomics by UHPLC-MS/MS, Ecotoxicol. Environ. Saf., 2019, 169, 120–127 CrossRef CAS
.
- D. Wang, Q. Wang, R. Chen, S. Yang, Z. Li and Y. Feng, Exploring the effects of Gastrodia elata Blume on the treatment of cerebral ischemia-reperfusion injury using UPLC-Q/TOF-MS-based plasma metabolomics, Food Funct., 2019, 10(11), 7204–7215 RSC
.
- W. Liu, F. Jin, D. Gao, L. Song, C. Ding and H. Liu, Metabolomics analysis reveals aminoquinazolin derivative 9d-induced oxidative stress and cell cycle arrest in A549 cells, RSC Adv., 2017, 7(22), 13149–13158 RSC
.
- Q. Xie, H. Zhao, N. Li, L. Su, X. Xu and Z. Hong, Protective effects of timosaponin BII on oxidative stress damage in PC12 cells based on metabolomics, Biomed. Chromatogr., 2018, 32(10), e4321 CrossRef
.
- S. Zhang, J. Zhuang, G. Yue, Y. Wang, M. Liu, B. Zhang, Z. Du and Q. Ma, Lipidomics to investigate the pharmacologic mechanisms of ginkgo folium in the hyperuricemic rat model, J. Chromatogr. B, 2017, 1060, 407–415 CrossRef CAS PubMed
.
- V. Frisardi, F. Panza, D. Seripa, T. Farooqui and A. A. Farooqui, Glycerophospholipids and glycerophospholipid-derived lipid mediators: a complex meshwork in Alzheimer's disease pathology, Prog. Lipid Res., 2011, 50(4), 313–330 CrossRef CAS PubMed
.
- R. Xu, Aruhan, L. Xiu, S. Sheng, Y. Liang, H. Zhang, Y. Liu, H. Tong, R. Du and X. Wang, Exopolysaccharides from Lactobacillus buchneri TCP016 Attenuate LPS- and d-GalN-Induced Liver Injury by Modulating the Gut Microbiota, J. Agric. Food Chem., 2019, 67(42), 11627–11637 CrossRef CAS PubMed
.
- D. Gao, Z. Gao and G. Zhu, Antioxidant effects of Lactobacillus plantarum via activation of transcription factor Nrf2, Food Funct., 2013, 4(6), 982–989 RSC
.
- X. Lin, Y. Xia, G. Wang, Z. Xiong, H. Zhang, F. Lai and L. Ai, Lactobacillus plantarum AR501 Alleviates the Oxidative Stress of D–Galactose–Induced Aging Mice Liver by Upregulation of Nrf2–Mediated Antioxidant Enzyme Expression, J. Food Sci., 2018, 83(7), 1990–1998 CrossRef CAS
.
- Y. Li, Y. Guo, M. Xi, P. Yang, X. Zhou, S. Yin, C. Hai, J. Li and X. Qin, Saponins from Aralia taibaiensis attenuate D-galactose-induced aging in rats by activating FOXO3a and Nrf2 pathways, Oxid. Med. Cell. Longevity, 2014, 2014, 320513 Search PubMed
.
Footnote |
† Electronic supplementary information (ESI) available. See DOI: 10.1039/d0fo02794d |
|
This journal is © The Royal Society of Chemistry 2021 |