DOI:
10.1039/D0FO02319A
(Paper)
Food Funct., 2021,
12, 203-214
In vitro simulated digestion and colonic fermentation of lychee pulp phenolics and their impact on metabolic pathways based on fecal metabolomics of mice†
Received
2nd September 2020
, Accepted 20th November 2020
First published on 21st November 2020
Abstract
Lychee pulp phenolics (LPP) was subjected to four simulated gastrointestinal digestions and colonic fermentation to investigate the changes in its phenolic composition and bioactivities; the fecal metabolic profiles of LPP-fed mice were also elucidated using UHPLC-ESI-QTOF-MS/MS. After simulated salivary, gastric and intestinal digestion, slight increases in phenolic acids and (+)-catechin occurred relative to undigested LPP, whereas other flavonoids showed an opposite trend. Unlike the above-described separate simulated digestions, successive gastrointestinal digestion significantly enhanced the release of phenolic compounds (p < 0.05), gallic acid (413.79%), ferulic acid (393.69%), (+)-catechin (570.27%) and rutin (247.54%). During colonic fermentation, ten detected phenolics were utilized by gut microbes, among which procyanidin B2 (22.35%) was the most degraded. LPP fermentation accelerated the production of short-chain fatty acids (122.79%). The metabolic pathways altered by LPP including unsaturated fatty acid, biotin, and nicotinamide metabolism may be the potential regulatory mechanisms and associated with the integrity of the gut barrier. These findings indicate that LPP may act as a promising candidate to protect gut health.
Introduction
Lychee (Litchi chinensis Sonn.) referring to the family of Sapindaceae is one of the most popular tropical fruits which is widely cultivated or naturally grown worldwide.1,2 As research on lychee fruits has been undertaken, lychee pulp has been considered as a functional food because of its specific components endowed with antioxidant, anti-inflammatory, hypolipidemic and anticancer potential.3–5 The biological potential of lychee pulp is mainly attributed to its high phenolic contents, especially flavonoids.6 The comparatively higher content phenolic compounds, including quercetin 3-O-rutinose-7-O-α-L-rhamnoside, rutin and (+)-catechin, have been isolated and identified by our group.7 It has been demonstrated that lychee pulp phenolics (LPP) exhibit robust antioxidant activity in the vitro chemical and cellular antioxidant assays,7 but whether the antioxidant activities of these phenolics will change in the digestive system is unknown. The in vivo bioactivities of phenolics depend on their liberation, stability and the way in which they are metabolized in the digestive tract.8 Thus, it is necessary to elucidate their changes and bioactivities under digestion conditions.
Simulated human gastrointestinal digestion and colonic fermentation models have been established and applied to assess the changes in compound compositions before absorption.9 This method is characterized by rapidity, security and no ethical restrictions.10 A previous study reported an increase in the antioxidant activities of various lychee pulp after in vitro simulated gastric and intestinal digestion,11 which may be attributed to the release of phenolics from food matrices. However, no study has focused on the biochemical changes of lychee pulp phenolics with purification under in vitro separate, successive digestion or colonic fermentation conditions.
Metabolomics analysis-based liquid chromatography-mass spectrometry is emerging as a powerful technique that could accurately and rapidly identify hundreds of small-molecule metabolites.12 Metabolites in feces are the final products from both gut cellular and microbial metabolism, reflecting gut function and the status of the gut microbes.13 Therefore, fecal metabolomics has gradually received attention and is considered a favored method for researching diet or disease metabolite biomarkers and the connection between symbiotic microbes and host health.12 To better elucidate the in vivo metabolic regulatory mechanisms of LPP, it is crucial to investigate the relative intensity of metabolites and the changes in metabolic pathways in mice after LPP consumption.
For the reasons above, the present research was planned to investigate the following: (1) the composition and antioxidant activities of LPP under in vitro simulated salivary, gastric, intestinal and successive gastrointestinal digestion conditions; (2) the effects of LPP on phenolic profile changes and the production of short-chain fatty acids (SCFA) during fecal fermentation; and (3) the changes in metabolic pathways after LPP intake based on fecal metabolomics of mice.
Materials and methods
Chemicals and materials
Human saliva α-amylase (1500 U mL−1), pepsin (25
000 U mL−1), pancreatin (800 U mL−1) and bile salts were obtained from Sigma-Aldrich Chemical Co. (St Louis, MO, USA). Trolox, phenolic acid standard and flavonoid standards were purchased from Yuanye Biotechnology Co., Ltd (Shanghai, China). Quercetin 3-O-rutinose-7-O-α-L-rhamnoside was prepared following a previous method reported by our team.7 HPLC-grade methanol, acetic acid, acetonitrile, 2,4,6-tripyridyl-s-triazine, ferrous sulphate, fluorescein disodium salt, 2′,7′-dichlorofluorescin diacetate and 2′,2′-azobis (2-amidinopropane) dihydrochloride were purchased from Sigma-Aldrich Chemical Co. (Oakville, ON, Canada).
Preparation of LPP
Fresh ripe lychee (cv. Guiwei) was purchased from a local market in Guangzhou, China. LPP was prepared following our previously reported method.4 Briefly, lychee pulp (500 g) was mashed in 1.5 L of aqueous ethanol solution (80%, v/v) for 5 min using a Philips blender. After the mixture was centrifuged at 2800g for 10 min (Changsha Xiangzhi Instrument Co. Ltd, Changsha, China), the supernatant was collected and the residue was re-extracted with 80% ethanol solution. The supernatant was combined and concentrated in a rotary evaporator (Yamato RE212, Japan) at 45 °C and further applied onto an HPD-826 resin column (Cangzhou Bonchem Co., Ltd, Cangzhou, China). The column was eluted using ultrapure water to remove the majority of non-phenolic impurities. The organic phase acquired through eluting with aqueous ethanol solution (95%, v/v) was collected, concentrated and lyophilized (Biosafer-10B, Biosafer Technology Co. Ltd, Ningbo, China) for obtaining LPP.
Simulated digestion of LPP
Simulated salivary, gastric and intestinal media were prepared according to a previously reported study.14 The detailed procedures are described in the ESI.†
Simulated successive gastrointestinal digestion (SGID) including three phases was carried out following the method reported by Juaniz et al.14 Briefly, 0.5 g of LPP was sequentially digested at 37 °C as follows: oral phase (addition of salivary medium and mixing for 30 min), gastric phase (addition of gastric medium and mixing for 2 h), and intestinal phase (addition of intestinal medium and mixing for 2 h). The aliquot was centrifuged at 4000g for 5 min and the supernatant was collected as the SGID sample.
To elucidate the effects of each digestive phase on the release of phenolics and antioxidant activities, three separated simulated digestions were carried out by the method of the same report with slight modifications. Simulated salivary digestion (SSD): 0.5 g of LPP was mixed with the salivary medium for 30 min at 37 °C. After centrifugation, the supernatant was collected as the SSD sample. Simulated gastric digestion (SGD): 0.5 g of LPP was mixed with the gastric medium for 2 h at 37 °C. The aliquot was centrifuged and the supernatant was collected as the SGD sample. Simulated intestinal digestion (SID): 0.5 g of LPP was mixed with the intestinal medium for 2 h at 37 °C. The aliquot was centrifuged and the supernatant was collected as the SID sample. All samples were stored at −20 °C until use.
Determination of total phenolic and flavonoid contents
The total phenolic content (TPC) in the sample was determined following a modified Folin–Ciocalteu assay with a 96-well plate.15 The TPC of each sample was presented as mg of gallic acid equivalents (GAE) per gram DW of LPP.
The total flavonoid content (TFC) in the sample was measured according to a previously reported procedure.7 The results of each sample were expressed as rutin equivalents (RE) per gram DW of lychee pulp.
Quantification of the antioxidant activity
Ferric reducing antioxidant power (FRAP) was determined by the reported method.11 After reacting with the FRAP working solution in the dark for 10 min, the absorbance of the sample was detected at 593 nm using a UV-2600 spectrometer (Shimadzu Inc., Kyoto, Japan). The FRAP values were calculated by standard curve (0.5–5 mmol mL−1), expressed as ferrous sulfate equivalents (mmol FeE per g DW).
Hydrophilic oxygen radical absorbance capacity (ORAC) assay was performed by the reported method.7 5 μL of the sample in potassium phosphate buffer reacted with 200 μL of fluorescein for 20 min at 37 °C, with addition of 20 μL of AAPH solution. The excitation and emission wavelengths were 485 and 520 nm, respectively, every 5 min for 12 cycles. Trolox served as the standard substance to build a standard curve (6.25–50 μmol L−1). The ORAC value was expressed as Trolox equivalents (μmol TE per g DW).
Individual phenolic compound quantitative determination by HPLC-DAD
Individual phenolic compounds in samples were analyzed and quantified using an Agilent 1260 HPLC system equipped with a diode array detector (DAD) and an Agilent Zorbax SB-C18 columns (250 mm × 4.6 mm, 5 μm, Palo Alto, CA), as previously reported by our team.7 Before chromatographic analysis, aliquots of each treatment were filtered through a 0.45 μm membrane and the resultant filtrates were injected into the sample bottles. The parameters set were: injection volume, 20 μL; column temperature, 30 °C and flow rate, 1.0 mL min−1. The mobile phase comprised acetonitrile (solvent A) and 0.4% glacial acetic acid (solvent B). The gradient elution program was applied as follows: 0–40 min, 5%–25% A; 40–45 min, 25%–35% A; and 45–50 min, 35%–50% A. The peaks detected at a wavelength of 280 nm were identified, based on retention times measured for standard substances. The contents of individual phenolic compounds were calculated using the standard curves established by the corresponding phenolic substances. Individual phenolic contents were expressed as mg per gram DW of LPP.
In vitro colonic fermentation
The LPP sample was subjected to colonic fermentation following a previously reported procedure.14 Fresh feces were collected from four healthy donors (two males and two females) who did not have gastrointestinal disease or accept antibiotic ingestion for over three months before donation. After blending equal amounts of feces from 4 donors, the resultant feces were added to autoclaved phosphate buffer saline, homogenized and filtered through sterile gauze to obtain a fecal slurry (10%, w/v). The growth medium was prepared with the same molarity as reported in the study. For colonic fermentation, 1 mL of LPP solution (prepared by dissolving 0.5 g of LPP power in PBS to make 4 mL) was added to 4.5 mL of growth medium in a 15 mL tube containing 4.5 mL of fecal slurry, and stirred and incubated at 37 °C under a strict anaerobic environment. The fermented sample was taken out at 0, 6, 12, 24 and 48 h, respectively, for further analysis.
Analysis of the pH shift and the SCFA contents
The pH shift in the fermented sample was determined in an ice water bath using a PB-10 pH meter (Hetian Apparatus Co., Shanghai, China).
The SCFA contents were measured following as previously described with adjustment.16 After centrifugation, the supernatants were collected and filtered through a 0.22 μm membrane. The analysis was conducted with a 2010Plus gas chromatograph equipped with a flame ionization detector and a DB-FFAP capillary column (30 m × 0.25 mm × 0.25 μm). The flow rates of N2, H2 and air were 30, 30 and 400 mL min−1, respectively, with a split ratio of 1
:
9. The oven temperature was set at 70 °C for 1 min, increased to 220 °C at a speed of 10 °C min−1, held at 220 °C for 5 min, further risen to 240 °C at the same speed and held at 240 °C for 14 min. The temperatures of both the injector and detector were 240 °C while the injection volume of each sample was 1 μL. The SCFA contents were expressed as nmol mL−1.
Mice and treatment
A total of twenty C57BL/6 male mice at eight-week-old, obtained from the Center of Laboratory Animal Science Research of Guangzhou University of Chinese Medicine (Guangzhou, China), were housed in a standard animal room maintained at 25 ± 1 °C and humidity of 55 ± 5%, with a 12 h light/dark cycle. Mice were fed ad libitum with distilled water and standard rat chow (AIN-93G), of which the composition is provided in Table S1.† After adaption for 7 days, the mice were randomly assigned to the control and LPP groups (n = 10 in each group). The LPP group was orally fed with LPP (200 mg kg−1 d−1) in drinking water by gavage for 21 days, while the control group was given distilled water only. Animals were anesthetized in the chambers by ether inhalation after fasting overnight. It should be pointed out that serum and liver tissue were also harvested for analysis of hepatic aminotransferase and other properties (unpublished results) to prevent excessive use in mice. Besides, only two kinds of anesthetics were available in our laboratory: pentobarbital and ether, of which ether was applied because pentobarbital may alter hepatic aminotransferase activities,17 serum metabolite18 and blood sampling.19 The colonic feces were collected and stored at −80 °C until analysis.
This experiment was performed after authorization by the Ethics Committee on Animals Experiment of Guangdong Academy of Agricultural Sciences (approval number: 00213903). All procedures followed the guidelines of Laboratory Animal Requirements of Environment and Housing Facilities (GB 14925–2010).
Fecal metabolomics of mice
Metabolomics analysis was conducted by UHPLC-ESI-QTOF-MS/MS following a modified method reported previously.20 Briefly, the fecal sample (25 mg) was blended with 80% methanol (1 mL) containing 2 μg of L-2-chlorophenylalanine, centrifuged at 4000g for 10 min, filtered through a 0.22 μm membrane and transferred to a glass vial. LC-MS analysis was conducted using an Agilent 1290 UHPLC system coupled to a Triple TOF 6600 mass spectrometer and equipped with a BEH amide column (1.7 μm, 2.1 mm × 100 mm). The parameters set were: column temperature, 25 °C; flow rate, 0.5 mL min−1; and injection volume, 1 μL. The mobile phase consisted of water of 25 mmol L−1 NH4Ac and NH4OH (A) and acetonitrile (B). The linear gradient was programmed as follows: 0–0.5 min, 95% B; 0.5–7.0 min, 95%–65% B; 7.0–8.0 min, 65%–40% B; 8.0–9.0 min, 40% B; 9.0–9.1 min, 40%–95% B; and 9.1–12.0 min, 95% B. The conditions of the ESI source were set as follows: temperature, 600 °C; gas 1, 60 psi; gas 2, 60 psi; curtain gas, 35 psi; and ISVF, 5000 V or −4000 V in positive or negative ion modes, respectively.
Metabolite identification was performed using in-house MS2, KEGG (http://www.genome.jp/kegg/) and HMDB (http://www.hmdb.ca/) databases. Raw MS data were transferred to the mzXML format using ProteoWizard and processed (e.g. peak integration) by R package XCMS R (version 3.2). After processing, the obtained data matrix was imported to the SIMCA15.0.2 software (Umetrics, Umea, Sweden) for multivariate analysis. Orthogonal projection to latent structures squares-discriminant analysis (OPLS-DA) was applied to estimate the overall difference between two experimental groups. Metabolites with variable importance in the projection (VIP) >1 and p < 0.05 (Student's t-test) were defined as differentially expressed metabolites. KEGG and MetaboAnalyst (http://www.metaboanalyst.ca/) databases were applied to search pathways annotated and enriched.
Statistical analysis
All sample data were displayed as the mean ± standard deviation (SD) after triplicate experiments. One-way analysis of variance (ANOVA) was applied to estimate the between-group difference using SPSS 24 (Chicago, USA). Tukey's test was used to compare statistically significant differences, and the significance threshold was set at p < 0.05 for this test.
Results
Effect of simulated digestion on TPC, TFC and antioxidant activities
The changes in the TPC, TFC and antioxidant activities of in vitro separately and successively simulated gastrointestinal-digested LPP compared with undigested LPP are presented in Fig. 1. The undigested LPP, extracted with deionized water, contained TPC and TFC values of 128.14 mg GAE per g DW and 296.12 mg TE per g DW, respectively, whereas those from the SSD group were 75.70% and 96.77% of the undigested values, respectively. A considerable decrease (p < 0.05) was noticed after SGD treatment compared to undigested LPP; more specifically, 13.94% losses for TPC and 15.15% losses for TFC were recorded. The TPC and TFC of LPP were comparable (p > 0.05) between the SGD and SID groups. However, SGID treatment promoted an effective increase in the TPC and TFC, which were significantly higher than those in the other groups (p < 0.05).
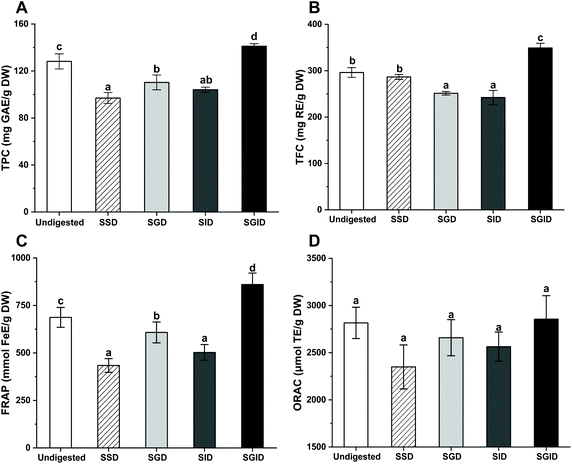 |
| Fig. 1
In vitro digestion of LPP, (A) total phenolic content (TPC); (B) total flavonoid content (TFC); (C) FRAP antioxidant activity; and (D) H-ORAC antioxidant activity. Undigested samples were extracted with water. DW: dry weight; SSD: simulated salivary digestion; SGD: simulated gastric digestion; SID: simulated intestinal digestion; SGID: simulated gastrointestinal digestion; bars with no letter in common are significantly different (p < 0.05). | |
The antioxidant activities of LPP after in vitro simulated digestions were further investigated based on FRAP and ORAC assays. The antioxidant activities of LPP showed a similar trend to the TPC results. Compared with undigested LPP, the FRAP values of the SSD, SGD and SID groups decreased dramatically (p < 0.05), with that of the SSD group being the lowest. However, the FRAP values of the SGID group were significantly higher than that of undigested LPP (p < 0.05). For the ORAC assay, although LPP showed the highest value among all samples after SGID treatment, no significantly different results were observed (p > 0.05).
Effect of in vitro digestion on monomeric phenolics
The contents of identified and quantified monomeric phenolics are presented in Table 1. Five phenolic acids (gallic acid, vanillic acid, caffeic acid, ferulic acid and syringic acid) and five flavonoids ((+)-catechin, procyanidin B2, quercetin 3-O-rutinose-7-O-α-L-rhamnoside, procyanidin A2 and rutin) were detected in undigested LPP. Ferulic acid was the most abundant phenolic acid in all samples, and its amount increased significantly (p < 0.05) after in vitro separately or successively simulated gastrointestinal digestion compared to the undigested group. No statistically different (p > 0.05) contents for ferulic acid were observed among the SSD, SGD and SID groups. However, the ferulic acid contents after SGID were higher than that in the other groups (p < 0.05), reaching up to 4.37 mg per g DW. The gallic acid contents in LPP after SGID treatment were higher than that in the other groups, while a slight increase occurred after the SSD, SGD or SID treatments. In addition, there were no significant differences (p > 0.05) in the contents of other phenolic acids among different groups.
Table 1 Individual phenolic contents in LPP before and after simulated in vitro human digestion
Compound (mg per g DW) |
Undigested |
In vitro separate gastrointestinal digestion |
SGID |
SSD |
SGD |
SID |
Values with different letters are significantly different (p < 0.05). LOQ: limit of quantification; the LOQ value was 0.01 mg g−1 for procyanidin B2. |
Phenolic acids |
Gallic acid |
0.29 ± 0.11a |
0.41 ± 0.03a |
0.40 ± 0.03a |
0.38 ± 0.01a |
1.20 ± 0.24b |
Vanillic acid |
0.69 ± 0.26a |
0.78 ± 0.10a |
0.72 ± 0.14a |
0.75 ± 0.06a |
0.63 ± 0.12a |
Caffeic acid |
0.39 ± 0.03a |
0.43 ± 0.09a |
0.39 ± 0.03a |
0.32 ± 0.06a |
0.39 ± 0.03a |
Ferulic acid |
1.11 ± 0.12a |
1.70 ± 0.24b |
2.02 ± 0.18b |
1.30 ± 0.06b |
4.37 ± 0.23c |
Syringic acid |
0.40 ± 0.02a |
0.42 ± 0.07a |
0.46 ± 0.07a |
0.32 ± 0.16a |
0.53 ± 0.01a |
Flavonoids |
(+)-Catechin |
0.37 ± 0.08a |
0.64 ± 0.08b |
0.70 ± 0.15b |
0.79 ± 0.16b |
2.11 ± 0.12c |
Procyanidin B2 |
1.08 ± 0.03a |
1.21 ± 0.19a |
<LOQ |
<LOQ |
<LOQ |
Quercetin 3-O-rutinose-7-O-α-L-rhamnoside |
168.14 ± 10.20a |
147.70 ± 26.05a |
152.22 ± 12.45a |
134.27 ± 8.32a |
231.91 ± 15.62b |
Procyanidin A2 |
4.94 ± 0.43a |
4.03 ± 0.25a |
4.35 ± 0.25a |
3.32 ± 0.06a |
7.29 ± 0.32b |
Rutin |
8.24 ± 0.18a |
5.42 ± 0.32a |
5.59 ± 0.89a |
5.71 ± 0.75a |
20.15 ± 1.61b |
With respect to flavonoids, the presence of quercetin 3-O-rutinose-7-O-α-L-rhamnoside was predominant in all samples, followed by rutin and procyanidin A2. The detected (+)-catechin in LPP demonstrated a considerable increase (p < 0.05) in the contents after in vitro separate or successive gastrointestinal digestions compared to the initial value (undigested LPP), while this compound increased by 5.7-fold in content following SGID treatment. The procyanidin B2 contents in LPP were lower than the limit of quantitation after the SGD, SID or SGID treatments, albeit increasing in the SSD group. After the SSD, SID or SID treatments, the amounts of quercetin-3-rutinose-7-rhamnoside, procyanidin A2 and rutin were lower than those of the corresponding flavonoids in the undigested group. However, these flavonoids displayed higher contents in the SGID group compared with the other groups (p < 0.05).
Effect of LPP fermentation on phenolic composition
LPP was subjected to a colonic fermentation assay. Table 2 details the composition and variation in the LPP sample during this process. The vanillic acid content was 1.11-fold the initial value after 12 h of fermentation. However, vanillic acid was degraded after 12 h, resulting in no significant differences (p > 0.05) between its final and initial values. After 48 h of fermentation, contents of gallic acid, caffeic acid, ferulic acid and syringic acid decreased by 4.86%, 12.23%, 13.65% and 17.29%, respectively, compared to the corresponding initial values.
Table 2 The contents of the phenolic compounds in LPP fermentation products
Compound (mg per 100 g DW) |
0 h |
6 h |
12 h |
24 h |
48 h |
Values with different letters are significantly different (p < 0.05). |
Phenolic acids |
Gallic acid |
21.61 ± 0.09d |
21.10 ± 0.02c |
21.07 ± 0.01c |
20.82 ± 0.05b |
20.56 ± 0.02a |
Vanillic acid |
26.26 ± 4.62a |
28.89 ± 1.24a |
29.10 ± 0.28a |
28.19 ± 0.05a |
27.57 ± 0.85a |
Caffeic acid |
10.22 ± 0.10c |
9.79 ± 0.26b |
9.77 ± 0.01b |
9.20 ± 0.26a |
8.97 ± 0.01a |
Ferulic acid |
339.90 ± 2.69d |
332.25 ± 2.27c |
325.79 ± 4.82c |
312.18 ± 2.51b |
293.50 ± 3.03a |
Syringic acid |
12.49 ± 0.80c |
11.38 ± 0.30b |
10.17 ± 0.21a |
11.00 ± 0.16a |
10.33 ± 0.60a |
Flavonoids |
(+)-Catechin |
51.40 ± 6.11a |
55.68 ± 2.14a |
57.43 ± 0.06a |
58.70 ± 0.75a |
58.95 ± 1.54a |
Procyanidin B2 |
156.95 ± 6.02c |
145.06 ± 8.14bc |
138.14 ± 1.26b |
127.19 ± 2.27a |
121.87 ± 4.65a |
Quercetin 3-O-rutinose-7-O-α-L-rhamnoside |
18 499.66 ± 212.92c |
18 164.39 ± 399.78bc |
17 869.73 ± 122.13b |
16 950.56 ± 83.80a |
16 178.50 ± 660.71a |
Procyanidin A2 |
126.01 ± 1.62b |
125.52 ± 6.07a |
120.37 ± 7.16a |
116.15 ± 4.43a |
110.90 ± 5.48a |
Rutin |
1647.98 ± 73.14b |
1597.23 ± 98.44ab |
1585.25 ± 20.14b |
1522.56 ± 22.56a |
1433.77 ± 58.68a |
Interestingly, after 48 h of fermentation, a slight increase (14.69%) in the contents of (+)-catechin was noted, showing an opposite trend from the other flavonoid compounds. The degradation of procyanidin B2 and quercetin 3-O-rutinose-7-O-α-L-rhamnoside mainly occurred at 0–24 h, which resulted in 18.96% and 8.37% losses, respectively. For procyanidin A2 and rutin, their contents decreased during the whole process from 126.01 to 110.90 mg per 100 g DW and 1647.98 to 1522.56 mg per 100 g DW, respectively. In addition, there were no significant changes in the contents of all quantitative flavonoids during the final stages of fermentation (24–48 h).
Effect of LPP fermentation on the pH value and SCFA concentrations
To elucidate the fecal fermentation behaviors of LPP, the pH shift and SCFA concentrations were determined and are summarized in Fig. 2 and Table 3. At 0 h, the initial pH value of the control group was significantly lower than that of the LPP group (p < 0.05). Gradually, the pH values in the control group decreased from 4.20 to 3.60 over 48 h of fermentation, while a dramatic (p < 0.05) decrease in the pH value, from 4.56 to 3.57, was noticed for the LPP fermentation group.
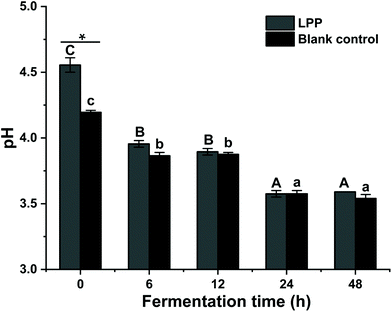 |
| Fig. 2 Changes in pH values during LPP fermentation with human feces. An asterisk (*) within the same treatment indicates a significant difference between LPP and the control (water) (p < 0.05). Bars with no letter in common are significantly different (p < 0.05). | |
Table 3 The concentrations of total and individual SCFA in LPP and control (water) fermentation products
|
Time (h) |
Control |
LPP |
0 |
48 |
0 |
48 |
Values with different letters are significantly different (p < 0.05). LOQ: limit of quantification; the LOQ value was 0.02 nmol mL−1 for i-valeric acid. |
SCFA (nmol mL−1) |
Acetic acid |
446.75 ± 0.35a |
482.57 ± 35.90a |
910.93 ± 51.44b |
1115.25 ± 21.41c |
Propionic acid |
76.45 ± 6.57b |
58.74 ± 9.79a |
67.40 ± 1.68b |
102.55 ± 3.89c |
i-Butyric acid |
31.35 ± 1.65a |
28.93 ± 4.01a |
41.60 ± 0.63b |
43.67 ± 1.01b |
Valeric acid |
115.31 ± 11.71a |
159.29 ± 10.45b |
129.54 ± 3.22a |
161.35 ± 2.11b |
i-Valeric acid |
19.94 ± 1.89b |
<LOQ |
19.54 ± 1.06b |
12.23 ± 4.68a |
Total |
689.8 ± 17.40a |
729.53 ± 40.35a |
1168.71 ± 56.73b |
1435.05 ± 32.35c |
The LPP group presented evidently (p < 0.05) higher concentrations in SCFA compared to the control group at the initial time and end of fermentation. Individually, the concentrations of acetic, i-butyric and valeric acids in the LPP group were much higher than those of the corresponding acids in the control group at 0 h, while an opposite trend was observed for propionic acid. In the control group, propionic acid and valeric acid showed significant changes (p < 0.05) after fermentation compared to their initial values. However, after 48 h of fermentation, the levels of acetic, propionic and valeric acids in the LPP group were markedly increased (p < 0.05), reaching up to 1.22-, 1.52- and 1.25-fold their initial values, respectively.
Fecal metabolic profiling of LPP-fed mice
After denoising, filtration of peaks with detection rates <50%, simulation of missing value recording and internal standard normalization, 2444 and 2285 peaks were detected in positive and negative ion modes, respectively. As a multivariate statistical analysis method, OPLS-DA could maximize the differences between groups. The OPLS-DA model showed that these two groups were obviously separated (Fig. 3). The parameters were R2Y = 0.999 and Q2 = 0.906 in positive ion mode and R2Y = 0.997 and Q2 = 0.869 in negative ion mode. Furthermore, the high reliability and good fitness of this model were validated through rigorous permutation tests (200 permutations), as shown in Fig. 3C and D. Among the detected metabolites, a total of 124 differentially expressed metabolites (41 upregulated and 83 downregulated) were found in positive ion mode while only 69 differentially expressed metabolites (21 upregulated and 48 downregulated) in negative ion mode by searching the in-house MS2, KEGG, and HMDB databases. The variations of differentially expressed metabolites are depicted in the heatmap for each treatment group (Fig. 4). Of note, linoleic and lithocholic acid levels were higher in the fecal contents of LPP-fed mice than in control group mice. Furthermore, higher levels of nicotinamide were found in the fecal contents of LPP-fed mice than in control group mice. In contrast, the levels of arachidonic acid, NAD, biotin and L-lysine were significantly down-regulated in the LPP group, compared with those in the control group. Based on the KEGG and MetaboAnalyst databases, annotation and enrichment analysis were conducted to search for metabolic pathways significantly affected by LPP, including linoleic acid metabolism, biotin metabolism, biosynthesis of unsaturated fatty acids and nicotinate and nicotinamide metabolism (Fig. 5).
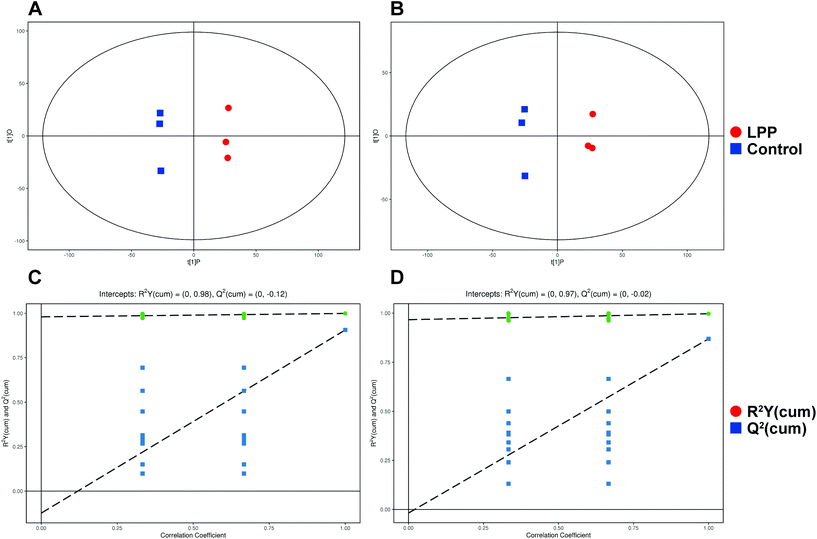 |
| Fig. 3 Multivariate analyses of fecal metabolic profiles of mice in the control and LPP groups. (A and B) OPLS-DA score plots of fecal metabolites in the control and LPP groups obtained in positive (A) and negative (B) ion modes. (C and D) Permutation test for the OPLS-DA model in positive (C) and negative (D) ion modes. | |
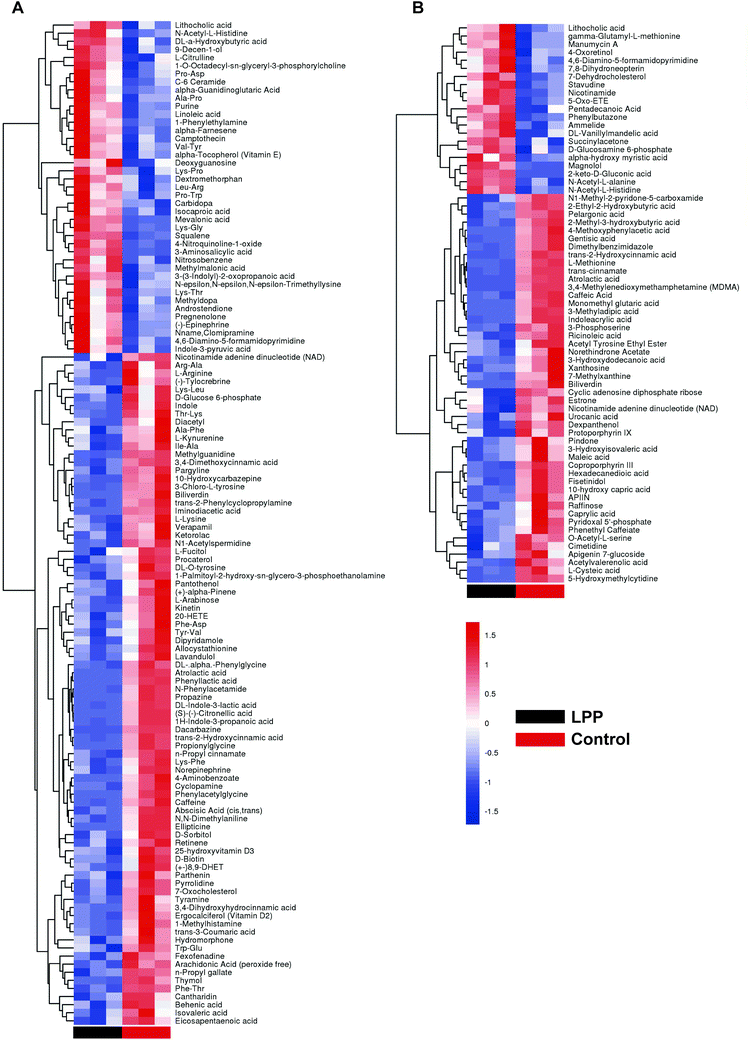 |
| Fig. 4 Heatmap showing different expression levels of significantly altered fecal metabolites between control and LPP groups in positive (A) and negative (B) ion modes. | |
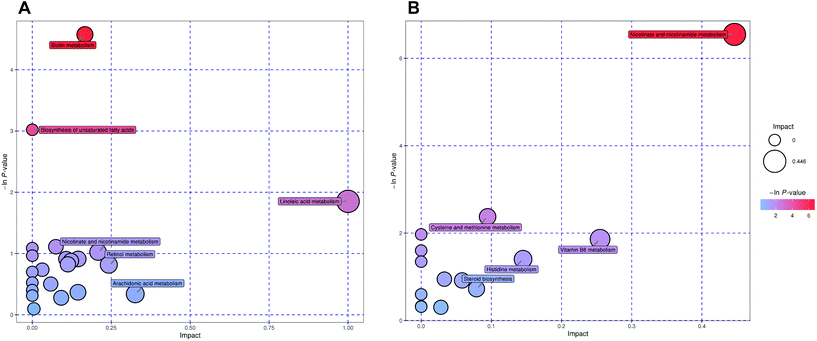 |
| Fig. 5 Summary of fecal sample pathway analysis in positive (A) and negative (B) ion modes using MetaboAnalyst. | |
Discussion
In the present study, the composition and variations in LPP during in vitro separate and successive gastrointestinal digestion (e.g., SSD, SGD, SID and SGID) were clarified; we also investigated the fecal metabolites and metabolic pathways altered by LPP.
To exert their bioactivities, phenolic compounds have to be solubilized in the digestive system, remaining stable until being absorbed. The degradation of phenolic compounds after SSD, SGD and SID treatments has been widely reported, such as with grape polyphenols,21 apple bagasse flours22 and Madeiran elderberry.23 The different pH conditions may lead to phenolic and flavonoid degradation or polymerization with enzymes by forming covalent or noncovalent bonds, and thus a decrease in their extractability or solubility.24 Another factor affecting release and stability is the complexity of the phenolic structure.25 TPC and TFC values of LPP presented a small decrease after SSD compared to undigested LPP. This result could be explained by the change in the physicochemical environment and the marginal impact from α-amylase as mentioned by Mosele et al.26 Manach et al.27 studied food sources and the bioavailability of polyphenols, pointing out that the gastric digestion does not affect the structures of most phenolic compounds significantly. Another work considered that phenolics are unstable in an alkaline or neutral environment, as is the case in intestinal condition, exhibiting weak resistance relative to the gastric environment.28 Thus, this may account for the highest TPC obtained after SGD treatment among the three separate gastrointestinal digestion treatments. Interestingly, the TPC and TFC values of LPP after SGID were higher than those of undigested LPP, indicating no significant degradation of phenolic compounds, despite the dramatic loss under the oral, gastric and intestinal conditions. According to these results, we could deduce that the increased release of phenolic compounds is highly dependent on the synergy of digestive enzymes at three digestive stages. It was also pointed out that the bio-accessibility of polyphenols is highly related to functional enzymes during digestion, and more specifically, at the intestinal stage.24 According to our previous work,29 LPP powder under study contains small amounts of dietary fiber which is probably bound with phenolics. Therefore, the fractures of non-covalent and covalent bonds between dietary fiber and phenolics could be another reason for increments in phenolic contents and antioxidant activities after SGID treatment.30,31 Additionally, using correlation analysis, a linear relationship was found between TPC and FRAP (r2 = 0.978, p < 0.05) and between TPC and ORAC (r2 = 0.965, p < 0.05), suggesting that antioxidant activity was highly correlated with the release of phenolic compounds.
The contents of monomeric phenolics, such as gallic acid, ferulic acid and (+)-catechin, showed an increase after separate or successive digestion compared to undigested LPP. This result was supported by the study of Chait et al.8 who demonstrated an increment in the levels of carob phenolic acids after gastrointestinal digestion. This is mainly a consequence of the following two factors: (i) hydrolysis of esterified phenolics and soluble conjugated phenolics leading to an increase in the contents of monomeric phenolics8 and (ii) isomerization in phenolic structures due to enzymatic actions affecting their solubility.24 For example, under gastric and intestinal digestion conditions, procyanidin B2 was completely degraded and transformed into gallocatechin, which further hydrolyzed and generated gallic acid and (+)-catechin.32 This may be one of the reasons accounting for the increase in gallic acid and (+)-catechin contents after in vitro separate or successive digestion. In addition, it should be pointed out that dehydration caused by freeze-drying in the preparation of LPP samples may also lead to the generation of esterified phenolics (e.g., gallocatechin) or soluble conjugates that can be hydrolyzed under acidic conditions.33 Another study reported that intestinal solutions may result in phenolics with more hydroxyl groups, leading to the degradation of phenolics.11 This was consistent with our result, as we observed 20.14% losses in quercetin 3-O-rutinose-7-O-α-L-rhamnoside and 32.79% losses in procyanidin A2 after SID treatment. Rutin showed large increases under SGID conditions, which may be related to the hydrolysis of complex compounds due to functional enzymes.34
After gastrointestinal digestion, the undigested phenolics may reach the colon where they could be utilized by the microbiota, resulting in the generation of new metabolites, and these new metabolites may not be included in the ten detected phenolic compounds. The main microbiota catabolic pathways include hydrolysis, ring cleavage and dihydroxylation.35 Although a previous study reported that gallic acid could be transformed into catechol (1,2-dihydroxy phenol),36 this metabolite was not found in the present study. Quercetin 3-O-rutinose-7-O-α-L-rhamnoside and rutin could be catabolized into quercetin by fecal microbial enzymes (e.g., α-rhamnosidase) in Bacteroides uniformis, resulting in the formation of aglycon. Furthermore, quercetin was converted to 2-(3,4-dihydroxy phenyl) acetic acid or vanillic acid due to ring cleavage.37 This may account for the decreased quercetin 3-O-rutinose-7-O-α-L-rhamnoside and rutin contents after 48 h of fermentation and the highest vallinic acid contents at 12 h of fermentation. Procyanidins are oligomers and polymers of flavan3-ols with different degrees of polymerization. Tomas-Barberan et al.38 considered that catechin and gallate derivatives are common catabolites of procyanidins. The (+)-catechin contents showed an increasing trend throughout the fermentation period. Therefore, a portion of (+)-catechin detected in the fermentation supernatants was probably transformed from procyanidin B2 or procyanidin A2. More information about phenolic catabolites from LPP requires further research. On the other hand, it has been reported that the levels of SCFA preliminarily reflect the growth of gut microbes.38 As the production of saccharolytic fermentation, SCFA also have regulating effects on the richness and evenness of the microbiota.39 In this study, the concentrations of various quantitative SCFA in the LPP group were all higher than those in the control group, indicating that LPP could modulate their biosynthesis, even though only small amounts of phenolic compounds were utilized. In addition, acetic acid was produced mainly by Bifidobacteria, through the fructose-6-phosphate phosphoketolase pathway,40 inhibiting DNA oxidative damage in isolated mouse distal colon cells.41 Propionic acid exhibits excellent effects on maintaining normal colonic cell phenotypes.22 Therefore, these results supported that LPP may exert a beneficial effect on gut function by producing higher amounts of SCFA.
Herein, our metabolomic analysis found that LPP modulated linoleic acid metabolism and the biosynthesis of unsaturated fatty acids in mice by upregulating the levels of linoleic and arachidonic acid. Their levels were highly associated with the production of pro-inflammatory and anti-inflammatory eicosanoids.42 Linoleic acid has various functions, such as being an essential component of ceramides and maintaining the membrane fluidity of the epidermis.43,44 It has been reported that fatty acids maintain the integrity of intestinal barrier through the GPR40-MEK-ERK pathway, with linoleic acid displaying the excellent affinity for free fatty acid receptor 1.45 Therefore, LPP may maintain the integrity of the gut barrier by promoting linoleic acid metabolism and the biosynthesis of unsaturated fatty acids. The increase in the levels of lithocholic acid in the LPP-fed mice feces was probably related to microbes in the colon, and it may exhibit a significant effect on inducing cancer cell apoptosis.46 In addition, it has been demonstrated that biotin metabolism was associated with gut barrier function in a previous study.47 Significant depletion of NAD together with an increase in nicotinamide was observed in the LPP group compared to the control group, which was consistent with a previous study48 and suggested that LPP may impact energy expenditure. Kim et al.49 pointed out that nicotinamide upregulation might be one mechanism regulating the integrity of intestinal barrier, as it could suppress epithelial cell apoptosis and the synthesis of inflammatory cytokines. As reported,50 the imbalance of energy and lipid metabolism will increase intestinal oxidative stress, resulting in the destruction of the intestinal barrier. Hence, the regulation of NAD and nicotinamide metabolism may be one of the molecular mechanisms underlying the protective effect of LPP on the intestinal barrier. Based on the in vitro and in vivo results, it can be deduced that LPP may exhibit a positive effect on regulating fatty acid metabolism in the digestive tract and promoting the integrity of gut barrier. To further elucidate the prebiotic functions of LPP, further studies are ongoing to examine the effects of chronic LPP treatment on bacterial flora distribution in the colon and ameliorating gut inflammation, especially assuming different levels of LPP intake.
Conclusion
In summary, the comparison of phenolic contents after separated and successive digestions firstly confirmed that the incremental release of phenolic compounds originated from the synergistic effect of enzymes associated with three digestive stages. Procyanidin B2 displayed a dramatic degradation and transformed to gallic acid and (+)-catechin under gastric, intestinal, successive gastrointestinal digestion and colonic fermentation conditions. During colonic fermentation, LPP induced SCFA biosynthesis leading to a decrease in pH values. Furthermore, fecal metabolomics of mice confirmed that LPP mainly affected three metabolic pathways, including unsaturated fatty acid, biotin, and nicotinamide metabolism which are associated with the integrity of the gut barrier. Taken together, these results suggest that LPP might be a beneficial food supplement for regulating lipid metabolism and improving gut health.
Conflicts of interest
The authors declare that they have no competing interests.
Acknowledgements
This project was supported by the National Natural Science Foundation of China (Grant No: 31601469) and the Science and Technology Program of Guangzhou (Grant No: 201604020089).
References
- X. Xu, H. Xie, J. Hao, Y. Jiang and X. Wei, Eudesmane sesquiterpene glucosides from lychee seed and their cytotoxic activity, Food Chem., 2010, 123, 1123–1126 CrossRef CAS.
- R. F. Tang, Y. J. Zhou, Z. S. Z. Chen, L. Wang, Y. K. Lai, S. K. Chang, Y. F. Wang, H. X. Qu, Y. M. Jiang and H. Huang, Regulation of browning and senescence of litchi fruit mediated by phenolics and energy status: A postharvest comparison on three different cultivars, Postharvest Biol. Technol., 2020, 168, 9 Search PubMed.
- S. Emanuele, M. Lauricella, G. Calvaruso, A. D'Anneo and M. Giuliano, Litchi chinensis as a Functional Food and a Source of Antitumor Compounds: An Overview and a Description of Biochemical Pathways, Nutrients, 2017, 9, 992 CrossRef.
- D. Su, R. Zhang, F. Hou, J. Chi, F. Huang, S. Yan, L. Liu, Y. Deng, Z. Wei and M. Zhang, Lychee pulp phenolics ameliorate hepatic lipid accumulation by reducing miR-33 and miR-122 expression in mice fed a high-fat diet, Food Funct., 2017, 8, 808–815 RSC.
- R. Yamanishi, E. Yoshigai, T. Okuyama, M. Mori, H. Murase, T. Machida, T. Okumura and M. Nishizawa, The Anti-Inflammatory Effects of Flavanol-Rich Lychee Fruit Extract in Rat Hepatocytes, PLoS One, 2014, 9, e93818 CrossRef.
- S. R. M. Ibrahim and G. A. Mohamed, Litchi chinensis: medicinal uses, phytochemistry, and pharmacology, J. Ethnopharmacol., 2015, 174, 492–513 CrossRef CAS.
- D. Su, H. Ti, R. Zhang, M. Zhang, Z. Wei, Y. Deng and J. Guo, Structural elucidation and cellular antioxidant activity evaluation of major antioxidant phenolics in lychee pulp, Food Chem., 2014, 158, 385–391 CrossRef CAS.
- Y. A. Chait, A. Gunenc, F. Bendali and F. Hosseinian, Simulated gastrointestinal digestion and in vitro colonic fermentation of carob polyphenols: Bioaccessibility and bioactivity, LWT–Food Sci. Technol., 2020, 117, 108623 CrossRef CAS.
- M. Alminger, A. M. Aura, T. Bohn, C. Dufour, S. N. El, A. Gomes, S. Karakaya, M. C. Martinez-Cuesta, G. J. McDougall, T. Requena and C. N. Santos, In Vitro Models for Studying Secondary Plant Metabolite Digestion and Bioaccessibility, Compr. Rev. Food Sci. Food Saf., 2014, 13, 413–436 CrossRef CAS.
- T. Bohn, F. Carriere, L. Day, A. Deglaire, L. Egger, D. Freitas, M. Golding, S. Le Feunteun, A. Macierzanka, O. Menard, B. Miralles, A. Moscovici, R. Portmann, I. Recio, D. Remond, V. Sante-Lhoutelier, T. J. Wooster, U. Lesmes, A. R. Mackie and D. Dupont, Correlation between in vitro and in vivo data on food digestion. What can we predict with static in vitro digestion models?, Crit. Rev. Food Sci. Nutr., 2018, 58, 2239–2261 CrossRef CAS.
- D. Su, M. Luo, H. Liu, X. Qi, Q. Zeng, S. He, S. Fen and J. Zhang, The effect of simulated digestion on the composition of phenolic compounds and antioxidant activities in lychee pulp of different cultivars, Int. J. Food Sci. Technol., 2019, 54, 3042–3050 CrossRef CAS.
- A. Jain, X. H. Li and W. N. Chen, An untargeted fecal and urine metabolomics analysis of the interplay between the gut microbiome, diet and human metabolism in Indian and Chinese adults, Sci. Rep., 2019, 9, 13 CrossRef.
- Y. Zhou, L. Men, Z. Pi, M. Wei, F. Song, C. Zhao and Z. Liu, Fecal Metabolomics of Type 2 Diabetic Rats and Treatment with Gardenia jasminoides Ellis Based on Mass Spectrometry Technique, J. Agric. Food Chem., 2018, 66, 1591–1599 CrossRef CAS.
- I. Juaniz, I. A. Ludwig, L. Bresciani, M. Dall'Asta, P. Mena, D. Del Rio, C. Cid and M.-P. de Pena, Bioaccessibility of (poly)phenolic compounds of raw and cooked cardoon (Cynara cardunculus L.) after simulated gastrointestinal digestion and fermentation by human colonic microbiota, J. Funct. Foods, 2017, 32, 195–207 CrossRef CAS.
- Z. Dou, C. Chen and X. Fu, Bioaccessibility, antioxidant activity and modulation effect on gut microbiota of bioactive compounds from Moringa oleifera Lam. leaves during digestion and fermentation in vitro, Food Funct., 2019, 10, 5070–5079 RSC.
- I. Gil-Sanchez, C. Cueva, M. Sanz-Buenhombre, A. Guadarrama, M. V. Moreno-Arribas and B. Bartolome, Dynamic gastrointestinal digestion of grape pomace extracts: Bioaccessible phenolic metabolites and impact on human gut microbiota, J. Food Compos. Anal., 2018, 68, 41–52 CrossRef CAS.
- A. S. Mohamed, M. Hosney, H. Bassiony, S. S. Hassanein, A. M. Soliman, S. R. Fahmy and K. Gaafar, Sodium pentobarbital dosages for exsanguination affect biochemical, molecular and histological measurements in rats, Sci. Rep., 2020, 10, 378 CrossRef CAS.
- G. Das, A. Vernunft, S. Gors, E. Kanitz, J. M. Weitzel, K. P. Brussow and C. C. Metges, Acute effects of general anesthesia with propofol, pentobarbital or isoflurane plus propofol on plasma metabolites and hormones in adult pigs, J. Anim. Sci., 2016, 94, 5182–5191 CrossRef CAS.
- R. Johnson, J. F. Fowler and G. D. Zanelli, Changes in mouse blood pressure, tumor blood flow, and core and tumor temperatures following nembutal or urethane anesthesia, Radiology, 1976, 118, 697–703 CrossRef CAS.
- J. L. Wang, T. Zhang, X. T. Shen, J. Liu, D. L. Zhao, Y. W. Sun, L. Wang, Y. J. Liu, X. Y. Gong, Y. X. Liu, Z. J. Zhu and F. Z. Xue, Serum metabolomics for early diagnosis of esophageal squamous cell carcinoma by UHPLC-QTOF/MS, Metabolomics, 2016, 12, 116–124 CrossRef.
- D. Tagliazucchi, E. Verzelloni, D. Bertolini and A. Conte, In vitro bio-accessibility and antioxidant activity of grape polyphenols, Food Chem., 2010, 120, 599–606 CrossRef CAS.
- B. Gullon, M. E. Pintado, X. Barber, J. Fernandez-Lopez, J. A. Perez-Alvarez and M. Viuda-Martos, Bioaccessibility, changes in the antioxidant potential and colonic fermentation of date pits and apple bagasse flours obtained from co-products during simulated in vitro gastrointestinal digestion, Food Res. Int., 2015, 78, 169–176 CrossRef CAS.
- J. Pinto, V. Spinola, E. J. Llorent-Martinez, M. L. Fernandez-de Cordova, L. Molina-Garcia and P. C. Castilho, Polyphenolic profile and antioxidant activities of Madeiran elderberry (Sambucus lanceolata) as affected by simulated in vitro digestion, Food Res. Int., 2017, 100, 404–410 CrossRef CAS.
- S. Ketnawa, J. Suwannachot and Y. Ogawa, In vitro gastrointestinal digestion of crisphead lettuce: Changes in bioactive compounds and antioxidant potential, Food Chem., 2020, 311, 125885 CrossRef CAS.
- D. Cianciosi, T. Yuliett Forbes-Hernandez, S. Afrin, M. Gasparrini, J. L. Quiles, E. Gil, S. Bompadre, J. Simal-Gandara, M. Battino and F. Giampieri, The Influence of In Vitro Gastrointestinal Digestion on the Anticancer Activity of Manuka Honey, Antioxidants, 2020, 9, 64 CrossRef CAS.
- J. I. Mosele, A. Macia, M. P. Romero and M. J. Motilva, Stability and metabolism of Arbutus unedo bioactive compounds (phenolics and antioxidants) under in vitro digestion and colonic fermentation, Food Chem., 2016, 201, 120–130 CrossRef CAS.
- C. Manach, A. Scalbert, C. Morand, C. Remesy and L. Jimenez, Polyphenols: food sources and bioavailability, Am. J. Clin. Nutr., 2004, 79, 727–747 CrossRef CAS.
- M. S. Lingua, D. A. Wunderlin and M. V. Baroni, Effect of simulated digestion on the phenolic components of red grapes and their corresponding wines, J. Funct. Foods, 2018, 44, 86–94 CrossRef CAS.
- D. Su, R. Zhang, C. Zhang, F. Huang, J. Xiao, Y. Deng, Z. Wei, Y. Zhang, J. Chi and M. Zhang, Phenolic-rich lychee (Litchi chinensis Sonn.) pulp extracts offer hepatoprotection against restraint stress-induced liver injury in mice by modulating mitochondrial dysfunction, Food Funct., 2016, 7, 508–515 RSC.
- M. Tomas, G. Rocchetti, S. Ghisoni, G. Giuberti, E. Capanoglu and L. Lucini, Effect of different soluble dietary fibres on the phenolic profile of blackberry puree subjected to in vitro gastrointestinal digestion and large intestine fermentation, Food Res. Int., 2020, 130, 10 CrossRef.
- L. Cervantes, E. Martinez-Ferri, C. Soria and M. Teresa Ariza, Bioavailability of phenolic compounds in strawberry, raspberry and blueberry: Insights for breeding programs, Food Biosci., 2020, 37, 100680 CrossRef CAS.
- K. Kahle, M. Kempf, P. Schreier, W. Scheppach, D. Schrenk, T. Kautenburger, D. Hecker, W. Huemmer, M. Ackermann and E. Richling, Intestinal transit and systemic metabolism of apple polyphenols, Eur. J. Nutr., 2011, 50, 507–522 CrossRef CAS.
- K. O. Pimenta Inada, S. Nunes, J. Alberto Martinez-Blazquez, F. A. Tomas-Barberan, D. Perrone and M. Monteiro, Effect of high hydrostatic pressure and drying methods on phenolic compounds profile of jabuticaba (Myrciaria jaboticaba) peel and seed, Food Chem., 2020, 309, 125794 CrossRef CAS.
- N. Ortega, A. Macia, M.-P. Romero, J. Reguant and M.-J. Motilva, Matrix composition effect on the digestibility of carob flour phenols by an in vitro digestion model, Food Chem., 2011, 124, 65–71 CrossRef CAS.
- J. Carlos Espin, A. Gonzalez-Sarrias and F. A. Tomas-Barberan, The gut microbiota: A key factor in the therapeutic effects of (poly) phenols, Biochem. Pharmacol., 2017, 139, 82–93 CrossRef.
- K. Hanhineva, R. Torronen, I. Bondia-Pons, J. Pekkinen, M. Kolehmainen, H. Mykkanan and K. Poutanen, Impact of Dietary Polyphenols on Carbohydrate Metabolism, Int. J. Mol. Sci., 2010, 11, 1365–1402 CrossRef CAS.
- M. V. Selma, J. C. Espin and F. A. Tomas-Barberan, Interaction between Phenolics and Gut Microbiota: Role in Human Health, J. Agric. Food Chem., 2009, 57, 6485–6501 CrossRef CAS.
- F. A. Tomas-Barberan and J. C. Espin, Effect of Food Structure and Processing on (Poly)phenol-Gut Microbiota Interactions and the Effects on Human Health, Annu. Rev. Food Sci. Technol., 2019, 10, 221–238 CrossRef CAS.
- K. B. Guergoletto, A. Costabile, G. Flores, S. Garcia and G. R. Gibson, In vitro fermentation of jucara pulp (Euterpe edulis) by human colonic microbiota, Food Chem., 2016, 196, 251–258 CrossRef CAS.
- R. M. Alqurashi, S. N. Alarifi, G. E. Walton, A. F. Costabile, I. R. Rowland and D. M. Commane, In vitro approaches to assess the effects of acai (Euterpe oleracea) digestion on polyphenol availability and the subsequent impact on the faecal microbiota, Food Chem., 2017, 234, 190–198 CrossRef CAS.
- D. L. Topping and P. M. Clifton, Short-chain fatty acids and human colonic function: Roles of resistant starch and nonstarch polysaccharides, Physiol. Rev., 2001, 81, 1031–1064 CrossRef CAS.
- S. H. Piao, Z. Q. Zhu, S. Y. Tan, H. X. Zhan, X. L. Rong and J. Guo, An integrated fecal microbiome and metabolome in the aged mice reveal anti-aging effects from the intestines and biochemical mechanism of FuFang zhenshu TiaoZhi(FTZ), Biomed. Pharmacother., 2020, 121, 10 Search PubMed.
- J. Whelan and K. Fritsche, Linoleic Acid, Adv. Nutr., 2013, 4, 311–312 CrossRef.
- M. A. Carabajal, G. Viarengo, L. Yim, A. Martinez-Sanguine, J. F. Mariscotti, J. A. Chabalgoity, R. M. Rasia and E. G. Vescovi, PhoQ is an unsaturated fatty acid receptor that fine-tunes Salmonella pathogenic traits, Sci. Signaling, 2020, 13, eaaz3334 CrossRef.
- S. S. Cheng, X. Ma, S. J. Geng, X. M. Jiang, Y. Li, L. S. Hu, J. R. Li, Y. Z. Wang and X. Y. Han, Fecal Microbiota Transplantation Beneficially Regulates Intestinal Mucosal Autophagy and Alleviates Gut Barrier Injury, mSystems, 2018, 3, 19 CrossRef.
- T. H. Luu, J. M. Bard, D. Carbonnelle, C. Chaillou, J. M. Huvelin, C. Bobin-Dubigeon and H. Nazih, Lithocholic bile acid inhibits lipogenesis and induces apoptosis in breast cancer cells, Cell. Oncol., 2018, 41, 13–24 CrossRef CAS.
- J. Skupsky, S. Sabui, M. Nakasaki, M. Hwang, J. N. Chen, M. Cahalan and H. M. Said, Biotin supplementation ameliorates murine colitis by maintaining intestinal mucosal integrity, Gastroenterology, 2019, 156, S623–S623 CrossRef.
- M. Yu, H.-M. Jia, C. Zhou, Y. Yang, L.-L. Sun and Z.-M. Zou, Urinary and Fecal Metabonomics Study of the Protective Effect of Chaihu-Shu-Gan-San on Antibiotic-Induced Gut Microbiota Dysbiosis in Rats, Sci. Rep., 2017, 7, 46551 CrossRef CAS.
- Y. Kim, S. W. Hwang, S. Kim, Y. S. Lee, T. Y. Kim, S. H. Lee, S. J. Kim, H. J. Yoo, E. N. Kim and M. N. Kweon, Dietary cellulose prevents gut inflammation by modulating lipid metabolism and gut microbiota, Gut Microbes, 2020, 11, 944–961 CrossRef.
- E. Cremonini, E. Daveri, A. Mastaloudis, A. M. Adamo, D. Mills, K. Kalanetra, S. N. Hester, S. M. Wood, C. G. Fraga and P. I. Oteiza, Anthocyanins protect the gastrointestinal tract from high fat diet-induced alterations in redox signaling, barrier integrity and dysbiosis, Redox Biol., 2019, 26, 101269 CrossRef CAS.
Footnotes |
† Electronic supplementary information (ESI) available. See DOI: 10.1039/d0fo02319a |
‡ These two authors should be considered joint first authors. |
|
This journal is © The Royal Society of Chemistry 2021 |
Click here to see how this site uses Cookies. View our privacy policy here.