DOI:
10.1039/D0FO02689A
(Paper)
Food Funct., 2021,
12, 215-229
The galloyl moiety enhances the inhibitory activity of catechins and theaflavins against α-glucosidase by increasing the polyphenol–enzyme binding interactions
Received
14th October 2020
, Accepted 23rd November 2020
First published on 24th November 2020
Abstract
The inhibition properties of 10 tea polyphenols against α-glucosidase were studied through inhibition assay, inhibition kinetics, fluorescence quenching and molecular docking. It was found that the inhibitory activity of polyphenols with a 3 and/or 3′ galloyl moiety (GM) was much higher than that without a GM. The GM could enter into the active site of α-glucosidase and bind with the catalytic amino acid residues through hydrogen bonding and π-conjugation, thus playing an important role in the competitive inhibition of catechins and theaflavins. The positive linear correlations among the constants characterizing the inhibitory activity and binding affinity of tea polyphenols to α-glucosidase indicate that enzyme inhibition by polyphenols is caused by the binding interactions between them, and that the combination of the characterization methods for polyphenol–glucosidase binding is reasonable. In addition, the in vivo hypoglycemic effects of galloylated polyphenols suggest that the GM may be considered as a pharmaceutical fragment for the alleviation of type II diabetes symptoms through α-glucosidase inhibition.
1. Introduction
Postprandial hyperglycemia has been reported to be highly related with the development of glucose metabolism disorders, such as type II diabetes.1 From the view of modern nutriology, retarding the increase in the postprandial blood sugar level is beneficial to human health, as it alleviates the burden of pancreatic islet B cells that secrete insulin to promote blood glucose to enter into functional tissues, such as brain, liver and muscle.2 Carbohydrates are the main source of exogenous sugars in human beings, of which starchy foods account for the majority of energy intake. In vivo, starch is initially hydrolyzed by salivary (minority in the mouth cavity) and pancreatic (majority in the small intestine) α-amylase, producing reducing sugars, such as maltose, maltotriose, maltooligosacchairdes, etc., followed by digestion to glucose under the action of pancreatic α-glucosidase in the small intestine.3 Therefore, α-amylase and α-glucosidase are two essential enzymes that regulate starch digestion, and the inhibition of both or respective enzymes has been suggested as an approach for retarding starch digestion and delaying the increase in the blood sugar level after a meal.4,5
Usually, acarbose and voglibose are prescribed to diabetic patients as inhibitors of carbohydrate-hydrolyzing enzymes, as they are the analogs of α-1,4- glycosidic bond substrates,6 although there are some adverse side-effects of long-term administration of the two pharmaceuticals, such as abdominal distension, bellyache and diarrhea.7 Therefore, it is necessary to explore natural enzyme inhibitors that have relatively fewer side-effects. Recently, some dietary polyphenols have been reported to show inhibitory effects on α-amylase and/or α-glucosidase to some extent, for example green coffee bean polyphenols,8 pomegranate proanthocyanidins,9 tea polyphenols,10etc. The inhibitory activity of serial polyphenolic compounds that have similar skeletons is highly dependent on their molecular structures, especially on certain moieties. For instance, the caffeoyl moiety is essential for the inhibitory activity of the chlorogenic acid family (caffeoylquinic acids and dicaffeoylquinic acids) against α-amylase and α-glucosidase,11,12 because the adjacent hydroxyl groups of caffeoyl can form hydrogen bonds with the key amino acid residues at the enzyme active sites, and the benzene ring of this moiety can conjugate (π–π stacking) with the aromatic rings of the enzyme fluorescent residues (e.g., Tyr and Trp).13,14 Besides, as for tea polyphenols that are normally composed of catechins and theaflavins, the 3 and/or 3′ galloyl moiety (GM) at ring C has been reported to enhance the association of catechins and theaflavins with porcine pancreatic α-amylase, thus increasing the inhibitory activity of both kinds of polyphenols against the enzyme.15,16 The role of the GM in the binding of tea polyphenols with α-amylase is also attributed to hydrogen bonding (between –OH and essential amino acids at the enzyme active site) and π-stacking (between benzene and the aromatic residues of the enzyme).17 Although some tea polyphenols have already been shown to exert the inhibitory activity against α-glucosidase,18–20 a detailed phenolic structure–inhibitory effect relationship, which is suggested to involve a series of catechins and theaflavins, needs to be further investigated.
It has been accepted that the inhibition of α-glucosidase by polyphenols results from the binding interactions between them which have been studied by using substrate depletion assay, inhibition kinetics, fluorescence quenching, molecular docking, etc.2,21 Using these methods, some constants that are able to suggest the binding affinity of a polyphenol to the enzyme can be obtained, such as inhibition constant, fluorescence quenching constant, binding energy, etc.2,21 However, how these constants are combined comprehensively to characterize polyphenol–glucosidase binding interactions needs to be illustrated by taking a series of polyphenols with comparable molecular structures into account. Therefore, in this study 10 tea polyphenols including 6 catechins and 4 theaflavins (structures are shown in Fig. 1) were selected to characterize the binding and inhibitory activity of these compounds against α-glucosidase in vitro by combining inhibitory activity assay, detailed inhibition kinetics, fluorescence quenching and molecular docking, through which the contribution of GM to enzyme inhibition by tea polyphenols is illustrated, and the mode of binding interactions between them that cause the inhibition is elucidated, as well as the reasonability of the combination of the above methods. In addition, the hypoglycemic effects of tea polyphenols which are attributed to α-glucosidase inhibition in vivo were also studied to evaluate the galloylated compounds as alternatives to commercial pharmaceuticals for the alleviation of hyperglycemia symptoms.
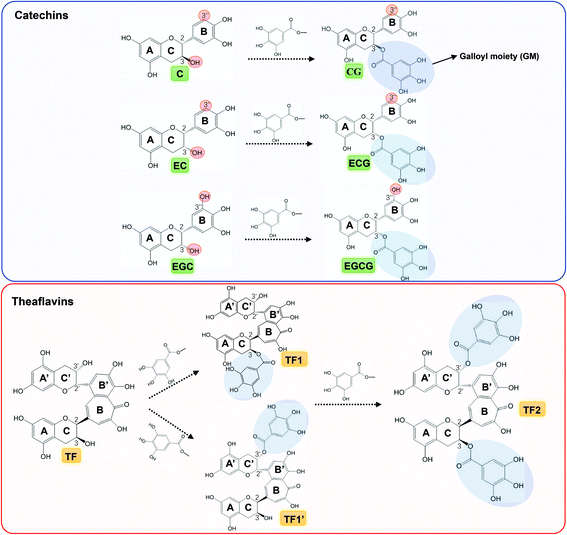 |
| Fig. 1 Molecular structures of catechins (green labelled) and theaflavins (yellow labelled), in which CG, ECG and EGCG are the galloylated forms of C, EC and EGC, respectively, and TF1/TF1′ and TF2 are the galloylated and digalloylated forms of TF, respectively. Besides, some groups/moieties related with the inhibitory activity of tea polyphenols are also labelled, such as 3-OH at ring C, 3′′-OH at ring B, 3 and/or 3′-galloyl moiety (GM) at ring C and/or C′. | |
2. Materials and methods
2.1 Materials and reagents
Ten pure tea polyphenols, including (+)-catechin (C), (−)-epicatechin (EC), (−)-epigallocatechin (EGC), (+)-catechin-3-gallate (CG), (−)-epicatechin-3-gallate (ECG), (−)-epigallocatechin-3-gallate (EGCG), theaflavin (TF), theaflavin-3-gallate (TF1), theaflavin-3′-gallate (TF1′) and theaflavin-3,3′-digallate (TF2) were purchased from Chengdu Biopurify Phytochemicals Ltd Co. (Chengdu, China). 1,3,6-Tri-O-galloyl-β-D-glucose (3G) was purchased from Shanghai Yuanye Biotech. Co. (Shanghai, China). α-Glucosidase (EC 3.2.1.20) from Saccharomyce cerevisiae and phosphate buffered saline tablet (P4417) were obtained from Sigma-Aldrich Co. (St Louis, US). p-Nitrophenyl-α-D-glucopyranoside (pNPG) was purchased from Yuanye Biotech. Co. (Shanghai, China). Other chemicals in this study were of analytical grade.
2.2 Inhibitory activity
The inhibitory effects of 10 tea polyphenols against α-glucosidase were initially characterized at the same phenolic concentration as follows.22 In detail, 4 U mL−1 α-glucosidase and 10 mM pNPG were prepared in PBS buffer. Ten tea polyphenols (1 mg mL−1) were dissolved in 20% (v/v) DMSO in PBS (the final DMSO concentration did not affect the enzyme activity significantly), respectively. Then, 200 μL of each tea polyphenol and 200 μL of α-glucosidase were incubated at 4 °C for 15 min, followed by the addition of 4 mL of PBS buffer. After that, 500 μL of 10 mM pNPG substrate solution was added to initiate the reaction at 37 °C. At an interval of 3 min during the initial 9 min, 500 μL of reaction solution was withdrawn into a 1.5 mL centrifuge tube containing 500 μL of 0.3 M Na2CO3 to stop the reaction. The respective absorbance values of the samples were determined using a UV-Vis spectrophotometer at 405 nm. The initial reaction velocity was obtained from the slope of the plot of pNP equivalent (Δ absorbance value) against the reaction time (min), and the inhibition (%) was calculated using the following eqn (1). | 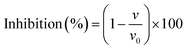 | (1) |
where v and v0 are the initial digestion velocities in the presence and absence of tea polyphenols, respectively.
To further characterize the inhibitory activity of tea polyphenols, the half-maximal inhibitory concentration (IC50) values were obtained from the enzyme inhibition (%) at a series of polyphenol concentrations using the following eqn (2).16,23
| 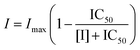 | (2) |
where [I] is the polyphenol concentration,
I is the enzyme inhibition (%) at the corresponding polyphenol concentration, and
Imax is the maximum percentage of inhibition.
2.3 Inhibition kinetics
To obtain the inhibition types and inhibition constants, the kinetics of polyphenols (eight tea polyphenols that were shown to exhibit a relatively high inhibitory activity, as well as 3G) involving the Dixon and Cornish-Bowden plots was studied at a series of polyphenol (inhibitor) and pNPG (substrate) concentrations using the following equations (3, Dixon equation; and4, Cornish-Bowden equation).24,25 | 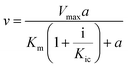 | (3) |
| 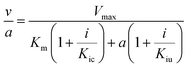 | (4) |
where a is the pNPG concentration; i is the polyphenol concentration; v is the initial reaction velocity at the corresponding pNPG and polyphenol concentrations, which is obtained by using the above introduced method; Vmax is the maximum initial reaction velocity; Km is the Michaelis constant; Kic is the competitive inhibition constant; and Kiu is the uncompetitive inhibition constant.
2.4 Fluorescence quenching
The fluorescence spectra of α-glucosidase in the absence and presence of tea polyphenols were obtained using a spectrofluorometer as follows.26 In detail, the ten tea polyphenols and α-glucosidase were dissolved in PBS buffer. Then, 30 μL of tea polyphenols with various concentrations were mixed with 400 μL of 50 U mL−1 α-glucosidase, followed by incubation at 4 °C for 15 min. Each polyphenol solution mixed with PBS was used as the control. After that, the fluorescence spectra were recorded at an excitation wavelength (λex) of 280 nm and at an emission wavelength in the range from 300 to 500 nm. Both the slit widths were set at 10 nm. The fluorescence quenching constant, KFQ, was calculated using the Stern–Volmer eqn (5) as follows.27 | 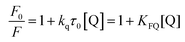 | (5) |
where F0 and F are the maximum fluorescence intensities in the absence and presence of tea polyphenols, respectively; kq is the bimolecular quenching constant; τ0 is the lifetime of the fluorophore (for α-glucosidase, the value is 10−8 s); and [Q] is the polyphenol concentration.
On the other hand, there may be some positive deviations for the Stern–Volmer equation observed for some quenchers, causing the fitted plot concave towards the y axis. For this case, the modified exponential form of the Stern–Volmer eqn (6) is obtained as follows.27
|  | (6) |
2.5 Molecular docking
The molecular docking software Sybyl 2.0 was used to predict the binding force and sites of polyphenols (ten tea polyphenols and 3G) with α-glucosidase as follows.28 The crystal structure of α-glucosidase was obtained from the Protein Data Bank (PDB ID: 3A4A). Before docking, the enzyme was prepared by minimizing energy, removing water, fixing side chains and adding hydrogen atoms and Gasteiger–Huckel charges, and the polyphenols were sketched and energy-minimized. The docking was performed using Surflex-Dock mode, and the binding energy (Eb) was calculated using eqn (7) as follows. | Eb = RT loge(10−pkd) | (7) |
where pkd is the affinity score according to the Surflex scoring function, and RT is 0.59 kcal mol−1.
2.6 Sucrose-loading test in vivo
The sucrose-loading test was performed to indicate the inhibitory effects of tea polyphenols against α-glucosidase in vivo.29 Normal six-week-old C57BL/6 mice (male, 20–22 g) were fasted for 10 h and divided into ten groups. The fasting blood glucose levels were tested at 0 min before the experiment. Sucrose was prepared in sterile water and was administered at a dose of 1 g per kg body weight. The tea polyphenols (EGCG, EGC, ECG, EC, TF2, TF1 and TF) were initially administered via a stomach tube at a dose of 50 mg per kg body weight, and acarbose (ACA) at a similar dose was considered the positive control. Sucrose was administered to the mice after 10 minutes. The blood samples collected from the tail vein were determined at 15, 30, 60, 90 and 120 min using a blood glucometer (OneTouch). The trapezoid calculation method was applied to calculate the area under the curve (AUC). All animal experiments were approved by the Guidelines for the Care and Use of Laboratory Animals: 8th Edition, ISBN-10: 0-309-15396-4, and the experiments were approved by the Animal Ethics Committee of Xi'an Jiaotong University.
2.7 Statistical analysis
One-way analysis of variance (ANOVA) followed by Tukey's test (GraphPad Prism 6) was used to analyze the significant difference between the constants. When P < 0.05, the data were considered to be statistically significant and thus they were marked with different superscripts.
3. Results
3.1 Inhibitory activity
The inhibitory activities of the 10 tea polyphenols against α-glucosidase were initially described by the inhibition ratio (%) per molar concentration of polyphenols. As shown in Fig. 2A, EC and EGC hardly inhibited the enzyme activity, while TF2 showed the strongest inhibitory effect among the studied polyphenols. To further describe the inhibitory activity, the IC50 values were obtained based on the inhibition ratios at a series of polyphenol concentrations. It was found that even at a relatively high concentration (1 mg mL−1), the inhibition ratios of EC and EGC were very low (<20%), while at the same concentration, the inhibition ratios of the other tea polyphenols were nearly 100% (Fig. 2B and C). Therefore, the IC50 values of EC and EGC were not able to be fitted using eqn (2). The IC50 values followed the order of CG < C, ECG ≪ EC, EGCG ≪ EGC, TF2 < TF1/TF1′ < TF (Table 1). As a lower IC50 value corresponds to a higher inhibitory activity, the inhibitory activity of each catechin/theaflavin group above followed an order opposite to that of the IC50 values. Taking the inhibitory activity into account, the detailed inhibition kinetics of the 8 tea polyphenols (excluding EC ad EGC) were analyzed in the following study.
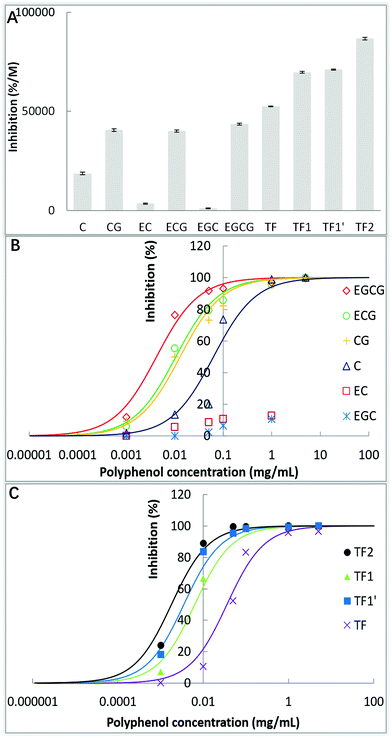 |
| Fig. 2 α-Glucosidase inhibition percentages per molar concentration of 10 tea polyphenols (A), and inhibition percentages at various concentrations of catechins (B) and theaflavins (C), in which the regression curves for IC50 calculation were fitted according to the eqn (2). | |
Table 1 Constants that indicate binding and inhibition of tea polyphenols against α-glucosidase
Tea polyphenols |
IC50 (mg mL−1) |
Inhibition type |
K
ic (M) |
1/Kic (M−1) |
KFQ (M−1) |
k
q (M−1 s−1) |
E
b (kcal mol−1) |
‘ND’ means not detected and calculated regarding IC50, Kic and 1/Kic for EC and EGC, due to very weak inhibition of the two tea polyphenols against α-glucosidase. ‘–’ means not calculated regarding KFQ and kq for C, EG and EGC due to very weak quenching effect on the enzyme. The different superscript letters in the same column indicate that they are significantly different (P < 0.05) from each other. The significance of difference in the Eb values was respectively analyzed for the 6 catechins and 4 theaflavins, because the molecular weights of the two group compounds are different, which may cause steric hindrance for the docking efficiency. |
C |
0.0834h |
Competitive |
8.83 × 10–4h |
1.13 × 103a |
— |
— |
−8.028c |
EC |
ND |
ND |
ND |
ND |
— |
— |
−7.140b |
EGC |
ND |
ND |
ND |
ND |
— |
— |
−6.952a |
CG |
0.0149f |
Competitive |
9.82 × 10–6f |
1.02 × 105c |
1.39 × 103a |
1.39 × 1011a |
−9.621d |
ECG |
0.0105e |
Competitive |
4.10 × 10–6e |
2.44 × 105d |
2.10 × 103c |
2.10 × 1011c |
−9.953e |
EGCG |
0.00642d |
Competitive |
1.44 × 10–6d |
6.97 × 105e |
2.06 × 103c |
2.06 × 1011c |
−12.028f |
TF |
0.0362g |
Competitive |
2.12 × 10–5g |
4.71 × 104b |
1.46 × 103b |
1.46 × 1011b |
−8.561A |
TF1 |
0.00589c |
Competitive |
1.01 × 10–6c |
9.90 × 105f |
7.48 × 103d |
7.48 × 1011d |
−9.041B |
TF1′ |
0.00412b |
Competitive |
7.90 × 10–7b |
1.27 × 106g |
8.38 × 103e |
8.38 × 1011e |
−8.986B |
TF2 |
0.00174a |
Competitive |
6.12 × 10–7a |
1.63 × 106h |
1.90 × 104f |
1.90 × 1012f |
−10.623C |
3.2 Inhibition kinetics
To obtain the inhibition types, Michaelis constant (Km, the substrate concentration at which the initial reaction velocity reaches half of the maximum one) and maximum initial reaction velocity, the kinetics study is performed, in which the Lineweaver–Burk equation (the double reciprocal Michaelis–Menten equation) is usually used. However, a relatively large error occurs in this plot when the substrate concentration is low. Besides, the Lineweaver–Burk equation is not able to neither distinguish between uncompetitive, non-competitive and mixed-type inhibition nor obtain the corresponding inhibition constants.25 The application of Dixon and Cornish-Bowden equations is useful in the case where the inhibition is more complex than the competitive and/or uncompetitive mechanisms.24,25 Both the Dixon and Cornish-Bowden plots were used to characterize the detailed inhibition kinetics of the tea polyphenols in this study. As shown in Fig. 3, all the 8 tea polyphenols inhibited the α-glucosidase activity in a similar mode. When the Dixon plots intersected at one point (there were certain experimental errors), and the Cornish-Bowden plots (the inserted ones) paralleled with each other (Fig. 3), the type of α-glucosidase inhibition by the studied tea polyphenols was defined as competitive inhibition. 3G (its molecular structure is shown in Fig. 9A) was also described as a competitive inhibitor of α-glucosidase (Fig. 9B). Therefore, these polyphenols were suggested to bind with the active site of α-glucosidase, competing with the substrate (here pNPG). Then, the competitive inhibition constant (Kic) that represents the dissociation constant of the inhibitor–enzyme complex was obtained from the absolute value of the intersection point abscissa of Dixon equations in the second quadrant (Fig. 3). The Kic values followed the order of CG < C, ECG ≪ EC, EGCG ≪ EGC, TF2 < TF1/TF1′ < TF (Table 1), which is similar to that of the IC50 values.
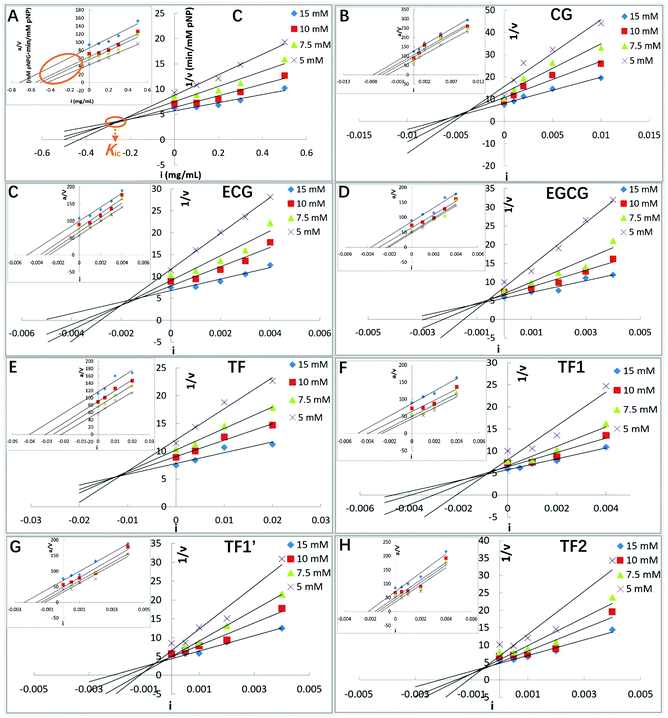 |
| Fig. 3 α-Glucosidase inhibition kinetics of C (A), CG (B), ECG (C), EGCG (D), TF (E), TF1 (F), TF1′ (G) and TF2 (H), including Dixon and Cornish-Bowden (inserted) equation plots. Here, i is the inhibitor (polyphenol) concentration, a is the substrate (pNPG) concentration, and v is the initial reaction velocity that is considered as the product (pNP) forming velocity. The data labels with different colors suggest various concentrations of the substrate (pNPG). As shown, the absolute value of the intersection point abscissa of Dixon equations is defined as the competitive inhibition constant (Kic). | |
3.3 Fluorescence quenching
Fluorescence quenching has been widely applied for analyzing the molecular interactions between quenchers and fluorescent proteins, including the polyphenol–glucosidase interactions. It is shown in Fig. 4A–C that three of the studied catechins, including C, EC and EGC, did not quench the enzyme intrinsic fluorescence significantly, while the catechins with a galloyl moiety (GM) (CG, ECG and EGCG) and four theaflavins (TF, TF1, TF1′ and TF2) were shown to exert the quenching effects to some extent (Fig. 4D–J). The quenching intensity was further analyzed by using the Stern–Volmer equation or its modified form. The Stern–Volmer equations for catechins and theaflavins with GM(s) were reported to be of linear type (Fig. 4K and L), suggesting that there was only one mechanism (static or dynamic) causing the quenching effects of these tea polyphenols.27 Differently, the plot of F0/F against the TF concentration concaved towards the y axis, and conformed to the exponential regression (Fig. 4L), indicating that there existed a ‘sphere-of-action’ or apparent static quenching mechanism for TF.27 Notably, the regression coefficients (R2) of the fitted quenching equations for all the polyphenols were above 0.99 (except for TF1, which was 0.9816), indicating that the selected respective regression analyses were reasonable. The values of fluorescence quenching constant (KFQ), which indicates the quenching efficiency of a quencher, for the 10 tea polyphenols followed the order of CG > C, ECG > EC, EGCG > EGC, TF2 > TF1/TF1′ > TF (Table 1). Accordingly, the bimolecular quenching constants (kq) that were calculated using eqn (5) or (6) followed a similar order (Table 1). Interestingly, the orders of both KFQ and kq were in accordance with that of the inhibitory activity of these polyphenols.
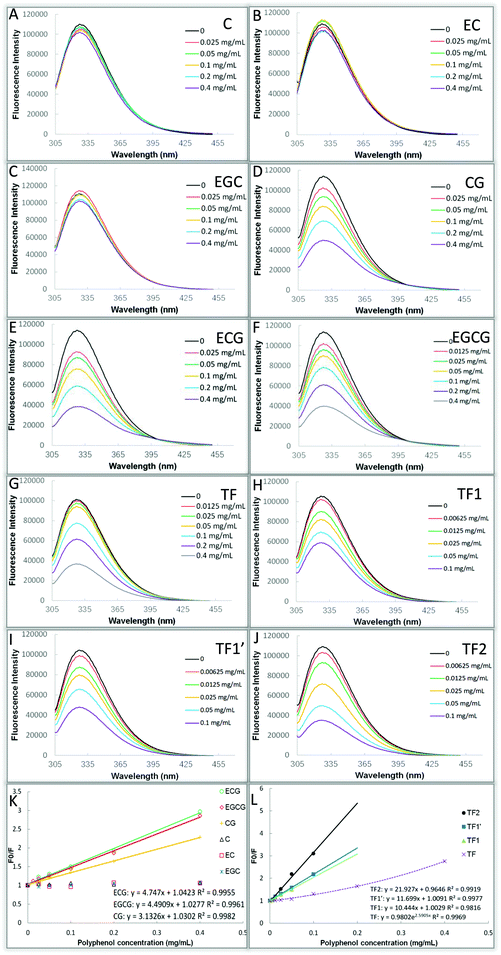 |
| Fig. 4 Fluorescence quenching effects of C (A), EC (B), EGC (C), CG (D), ECG (E), EGCG (F), TF (G), TF1 (H), TF1′ (I) and TF2 (J) on various phenolic compounds (different colors), respectively. The Stern–Volmer equation or its modified (exponential) form was used to analyze the maximum fluorescence intensity in the absence and presence of catechins (K) and theaflavins (L). | |
3.4 Molecular docking
Molecular docking has been used to predict the preferred orientation of one micromolecule (ligand) that binds with the active site of one macromolecule mainly through non-covalent bonding, and to identify the ligand/macromolecule residues involved in the binding interactions, as well as to obtain the binding energy (Eb) that indicates the binding affinity between the two molecules.30 This method was used to characterize the binding interactions between the tea polyphenols and α-glucosidase in this study. It was found that hydrogen bonding and π–π hydrophobic stacking (parallel and/or vertical conjugation) occurred between the functional groups (hydroxyl and benzene rings) of the tea polyphenols and amino acid residues at the active site of α-glucosidase (Fig. 5). Similar molecular interactions were found to occur between 3G and the enzyme (Fig. 9C). In general, the numbers of amino acid residues involved in the binding interactions of the polyphenols with a low inhibitory activity (C, EC and EGC) were fewer than the polyphenols with a relatively high inhibitory activity (CG, ECG, EGCG, TF, TF1, TF1′ and TF2) (Fig. 5). Specifically, for EGCG and TF2, the two phenolic compounds that show the respective highest inhibitory effects among the 6 catechins and 4 theaflavins, the polyphenol–enzyme non-covalent bonding numbers were 11 (8 hydrogen bonds and 3 π–π stacking interactions) and 15 (11 hydrogen bonds and 4 π–π stacking interactions), respectively (Fig. 5F and J). In addition, the Eb values were negative (Table 1), indicating that the binding process of tea polyphenols with α-glucosidase was an exothermic one; therefore, a lower temperature is favorable to the binding interactions. Interestingly, the absolute values of Eb followed the order of CG > C, ECG > EC, EGCG > EGC, TF2 > TF1/TF1′ > TF (Table 1), which is similar to the order of KFQ. This is not surprising as both the constants indicate, by definition, the binding affinity of a polyphenol to an enzyme.
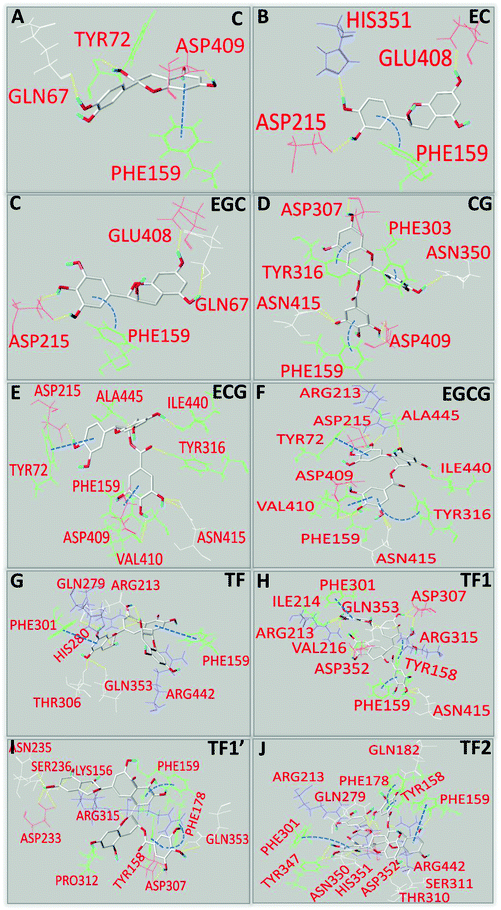 |
| Fig. 5 Molecular docking analysis of binding interactions of C (A), EC (B), EGC (C), CG (D), ECG (E), EGCG (F), TF (G), TF1 (H), TF1′ (I) and TF2 (J) with α-glucosidase, in which hydrogen bonds between the amino acid residues and hydroxyl groups of polyphenols are labelled with yellow dashed lines, and π–π conjugation between the aromatic residues of α-glucosidase and benzene rings of polyphenols is labelled with blue dashed lines (straight lines for parallel conjugation and curves for vertical conjugation). | |
3.5 Hypoglycemic effect
To explore the inhibitory effects of tea polyphenols on α-glucosidase in vivo, normal C57BL/6 mice were initially administered catechins and theaflavins, followed by the administration of sucrose solutions. It can be found from Fig. 6A, C and E that sucrose intake enhanced the blood glucose level of control mice significantly during 1 h after treatment. As an effective prescribed medicine, acarbose was able to markedly attenuate the enhancement of the postprandial blood sugar content (Fig. 6A, C and E), as well as the area under the curve (AUC) (Fig. 6B, D and F). The galloylated tea polyphenols, including EGCG, ECG, TF1 and TF2, were shown to exert higher retarding effects on increase in the postprandial blood glucose level than their respective ungalloylated counterparts, including EGC, EC, and TF (Fig. 6), which are similar to their inhibitory activity against α-glucosidase in vitro.
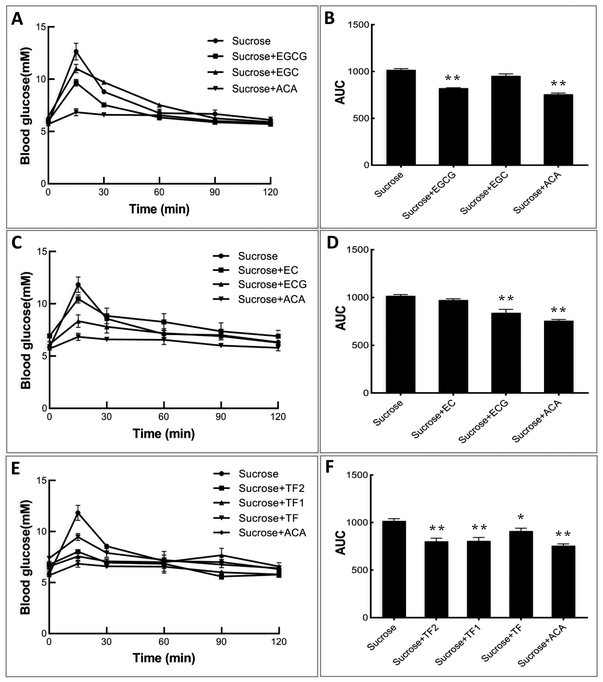 |
| Fig. 6 Effects of catechins and theaflavins on the postprandial blood sugar level (A, C and E) and the calculated area under the curve of glucose content (B, D and F) of mice for acute sucrose administration. * and ** mean significantly different between the polyphenol (or acarbose)-sucrose feeding groups and the sucrose feeding group by P < 0.05 and P < 0.01, respectively. | |
4. Discussion
4.1 The inhibitory activity of tea polyphenols
α-Amylase and α-glucosidase are two key enzymes for starch digestion both in vitro and in vivo.31 Inhibition of the activity of both or respective enzymes by dietary polyphenols has been reported as a recommended alternative approach for the application of commercial enzyme inhibitors.2,32 There exist ‘structure–inhibition relationships’ regarding enzyme inhibition by polyphenolic compounds, especially for the phenolic moieties.8,33,34 In previous studies, the galloyl moiety (GM) has been proved pivotal to the inhibitory activity of tea polyphenols against α-amylase.16,35 In this study, the role of the GM in the α-glucosidase inhibition by tea polyphenols (including catechins and theaflavins) was investigated. It was found that at the same molar concentration, the tea polyphenols with GM exhibited stronger inhibitory effects than those without GM (Fig. 2A). To further characterize the inhibitory activity precisely, the IC50 values were determined for all the polyphenols (Fig. 2B and C). According to the catechin structures, CG, ECG and EGCG are the galloylated forms of C, EC and EGC, respectively (Fig. 1). The IC50 values of the three galloylated catechins were significantly lower than those of their respective ungalloylated counterparts (Table 1), indicating that the substitution of galloyl at the 3-position (ring C) is able to increase the inhibitory activity of catechins to a large extent. Besides, TF1 (or TF1′) and TF2 are one and two galloyl substitution at the 3 and/or 3′-positions (ring C and/or C′) of TF (Fig. 1), and the IC50 values of the four polyphenols followed the order of TF2 < TF1/TF1′ < TF (Table 1). Therefore, similar to catechins, the introduction of GM into theaflavins also enhanced their inhibitory activity against α-glucosidase.
4.2 Inhibition kinetics of tea polyphenols
To elucidate how tea polyphenols develop enzyme inhibition and how GM contributes to the polyphenol–enzyme binding interactions, inhibition kinetics, fluorescence quenching and molecular docking were applied. By combining Dixon and Cornish-Bowden plots, all the studied tea polyphenols were found to be competitive inhibitors of α-glucosidase (except EC and EGC due to their very weak inhibitory effect) (Fig. 3), indicating that these polyphenols bound with the active site of α-glucosidase, instead of non-active sites.24 Notably, by inhibition kinetics definition, Kic is the dissociation constant of the inhibitor–enzyme complex; therefore, 1/Kic describes the association constant of the inhibitor with the enzyme (Fig. 7), and hence a higher 1/Kic value indicates the stronger binding affinity of a tea polyphenol to α-glucosidase.25 From EC to ECG (galloylated EC), and EGC to EGCG (galloylated EGC), the inhibition properties changed from a very weak to competitive characteristic (Table 1). This suggests that it is GM that promoted ECG and EGCG to enter into the enzyme active site and interact with the essential amino acid residues there, causing the inhibitory effects (Fig. 7). Besides, CG had a higher 1/Kic value than C, and as for the four theaflavins, the 1/Kic value increased with the increasing number of GM (TF2 > TF1/TF1′ > TF) (Table 1), suggesting that GM increased the binding of both catechins and theaflavins with α-glucosidase. It should be noted that as the competitive inhibitory characteristic increased (but not changed) for theaflavins with the increasing GM number, the GM of galloylated theaflavins was also able to enter into and interact with the active pocket of α-glucosidase (Fig. 7) (otherwise galloylated theaflavins would change to other inhibition types). Furthermore, it was found that there was a positive linear relationship between the IC50 and Kic values for the 7 studied tea polyphenols (Kic = 0.3547·IC50 − 0.0011, R2 = 0.9798, Fig. 8A). As a lower IC50 value describes a stronger inhibitory activity of a polyphenol, and a lower Kic value suggests a higher binding affinity of a polyphenol to an enzyme, the positive correlation indicates that α-glucosidase inhibition by these tea polyphenols is supposed to be caused by the binding interactions between them. Notably, although C and EC have similar molecular structures (only stereoisomerism of 3-OH, Fig. 1), C showed a much higher inhibitory effect than EC. This may result from the higher binding affinity of C to the α-glucosidase active site than EC, as suggested by the 1/Kic value (1.13 × 103 for C and not detectable for EC, Table 1). Interestingly, the molecular docking results supported this, and indicated that the hydrogen bonding of 3-OH (at the different side of ring B) of C with Tyr72 of α-glucosidase occurred, while this group (at the same side of ring B) of EC did not bond with the enzyme (Fig. 5A and B). Therefore, the stereoisomerism –OH of a polyphenol is suggested to affect its binding and inhibitory activity against α-glucosidase. Besides, the difference in the molecular structures between EC and EGC is due to the existence of 3′′-OH at ring B (Fig. 1). As both EC and EGC hardly showed the inhibitory activity (Fig. 2B), 3′′-OH did not contribute to the inhibitory effect of EGC. This hydroxyl also did not show hydrogen bonding with the α-glucosidase amino acid residues as indicated by the molecular docking results (Fig. 5C). However, as for ECG and EGCG (galloylated EC and EGC, respectively), the existence of 3′′-OH at ring B contributed to the α-glucosidase inhibition of EGCG and binding interactions with the enzyme, as suggested by the lower IC50 and higher 1/Kic values of EGCG than those of ECG (Table 1). Correspondingly, hydrogen bonds were found to form between 3′′-OH in EGCG and the amino acid residues at the active site of α-glucosidase, including Arg213 and Asp215 (Fig. 5F). Therefore, it is concluded that the difference in the contribution of 3′′-OH to the inhibition properties of EGC and EGCG (both polyphenols have this group) is due to the existence of GM. Here, the GM may form stacking interactions with ring B in solvents, which is supposed to realign the arrangement of the hydroxyl groups in ring B and GM (including 3′′-OH), thereby promoting the formation of hydrogen bonds with the α-glucosidase amino acid residues.36
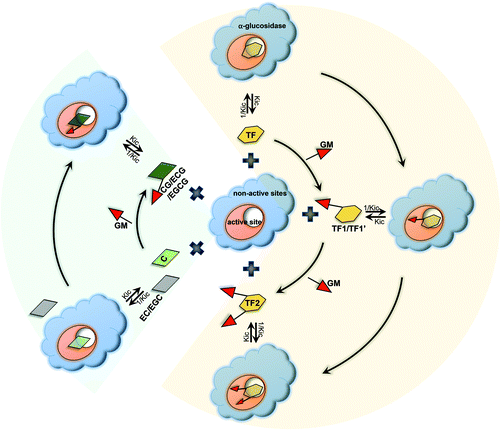 |
| Fig. 7 Scheme of binding interactions between tea polyphenols and α-glucosidase. For the 6 catechins, C binds with the enzyme active site, while EC and EGC cannot bind with the enzyme or the interactions are very weak. After the GM is introduced into the three compounds, the moiety promotes the respective galloylated ones (CG, ECG and EGCG) to enter into and bind with the active pocket of the enzyme. As for theaflavins, TF also interacts with the enzyme active site, and GM substitution enhances the binding interactions of TF1/TF1′ (3 or 3′-galloylated TF) and TF2 (3 and 3′-digalloylated TF) with α-glucosidase due to the binding property of GM with the enzyme active site. By inhibition kinetics definition, Kic is the dissociation constant of the polyphenol–enzyme complex; therefore, 1/Kic describes the association constant of a polyphenol with an enzyme. | |
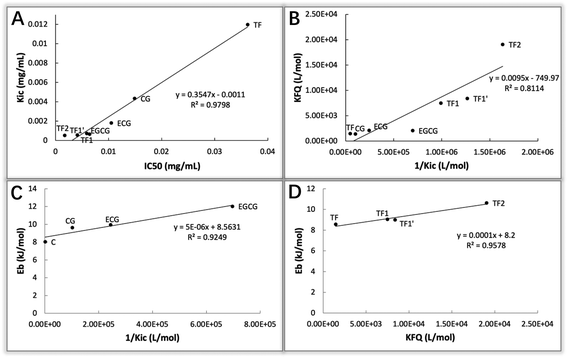 |
| Fig. 8 Correlations between the constants that indicate the inhibitory activity of tea polyphenols against α-glucosidase and polyphenol–enzyme binding interactions, including Kic − IC50 (A), KFQ − 1/Kic (B), Eb − 1/Kic (C) and Eb − KFQ (D). As for the correlations involving the binding energy (Eb, obtained from molecular docking), catechins and theaflavins were analyzed separately (C and D), as there likely existed steric hindrance for theaflavin–enzyme binding because the molecular weights of theaflavins were higher than those of catechins when the docking procedure was performed, which may limit the corresponding binding efficiency. | |
4.3 The fluorescence quenching effects of tea polyphenols
There are some aromatic amino acids existing in the α-glucosidase molecule, such as Phe and Tyr, providing the enzyme with the specific fluorescence emission property under ultraviolet excitation at certain wavelength.37 When exogenous hydroxyls and/or aromatic rings get close to the fluorescent amino acids, hydrogen bonding (hydroxyl–amino) and/or π-stacking (aromatic–aromatic) would take place, causing the quenching effect on the enzyme fluorescence spectrum.33 Therefore, fluorescence quenching was applied in this study to characterize the binding interactions between the tea polyphenols and α-glucosidase. As shown, all the polyphenols with a GM exhibited similar quenching characteristics, and their Stern–Volmer equations were linear (Fig. 4K and L). This is consistent with the similar competitive inhibitory characteristics of these compounds (Fig. 3). On the other hand, the polyphenols without a GM were shown with distinct quenching mechanism (no quenching effects for C, EC, and EGC, and the ‘sphere of action’ quenching mechanism for TF, Fig. 4K and L). Besides, in accordance with inhibition kinetics, along with the introduction of GM, the KFQ values of the polyphenols were increased (CG > C, ECG > EC, EGCG > EGC, TF2 > TF1/TF1′ > TF, Table 1). KFQ is able to reflect the intensity of binding interactions between a quencher and a fluorescent molecule.38 Therefore, these quenching results also indicate the important role of the GM in the binding of catechins and theaflavins with α-glucosidase. As both 1/Kic and KFQ can indicate the binding affinity of polyphenols with α-glucosidase, the correlation between the two constants was analyzed for the 7 compounds (Fig. 8). It was found that there was a positive linear relationship between the two constants (KFQ = 0.0095·1/Kic − 749.97, R2 = 0.8114, Fig. 8B), indicating that the combination of inhibition kinetics and fluorescence quenching may reasonably characterize the tea polyphenol–glucosidase non-covalent interactions, and the two methods may be mutually supported. Furthermore, from the value of kq, the quenching mechanisms can be obtained. The kq value of typical dynamic quenching (collision mechanism) has been reported to be around 1 × 1010 M−1 s−1,27 and all the kq values of tea polyphenols that had obvious quenching effects were 14–190 fold higher than this value (Table 1). As only one quenching mechanism (dynamic or static) occurs for a quencher with the linear characteristic Stern–Volmer equation, these tea polyphenols (CG, ECG, EGCG, TF, TF1, TF1′ and TF2) quenched α-glucosidase fluorescence through the static mechanism, i.e. the formation of the polyphenol–enzyme complex.
4.4 Molecular interactions between tea polyphenols and α-glucosidase simulated by molecular docking
Molecular docking is a computer-simulated characterization approach that evaluates the binding efficiency of two molecules at specific binding sites. In this study, it was used to characterize the binding interactions between tea polyphenols and α-glucosidase. Generally, the number of amino acid residues that are involved in the tea polyphenol–glucosidase interactions increase with the increasing inhibitory activity (Fig. 5). There was only one π–π conjugation with the aromatic amino acid residues (the main source of enzyme fluorescence) for C, EC and EGC (Fig. 5A, B and C), which supports the very weak fluorescence quenching effects on α-glucosidase of the three polyphenols (Fig. 4A, B and C). With more aromatic residues involved in the polyphenol–enzyme π–π interactions, such as Phe159,178,301,303 and Tyr72,158,316 (Fig. 5D–J), the fluorescence quenching effects increased (Fig. 4D–J). This explains the highest fluorescence quenching effect of TF2 (Fig. 4J). Interestingly, the role of the GM in the binding of the galloylated tea polyphenols with α-glucosidase was also suggested by the docking method, in which the hydroxyls of GM formed hydrogen bonds with the catalytic residues at the active site of α-glucosidase, and the benzene ring of GM conjugated with the aromatic rings of Phe and Tyr in a parallel and/or vertical mode (Fig. 5). Besides, structurally for GM, the C
O carbonyl is conjugated with the benzene ring, which facilitates the electron delocalization in GM. This may further promote the stability of the π-conjugation system of the galloylated polyphenols with the enzyme aromatic residues.39 Notably, a higher absolute value of Eb indicates a higher interaction intensity between a macromolecule and a ligand; therefore, the order of the Eb values (CG > C, ECG > EC, EGCG > EGC, TF2 > TF1/TF1′ > TF, Table 1) also suggests the contribution of GM to the catechin/theaflavin–enzyme binding interactions. In addition, to evaluate the rationality of docking results, the correlations among Eb, 1/Kic and KFQ for catechins or theaflavins were analyzed (Fig. 8C and D). It was found that there were positive linear relationships between the constants (Eb = 0.0001·KFQ + 8.2 for theaflavins; Eb = 0.000005·1/Kic + 8.6 for catechins), which may be illustrated by the fact that all the three constants describe the binding affinity of an inhibitor to an enzyme. This also suggests that the methods of inhibition kinetics, fluorescence quenching and molecular docking may be comprehensively combined to characterize the binding interactions between the tea polyphenols and α-glucosidase. It should be noted that although the galloylated theaflavins (TF1, TF1′ and TF2) were shown to exhibit a relatively higher binding affinity (higher 1/Kic and KFQ values) to α-glucosidase than the galloylated catechins (CG, ECG and EGCG), the Eb values were suggested to show an opposite tendency (theaflavins < catechins, Table 1). This may result from the higher steric hindrance for theaflavins–enzyme binding due to the higher molecular weights of theaflavins when the docking procedure is performed.17 However, the order of the Eb values was in accordance with that of IC50, 1/Kic and KFQ for the respective groups of 6 catechins or 4 theaflavins (Table 1), as the phenolic groups have similar molecular weights and steric configurations (the main difference lies in the absence/presence of GM, Fig. 1).
4.5 Verification of the role of the galloyl moiety
To further verify the role of the GM in the binding of tea polyphenols with α-glucosidase, one galloyl-based polyphenol that is composed of three GM branches and one glucose bone (1,3,6-tri-O-galloyl-β-D-glucose, named 3G, Fig. 9A) was selected to study its inhibition mechanism through inhibition kinetics and molecular docking (Fig. 9B and C). Notably, 3G was found to be a typical competitive inhibitor (Fig. 9B). As the glucosyl hardly showed inhibitory effects on enzymes,17 the inhibitory activity of 3G increased due to the contribution of GM that was able to enter into and interact with the active site of α-glucosidase (the competitive inhibitory characteristic). Supportively, the molecular docking result indicates that the three GMs interacted with the amino acid residues at the enzyme active site, where the hydroxyls of GMs formed hydrogen bonds with Gln182, Arg413, Ser441, Asn350 and Gln353, and the benzene rings of GMs conjugated with the aromatic essential residues, including Phe159 (vertical conjugation), Tyr316 (parallel conjugation) and Phe303 (vertical conjugation) (Fig. 9C). Therefore, it is concluded that the GM contributed a lot to the inhibitory activity of tea polyphenols and the binding interactions with α-glucosidase (Fig. 7).
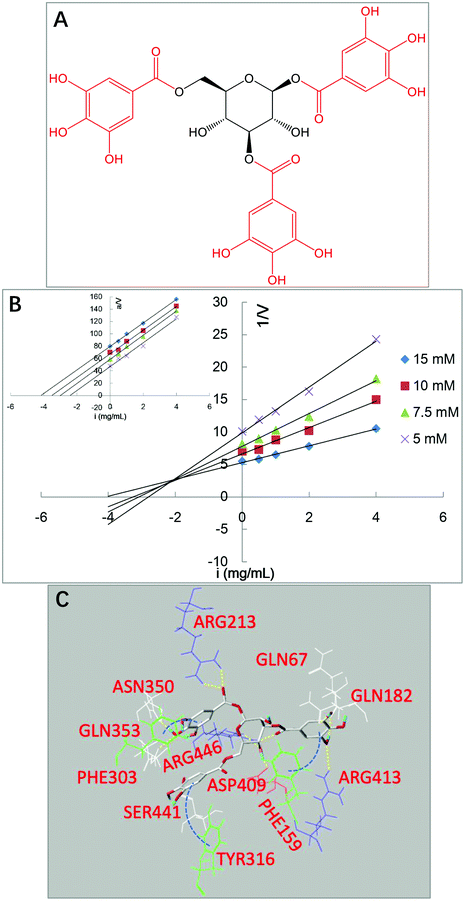 |
| Fig. 9 Molecular structure of a galloyl-based polyphenol (1,3,6-tri-O-galloyl-β-D-glucose, named3G) (A), and inhibition kinetics (B) and molecular docking (C) analyses of this compound. | |
4.6 The hyperglycemic effects of tea polyphenols
As sucrose is composed of glucose and fructose, linked through an α-glycosidic bond that is considered as the substrate of α-glucosidase, the inhibitory activity of tea polyphenols against the enzyme in vivo was studied using mice fed with sucrose. The stronger inhibitory effects on sucrose digestion in vivo of the galloylated catechins and theaflavins than the ungalloylated ones (Fig. 6) may be attributed to their higher inhibitory activity against α-glucosidase, as suggested by the IC50 and Kic values. Notably, the produced glucose is absorbed by enterocytes in the small intestine and transported into blood circulation via glucose transporters, including sodium-dependent glucose transporter 1 (SGLT1), glucose transporter 2 (GLUT2), etc. After that, blood glucose enters into functional tissues, such as brain, muscle and liver, under the action of insulin through glucose transporters, such as GLUT2 and GLUT4. Dietary polyphenols have been shown to inhibit the expression of SGLT1/GLUT2 in enterocytes or to promote the expression of GLUT4 in functional tissue cells,40,41 and both these effects retard the increase in the blood glucose level after meal. Therefore, the hypoglycemic effects of the galloylated tea polyphenols may also arise from the influence on glucose transporter expression, which may work synergistically with α-glucosidase inhibitory effects and needs to be further investigated. Conclusively, the finding that the GM plays an important role in the α-glucosidase inhibition of tea polyphenols indicates that the potential inhibitory activity of some phenolic compounds with GM(s) against the enzyme may be predicted. Besides, based on the binding property of the GM with the active sites of both α-glucosidase and α-amylase,15,16 it may be considered as a functional factor/fragment when designing or synthesizing pharmaceuticals for controlling postprandial hyperglycemia.
5. Conclusion
Galloyl is an important substitution of tea polyphenols that affects the bioactivities of those compounds. In this study, the role of a GM in the binding interactions between tea polyphenols and α-glucosidase and the resultant inhibitory activity were investigated. The GM was found to enhance the binding and inhibitory activity of catechins/theaflavins against the enzyme, as both the inhibitory activity and binding affinity followed the order of CG > C, ECG > EC, EGCG > EGC, TF2 > TF1/TF1′ > TF. The competitive inhibitory characteristics of the studied tea polyphenols, as well as the simulated docking details, indicate that the GM was able to enter into the active site of α-glucosidase and interact with the catalytic amino acid residues there, causing the inhibitory effects. Interestingly, the inhibition kinetics (typical competitive inhibition) and molecular docking (GMs involved in the binding interactions with α-glucosidase) results of a galloyl-based polyphenol that is composed of three GMs and one glucosyl supported this. Furthermore, the hypoglycemic effects of the galloylated tea polyphenols indicate that the GM may be considered a functional factor or fragment for exploring natural phytochemicals and designing efficient pharmaceuticals for the alleviation of postprandial hyperglycemia.
Conflicts of interest
The authors declare no competing financial interest.
Acknowledgements
This study is supported by the National Natural Science Foundation of China (No. 31901685), project funded by the China Postdoctoral Science Foundation (No. 2019M660267), the National Key Research and Development Program of China (2017YFD0400200-4), Mongolian Medicine Food and Drug Source Protection and Utilization Innovation Team Construction Project (190301), and the National Undergraduate Training Program for Innovation and Entrepreneurship (No. S201910712190). Lijun Sun thanks his previous PhD supervisors Prof. Michael J. Gidley who is at the University of Queensland, and Dr Fredrick J. Warren who is at Quadram Institute Bioscience. The inhibitory activity, inhibition kinetics and fluorescence quenching, as well as the related analysis were taught by both scientists, and the idea of α-glucosidase inhibition by tea polyphenols was also discussed with them.
References
- S. E. Inzucchi, R. M. Bergenstal, J. B. Buse, M. Diamant, E. Ferrannini, M. Nauck, A. L. Peters, A. Tsapas, R. Wender and D. R. Matthews, Management of hyperglycemia in type 2 diabetes: a patient-centered approach: position statement of the American Diabetes Association (ADA) and the European Association for the Study of Diabetes (EASD), Diabetes Spectrum, 2012, 25, 154–171 CrossRef.
- L. Sun and M. Miao, Dietary polyphenols modulate starch digestion and glycaemic level: A review, Crit. Rev. Food Sci. Nutr., 2020, 60, 541–555 CrossRef CAS.
-
C. H. Edwards and F. J. Warren, in Interdisciplinary Approaches to Food Digestion, Springer, 2019, pp. 277–290 Search PubMed.
- Y. Zheng, J. Tian, W. Yang, S. Chen, D. Liu, H. Fang, H. Zhang and X. Ye, Inhibition mechanism of ferulic acid against α-amylase and α-glucosidase, Food Chem., 2020, 317, 126346 CrossRef CAS.
- C. Raghu, H. Arjun and P. Anantharaman, In vitro and in silico inhibition properties of fucoidan against α-amylase and α-D-glucosidase with relevance to type 2 diabetes mellitus, Carbohydr. Polym., 2019, 209, 350–355 CrossRef.
- Q. Zhao, Y. Luo, X. Zhang, Q. Kang, D. Zhang, L. Zhang, L. Bai and Z. Deng, A severe leakage of intermediates to shunt products in acarbose biosynthesis, Nat. Commun., 2020, 11, 1–15 Search PubMed.
- N. Miller, C. J. Malherbe and E. Joubert, In vitro α-glucosidase inhibition by honeybush (Cyclopia genistoides) food ingredient extract—potential for dose reduction of acarbose through synergism, Food Funct., 2020, 11, 6476–6486 RSC.
- Y. Narita and K. Inouye, Inhibitory effects of chlorogenic acids from green coffee beans and cinnamate derivatives on the activity of porcine pancreas α-amylase isozyme I, Food Chem., 2011, 127, 1532–1539 CrossRef CAS.
- A. Colantuono, R. Ferracane and P. Vitaglione, In vitro bioaccessibility and functional properties of polyphenols from pomegranate peels and pomegranate peels-enriched cookies, Food Funct., 2016, 7, 4247–4258 RSC.
- S. Yan, H. Shao, Z. Zhou, Q. Wang, L. Zhao and X. Yang, Non-extractable polyphenols of green tea and their antioxidant, anti-α-glucosidase capacity, and release during in vitro digestion, J. Funct. Foods, 2018, 42, 129–136 CrossRef CAS.
- Y. Narita and K. Inouye, Kinetic Analysis and Mechanism on the Inhibition of Chlorogenic Acid and Its Components against Porcine Pancreas α-Amylase Isozymes I and II, J. Agric. Food Chem., 2009, 57, 9218–9225 CrossRef CAS.
- H. Gao, Y.-N. Huang, B. Gao, P.-Y. Xu, C. Inagaki and J. Kawabata, α-Glucosidase inhibitory effect by the flower buds of Tussilago farfara L, Food Chem., 2008, 106, 1195–1201 CrossRef CAS.
- L. Sun, F. J. Warren, M. J. Gidley, Y. Guo and M. Miao, Mechanism of binding interactions between young apple polyphenols and porcine pancreatic α-amylase, Food Chem., 2019, 283, 468–474 CrossRef CAS.
- Y. Zheng, W. Yang, W. Sun, S. Chen, D. Liu, X. Kong, J. Tian and X. Ye, Inhibition of porcine pancreatic α-amylase activity by chlorogenic acid, J. Funct. Foods, 2020, 64, 103587 CrossRef CAS.
- L. Sun, M. J. Gidley and F. J. Warren, The mechanism of interactions between tea polyphenols and porcine pancreatic alpha–amylase: Analysis by inhibition kinetics, fluorescence quenching, differential scanning calorimetry and isothermal titration calorimetry, Mol. Nutr. Food Res., 2017, 61, 1700324 CrossRef.
- L. Sun, F. J. Warren, G. Netzel and M. J. Gidley, 3 or 3′-Galloyl substitution plays an important role in association of catechins and theaflavins with porcine pancreatic α-amylase: The kinetics of inhibition of α-amylase by tea polyphenols, J. Funct. Foods, 2016, 26, 144–156 CrossRef CAS.
- J. Cao, Y. Zhang, L. Han, S. Zhang, X. Duan, L. Sun and M. Wang, Number of galloyl moieties and molecular flexibility are both important in alpha-amylase inhibition by galloyl-based polyphenols, Food Funct., 2020, 3838–3850 RSC.
- M. Honda and Y. Hara, Inhibition of Rat Small Intestinal Sucrase and. ALPHA.-Glucosidase Activities by Tea Polyphenols, Biosci., Biotechnol., Biochem., 1993, 57, 123–124 CrossRef CAS.
- X. Yang and F. Kong, Evaluation of the in vitro α–glucosidase inhibitory activity of green tea polyphenols and different tea types, J. Sci. Food Agric., 2016, 96, 777–782 CrossRef CAS.
- O. Kamiyama, F. Sanae, K. Ikeda, Y. Higashi, Y. Minami, N. Asano, I. Adachi and A. Kato, In vitro inhibition of α-glucosidases and glycogen phosphorylase by catechin gallates in green tea, Food Chem., 2010, 122, 1061–1066 CrossRef CAS.
- J. Zhu, B. Zhang, C. Tan and Q. Huang, α-Glucosidase inhibitors: consistency of in silico docking data with in vitro inhibitory data and inhibitory effect prediction of quercetin derivatives, Food Funct., 2019, 10, 6312–6321 RSC.
- T. Gong, X. Yang, F. Bai, D. Li and Y. Guo, Young Apple Polyphenols as Natural α-Glucosidase Inhibitors: in Vitro and in Silico Studies, Bioorg. Chem., 2020, 96, 103625 CrossRef CAS.
-
J. A. Goodrich, J. F. Kugel and J. K. Kugel, Binding and kinetics for molecular biologists, CSHL Press, 2007 Search PubMed.
- P. J. Butterworth, The use of Dixon plots to study enzyme inhibition, Biochim. Biophys. Acta, 1972, 289, 251–253 CrossRef CAS.
- A. Cornish-Bowden, A simple graphical method for determining the inhibition constants of mixed, uncompetitive and non-competitive inhibitors (Short Communication), Biochem. J., 1974, 137, 143–144 CrossRef CAS.
- T. Dai, J. Chen, D. J. McClements, T. Li and C. Liu, Investigation the interaction between procyanidin dimer and α-glucosidase: Spectroscopic analyses and molecular docking simulation, Int. J. Biol. Macromol., 2019, 130, 315–322 CrossRef CAS.
-
J. R. Lakowicz, Principles of fluorescence spectroscopy, Springer science & business media, 2013 Search PubMed.
- L. Han, C. Fang, R. Zhu, Q. Peng, D. Li and M. Wang, Inhibitory effect of phloretin on α-glucosidase: Kinetics, interaction mechanism and molecular docking, Int. J. Biol. Macromol., 2017, 95, 520–527 CrossRef CAS.
- Y.-T. Deng, S.-Y. Lin-Shiau, L.-F. Shyur and J.-K. Lin, Pu-erh tea polysaccharides decrease blood sugar by inhibition of α-glucosidase activity in vitro and in mice, Food Funct., 2015, 6, 1539–1546 RSC.
- M. Miao, H. Jiang, B. Jiang, Y. Li, S. W. Cui and Z. Jin, Elucidation of structural difference in theaflavins for modulation of starch digestion, J. Funct. Foods, 2013, 5, 2024–2029 CrossRef CAS.
- Q.-Z. Liu, H. Zhang, H.-Q. Dai, P. Zhao, Y.-F. Mao, K.-X. Chen and Z.-X. Chen, Inhibition of Starch Digestion: The Role of Hydrophobic Domain of Both α-Amylase and Substrates, Food Chem., 2020, 128211 Search PubMed.
- L. Sun, Y. Wang and M. Miao, Inhibition of α-amylase by polyphenolic compounds: Substrate digestion, binding interactions and nutritional intervention, Trends Food Sci. Technol., 2020, 104, 190–207 CrossRef CAS.
- Q. Fei, Y. Gao, X. Zhang, Y. Sun, B. Hu, L. Zhou, S. Jabbar and X. Zeng, Effects of oolong tea polyphenols, EGCG, and EGCG3 ′′Me on pancreatic α-amylase activity in vitro, J. Agric. Food Chem., 2014, 62, 9507–9514 CrossRef CAS.
- J. Xiao, X. Ni, G. Kai and X. Chen, A review on structure–activity relationship of dietary polyphenols inhibiting α-amylase, Crit. Rev. Food Sci. Nutr., 2013, 53, 497–506 CrossRef CAS.
- L. Sun, M. J. Gidley and F. J. Warren, The mechanism of interactions between tea polyphenols and porcine pancreatic
alpha-amylase: Analysis by inhibition kinetics, fluorescence quenching, differential scanning calorimetry and isothermal titration calorimetry, Mol. Nutr. Food Res., 2017, 61, 1700324 CrossRef.
- L. K. Williams, X. Zhang, S. Caner, C. Tysoe, N. T. Nguyen, J. Wicki, D. E. Williams, J. Coleman, J. H. McNeill and V. Yuen, The amylase inhibitor montbretin A reveals a new glycosidase inhibition motif, Nat. Chem. Biol., 2015, 11, 691–696 CrossRef CAS.
- L. Zeng, H. Ding, X. Hu, G. Zhang and D. Gong, Galangin inhibits α-glucosidase activity and formation of non-enzymatic glycation products, Food Chem., 2019, 271, 70–79 CrossRef CAS.
- R. Ferrer-Gallego, R. Gonçalves, J. C. Rivas-Gonzalo, M. T. Escribano-Bailón and V. de Freitas, Interaction of phenolic compounds with bovine serum albumin (BSA) and α-amylase and their relationship to astringency perception, Food Chem., 2012, 135, 651–658 CrossRef CAS.
- E. Lo Piparo, H. Scheib, N. Frei, G. Williamson, M. Grigorov and C. J. Chou, Flavonoids for Controlling Starch Digestion: Structural Requirements for Inhibiting Human α-Amylase, J. Med. Chem., 2008, 51, 3555–3561 CrossRef CAS.
- J. A. Villa-Rodriguez, A. Kerimi, L. Abranko, S. Tumova, L. Ford, R. S. Blackburn, C. Rayner and G. Williamson, Acute metabolic actions of the major polyphenols in chamomile: an in vitro mechanistic study on their potential to attenuate postprandial hyperglycaemia, Sci. Rep., 2018, 8, 1–14 CrossRef CAS.
- M. Ueda-Wakagi, K. Hayashibara, T. Nagano, M. Ikeda, S. Yuan, S. Ueda, Y. Shirai, K.-i. Yoshida and H. Ashida, Epigallocatechin gallate induces GLUT4 translocation in skeletal muscle through both PI3K-and AMPK-dependent pathways, Food Funct., 2018, 9, 4223–4233 RSC.
|
This journal is © The Royal Society of Chemistry 2021 |
Click here to see how this site uses Cookies. View our privacy policy here.