DOI:
10.1039/D0FO02337J
(Paper)
Food Funct., 2021,
12, 154-161
β-Conglycinin induces the formation of neutrophil extracellular traps dependent on NADPH oxidase-derived ROS, PAD4, ERK1/2 and p38 signaling pathways in mice
Received
3rd September 2020
, Accepted 11th November 2020
First published on 16th November 2020
Abstract
β-Conglycinin is one of the key thermostable anti-nutritional factors in soybean, which has strong immunogenicity that usually leads to weaning in some young animals such as piglets and calves and allergic reaction in rats. Neutrophils are involved in the pathogenesis of an allergy. However, the contribution of functional neutrophils to allergy needs to be clarified. The formation of neutrophil extracellular traps is a novel effector mechanism of neutrophils and has been extensively investigated in recent years. To the best of our knowledge, there is no information available on β-conglycinin-induced NETs. In this study, β-conglycinin-induced NET formation in mice was examined via immunofluorescence analysis and fluorescence microplate reader. The mechanism of β-conglycinin-induced NETs was investigated using inhibitors and fluorescent microplate methods. The results showed that β-conglycinin induced the classical characteristics of NETs, which mainly consist of DNA as the backbone and decorated with histones, myeloperoxidase (MPO) and neutrophil elastase (NE). Moreover, β-conglycinin significantly induced the formation of NETs in a dose-dependent way. NET degrading enzyme DNase I markedly reduced β-conglycinin-induced NETs, which suggests that β-conglycinin indeed triggered the release of NETs. Further investigation showed that the quantitation of NETs was markedly decreased by the inhibitors of reactive oxygen species (ROS)-derived-NADPH oxidase, ERK1/2, p38, Rac and PAD4 signaling pathways, indicating the crucial role of these signaling pathways in β-conglycinin-induced NETs. Furthermore, our findings revealed that β-conglycinin induced the formation of NETs, which is dependent on NADPH oxidase-derived ROS, ERK1/2, p38, Rac and PAD4 signaling pathways. This study is the first to demonstrate the underlying mechanisms of β-conglycinin-induced NET formation, and it could be helpful to understand diarrhea caused by β-conglycinin overexposure in young animals and provides the corresponding theoretical basis for clinical applications.
Introduction
Soybean has always been a good vegetable protein source for livestock in light of its nutrient-rich characteristics. Nevertheless, soybean also contains antigenic proteins that cause allergic reactions in young animals.1,2 Most of the commercial soybean protein products currently in contact are defatted soybean protein products, and the main raw material source is the low temperature defatted soybean meal. Soybean antigens refer to some substances in the soybean and its products that cause allergic reactions in humans and animals, among which β-conglycinin and glycinin are the most important allergens due to their thermal stability, anti-enzymatic hydrolysis, acid and alkali resistance, and other characteristics.3–5 The antigenic activity of β-conglycinin could not be destroyed during the processing of soybean meal. Therefore, with the entry into the gastrointestinal tract, its overexposure leads to the abnormal digestion and inflammatory reaction of intestinal mucosa in young animals,3,6 subsequently causing allergic diarrhea in young animals clinically and resulting in decreased performance and damage to the benefits of the aquaculture industry.
Polymorphonuclear leukocytes (PMNs) are crucial in non-specific immunity and constitute the first line of defense against foreign matter invasion in the body7,8 by well-known effector mechanisms such as phagocytosis and degranulation.9–11 In 2004, Brinkmann et al. found that PMNs had another means of resistance to pathogens, that is, smooth and extended mesh-like structures were released outside the cell to capture and kill pathogens.12,13 The main skeleton of this network structure is DNA accompanied by histones and antimicrobial peptides released from neutrophils. This network structure is called neutrophil extracellular traps (NETs), and NET formation is often associated with the death of neutrophils;13,14 therefore, the process of NET formation is called “NETosis”,9,11 which is a process different from apoptosis. It is similar to the programmed death pathway characterized by changes in the nuclear structure and capture of particles.8
Although NETs play a positive role in resisting pathogen invasion, pathogens also escape NETs by various means, and NETs themselves are like double-edged swords.7 Similar to all inflammatory reactions, if the formation of NETs is out of control, it will participate in the development of numerous diseases, causingsevere damage to the body. Studies showed that neutrophils not only kill pathogenic microorganisms but also cause damage to the body.15–17 DNase I in serum degrade NETs in the blood, but some auto-antibodies would be produced in the body if this process was blocked, and these auto-antibodies are closely related to certain autoimmune diseases, such as systemic lupus erythematosus.16,17 In addition, it is known that the NET formation is closely related to ROS and peptidyl arginine deiminase 4 (PAD4). ROS could active the mitogen-activated protein kinase (MAPK) signaling pathway, which stimulates downstream ERK1/2 and p38 via numerous cascade reactions to promote the formation of NETs.
β-Conglycinin is an allergenic antigen contained in soybeans that usually induces allergies and intestinal damage in animals and human beings. Neutrophils are involved in the pathogenesis of allergy. However, the contribution of functional neutrophils in allergy needs to be clarified. The formation of neutrophil extracellular traps is a novel effector mechanism of neutrophils and has been extensively investigated in recent years. To the best of our knowledge, there is no information available on β-conglycinin-induced NETs. Therefore, we speculated that β-conglycinin would induce the formation of NETs, which possibly play an important role in the β-conglycinin-stimulated allergy. In this study, we first investigated the effects of β-conglycinin on the emergence of NETs and then explored its underlying molecular mechanisms.
Materials and methods
Chemicals and reagents
β-Conglycinin (purity ≥ 98%) was bought from Changchun Huacheng Biotechnology Co., Ltd Zymosan. 2,7-Dichlorodihydrofluorescein diacetate (DCFH-DA), DNase I, diphenyleneiodonium chloride (DPI), SB202190, U0126 and poly-L-lysine were purchased from Sigma-Aldrich. NSC23766 was obtained from MedChem Express. Cl-Amidine was provided by Selleck. A lactate dehydrogenase (LDH) release assay kit was acquired from Beyotime Biotechnology. Myeloperoxidase (MPO) antibody (FITC) (Orb16003, Biorbyt), rabbit anti-neutrophil elastase (NE) antibody (ab68672, Abcam) and rabbit anti-mouse Ig-G-Fc/FITC (bs-0377R-FITC, Bioss) were used. Sytox Orange and Pico Green were purchased from Invitrogen. Anti-p-p38 (Cell Signaling Technology, Inc.), anti-p-ERK (Cell Signaling Technology, Inc.), anti-p38 (Bs4766, Bioword), anti-ERK1/2 (Bs3627, Bioword), anti-β-actin antibody (66
009-1-Ig, Proteintech), anti-CD11b (EPR1344, Abcam) and goat anti-rabbit IgG (H + L) HRP (BS13278, Bioword) were used.
Isolation of PMNs
Female BALB/c mice (25–30 g) were bought from Liaoning Changsheng Biotechnology Co., Ltd (Certificate SCXK2010-0001; Liaoning, China), and housed under 12 h light and 12 h dark-protected cycling conditions with the temperature at 24 ± 1 °C and the relative humidity of 50% ± 10%. Blood from mice was collected using blood tubes containing heparin, and 15 mL was used to isolate PMNs in each experiment according to the instructions of a mouse PMN isolation kit® (TianJin HaoYang Biological Manufacture Co. China). Obtained PMNs were resuspended in a phenol-free red 1640 medium (Gibco) for further use. All animal experiments were approved by the Institutional Animal Care and Use Ethics Committee at Jilin University (approval ID: SY201905017).
Confocal microscopy analyses
PMNs were incubated with β-conglycinin (0.25, 0.5, 1 mg mL−1, the concentration of β-conglycinin used in vitro studies is within the range that could reasonably be expected to be encountered in vivo) for 90 min on coverslips pre-treated by poly-L-lysine (0.1 mg mL−1) at 37 °C. After stimulation, the samples were fixed with 4% paraformaldehyde for 20 min. Then, they were washed three times with PBS for 5 min each time. After permeabilization with 0.2% Triton for 20 min, followed by two washes with PBS, the samples were incubated for 2 h with blocking buffer (3% goat serum) at room temperature. After that, the samples were incubated with anti-MPO or anti-NE antibody (1
:
200) overnight at 4 °C, and subsequently incubated for 2 h with a secondary rabbit anti-mouse Ig-G-Fc/FITC antibody (1
:
200) at room temperature. After being stained with 5 μM Sytox Orange (dissolved in PBS, 1
:
1000) for 15 min, the samples were observed and analyzed under a confocal microscope (Olympus FluoView FV1000).
NET quantification
NET quantification was carried out based on Pico® Green-derived fluorescence intensities using a multi-plate reader. Briefly, PMNs were evenly distributed into a 96-well plate and stimulated with β-conglycinin (0.25, 0.5, 1 mg mL−1) for 90 min at 37 °C. For investigating the mechanisms of NET formation, the following inhibitors were used: the NAPDH oxidase-inhibitor DPI (10 μM), the PAD4-inhibitor cl-amidine (6 μM), the ERK1/2 inhibitor U0126 (50 μM), SB202190 (10 μM) as an inhibitor of p38 MAPK, and NSC23766 (200 μM) as an inhibitor of Rac. PMNs were pretreated with these inhibitors (30 min, 37 °C), and then incubated with β-conglycinin (1 mg mL−1, 90 min, 37 °C). Finally, the plate was read with a fluorescent microplate reader Infiniti M200® (TECAN, Austria), excited at 485 nm, and emitted at 535 nm. Zymosan (1 mg mL−1) was used as a positive control. In addition, in order to prevent the influence of β-conglycinin itself on the fluorescence value, we pretreated PMNs with DNase I (1 mg mL−1) to confirm that it was β-conglycinin that stimulated the formation of NETs.
Detection of reactive oxygen species (ROS)
The β-conglycinin-stimulated ROS levels were detected by DCFH-DA (Sigma-Aldrich). Briefly, PMNs were incubated with DCFH-DA (10 μM) for 30 min. Next, PMNs were stimulated with β-conglycinin (0.25, 0.5, 1 mg mL−1) for 90 min after washing two times with RPMI 1640 (without phenol red). Then, the fluorescence values were detected on a fluorometric reader Infiniti M200® (TECAN, Austria). Zymosan (1 mg mL−1) was used as a positive control. For the inhibitor experiment, after incubation of 30 min with DCFH-DA (10 μM), inhibitor DPI (10 μM) was added into the wells, and then PMNs were incubated with β-conglycinin (1 mg mL−1, 90 min, 37 °C). Finally, the samples were detected with a fluorometric reader Infiniti M200® (TECAN, Austria) at 485 nm excitation wavelength and 535 nm emission wavelength.
Western blotting
PMNs were incubated with β-conglycinin (0.25, 0.5, and 1 mg mL−1) at 37 °C for 90 min. The total protein was extracted from PMNs, and the concentration was measured using the Micro BCA™ Protein Assay Kit (Thermo Fisher Scientific, USA). SDS-PAGE was performed to separate proteins, followed by transference to (polyvinylidene fluoride) PVDF membranes. The membranes were blocked with 5% BSA for 3 h at room temperature and subsequently incubated with the primary antibody overnight at 4 °C. The following primary antibodies were used: anti-p-p38 (1
:
1000), p-ERK1/2 (1
:
1000), p38 (1
:
1000), ERK1/2 (1
:
1000), and CD11b (1
:
500). Then, the membranes were washed three times with TBST for 10 min each time and were incubated with the secondary antibody (1
:
5000) for 2 h at room temperature. After washing with TBST, signals on the membranes were detected by an ECL Plus Western Blotting Detection System (ProteinSimple, San Jose, CA, USA) and analyzed by the Image J gel analysis software.
Lactate dehydrogenase (LDH) detection
PMNs were incubated with β-conglycinin (0.25, 0.5, 1 mg mL−1, 90 min, 37 °C). The supernatants were collected, and the LDH level was detected by the LDH Cytotoxicity Assay kit® (Beyotime Biotechnology, China) by following the operational rules.
Statistical analysis
All the data mentioned above are expressed as mean ± SD. The statistical analysis was performed by the GraphPad Prism 6.0 software. The one-way analysis of variance (ANOVA), followed by the Dunnett's multiple comparison tests was used. Statistical significance was defined by a p value < 0.05.
Results
β-Conglycinin triggered the release of NETs
PMNs treated with β-conglycinin on coverslips were observed under a confocal microscope. There was no NET-like structure in the PMN alone group (Fig. 1A–F), while β-conglycinin induced the formation of NETs (indicated by white arrows), which is confirmed by the co-localization of extracellular DNA and NE or MPO that are the classical characteristics of NETs (Fig. 1G–L).
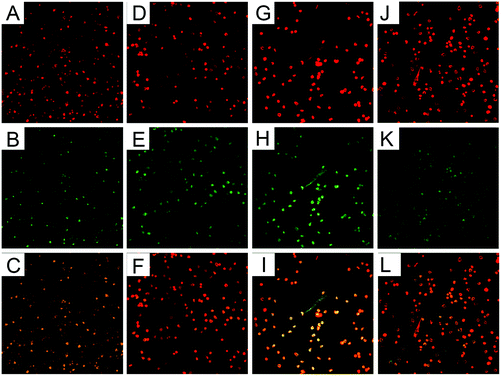 |
| Fig. 1 β-Conglycinin triggered NET release. PMNs were treated with β-conglycinin (1 mg ml−1) for 90 min, and the formation of NETs was determined via Sytox Orange and fluorescence confocal microscopy. (A) DNA (red). (B) NE (green). (C) The merger of (A) and (B) showed DNA decorated with NE in the blank control group NET-network structures (D) DNA (red). (E) MPO (green). (F) Merge of (D) and (E) showed DNA decorated with MPO in the blank control group NET-network structures. (G) DNA (red). (H) NE (green). (I) The merger of (G) and (H) showed DNA decorated with NE in the β-conglycinin-inducted NET-network structures. (J)MPO (red). (K) NE (green). (L) Merge of (J) and (K) showed DNA decorated with MPO in the β-conglycinin-inducted NET-network structures. White arrows indicated NET structures in images J and K. | |
β-Conglycinin induced NET formation in a dose-dependent manner
Pico® Green was used for the quantitation of β-conglycinin-induced NETs. Compared to that of the control group, the NET formation in the β-conglycinin group was markedly increased, and this increase was in a dose-dependent manner (Fig. 2). In addition, DNA degrading enzyme DNase I significantly decreased the formation of NETs induced by β-conglycinin (Fig. 3), suggesting that the DNA concentration derived from the fluorescence intensity with Pico® Green was not affected by β-conglycinin.
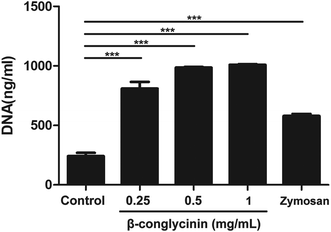 |
| Fig. 2 Quantitation of β-conglycinin-inducted NETs. PMNs were stimulated with β-conglycinin (0.25, 0.5, 1 mg mL−1) for 90 min, and the formation of β-conglycinin-inducted NETs was examined with a fluorometric reader at an excitation wavelength of 485 nm and detecting at 535 nm. Zymosan was used as a positive control. Data are presented as mean ± SD. (***P < 0.001.) | |
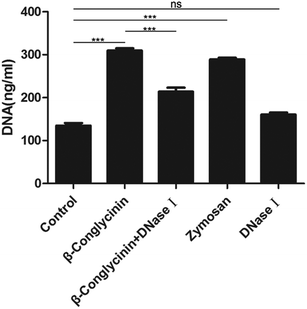 |
| Fig. 3 DNase I reduced β-conglycinin-inducted NETs. PMNs were pretreated with DNase I (1 mg mL−1) for 30 min, the NETs caused by β-conglycinin(1 mg mL−1) were reduced, and the values were similar to those of the blank group. Data are presented as mean ± SD. (***P < 0.001 and “ns” means not significant.) | |
β-Conglycinin-induced NET formation was dependent on NADPH oxidase, ERK1/2, and p38 signaling pathways
Western blotting analysis was used to detect the phosphorylation of ERK1/2 and p38 signaling pathways. As shown in Fig. 4, β-conglycinin markedly increased the phosphorylation of ERK1/2 and p38 in a dose-dependent way. To further study the roles of the NADPH oxidase, ERK1/2, and p38 signaling pathways in the β-conglycinin-induced NET formation, PMNs were pretreated with inhibitors DPI, U0126, and SB202190. Our results showed that the pretreatment of DPI, U0126, and SB202190 significantly inhibited the NET formation induced by β-conglycinin (Fig. 5). These findings suggested that the β-conglycinin-induced NET formation was dependent on the NADPH oxidase, ERK1/2 and p38 signaling pathways.
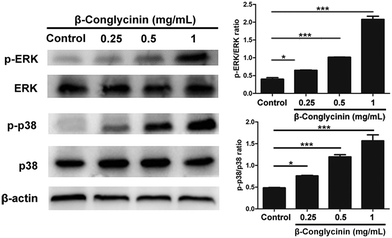 |
| Fig. 4 β-Conglycinin increased the phosphorylation of ERK 1/2 and p38 signaling pathways. PMNs were stimulated with β-conglycinin (0.25, 0.5, 1 mg mL−1) for 90 min, and phosphorylation of ERK and p38 was investigated via the western blotting analysis. The quantification of protein samples was determined via densitometry and normalized. Data are presented as mean ± SD (n = 3). (*P < 0.05 and ***P < 0.001.) | |
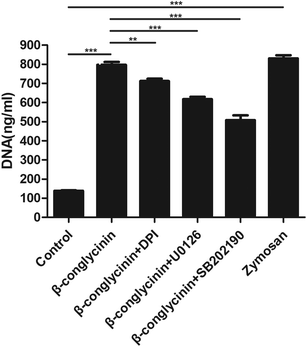 |
| Fig. 5 β-Conglycinin-induced NET formation was dependent on the NADPH oxidase, ERK 1/2, and p38 pathways. PMNs were treated with β-conglycinin (1 mg mL−1) for 90 min in the presence or absence of inhibitors DPI (10 μM), U0126 (50 μM) and SB202190 (10 μM). NET release was quantified with Pico Green. Data are presented as the mean ± SD (*P < 0.05; **P < 0.01; and ***P < 0.001). | |
β-Conglycinin-induced NET formation was a PAD4 and Rac signaling pathway-dependent process
Cl-Amidine and NSC23766, as the specific inhibitor of PAD4 and Rac, respectively, were added to further investigate the underlying mechanism of the β-conglycinin-induced NET formation. Similarly, Cl-amidine and NSC23766 markedly decreased the β-conglycinin-induced NET formation (Fig. 6), indicating the crucial roles of PAD4 and Rac signaling pathways during NET release induced β-conglycinin.
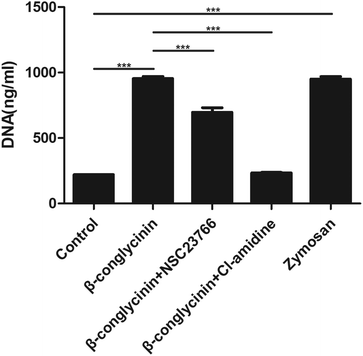 |
| Fig. 6 β-Conglycinin-induced NET formation was a PAD4 and Rac pathway-dependent process. PMNs were treated with β-conglycinin (1 mg mL−1) for 90 min in the presence or absence of inhibitors cl-amidine (6 μM) and NSC23766 (200 μM). NETs release was quantified with Pico Green. Data are presented as the mean ± SD (*P < 0.05; **P < 0.01; and ***P < 0.001). | |
DPI inhibited β-conglycinin-induced the generation of ROS
ROS levels of β-conglycinin-stimulated PMNs were detected by DCFH-DA. As shown in Fig. 7, β-conglycinin markedly increased the level of ROS when compared to the control group. The ROS level induced by β-conglycinin was increased in a dose-dependent way. The formation of NETs was closely related to the production of ROS, which was associated with the activation of the NADPH oxidase.18 As shown in Fig. 8, when PMNs were pretreated with inhibitor DPI for 30 min, the ROS levels increased by β-conglycinin were significantly decreased, suggesting that the NAPDH-dependent ROS participated in the β-conglycinin-induced NET formation.
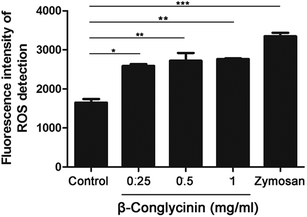 |
| Fig. 7 β-Conglycinin increased the level of ROS. ROS levels in β-conglycinin-inducted PMNs were determined by DCFH-DA and a fluorometric reader. PMNs were treated with β-conglycinin (0.25, 0.5, 1 mg mL−1) for 90 min. Data are presented as mean ± SD (n = 5) (*P < 0.05, **P < 0.01, ***P < 0.001). | |
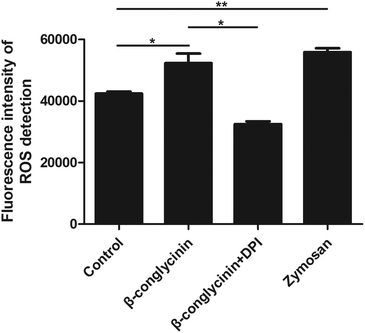 |
| Fig. 8 DPI inhibited β-conglycinin-induced the generation of ROS. PMNs were treated with β-conglycinin (1 mg mL−1) for 90 min in the presence or absence of inhibitors DPI (10 μM). ROS levels were detected by DCFH-DA (10 μM). Data are presented as the mean ± SD (*P < 0.05; **P < 0.01; and ***P < 0.001). | |
β-Conglycinin activated the expression of CD11b in PMNs
CD11b-mediated cell adsorption is essential for cells to exert immune response. Under normal conditions, CD11b has no adsorption.19 When PMNs were stimulated, CD11b had a higher affinity and bond with ligands to mediate PMN adsorption and exudation.20 The activation of CD11b was also detected to further investigate the mechanism of the β-conglycinin-induced NET formation. The western blotting analysis showed that β-conglycinin significantly enhanced the expression of CD11b in a dose-dependent way (Fig. 9).
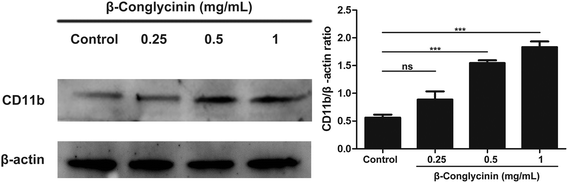 |
| Fig. 9 β-Conglycinin activated the expression of CD11b in PMNs. PMNs were treated with β-conglycinin (0.25, 0.5, 1 mg mL−1) for 90 min. The expression of CD11b was investigated by the western blotting analysis. The quantification of protein samples was determined by densitometry and normalized to β-actin. Data are presented as the mean ± SD (*P < 0.05; **P < 0.01; and ***P < 0.001). | |
β-Conglycinin had no effect on the activity of LDH
The activity of LDH induced by β-conglycinin was detected. The result indicated that β-conglycinin did not influence the activity of LDH in PMNs, while the lysis buffer, which was regarded as the positive control, markedly increased the LDH activity (Fig. 10).
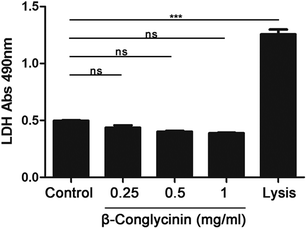 |
| Fig. 10 β-Conglycinin had no effect on the activity of LDH. PMNs were stimulated with β-conglycinin (0.25, 0.5, 1 mg mL−1) for 90 min, LDH activities were detected by the LDH Cytotoxicity Assay kit. Lysis provided by the kits was used as the positive controls. Data are presented as the mean ± SD (***P < 0.001) and “ns” means not significant. | |
Discussion
β-Conglycinin is a heat-stable protein antigen in soybean. Direct heating cannot destroy its antigenic activity, and heated soybean products still cause the abnormal digestion and inflammation of intestinal mucosa in weaning piglets and calves. Thus, considerable attention should be paid to restrict the application of soybean products in the food and feed industry.21 Since β-conglycinin is an antigenic protein, it would activate the body's innate immune system. PMNs, as an indispensable part of the body's non-specific immune cells, are also the primary line of defense against the invasion of harmful pathogens in the body, hence playing an important role in the sensitization process of β-conglycinin.22 However, when diarrhea occurs in young animals, the soy antigen protein contacts the intestinal mucosa and stimulates neutrophils to release NETs. The intestine contains a specific concentration of DNase I, which can hydrolyze the DNA skeleton and form complexes with particulate matter such as MPO. Most of these complexes can be cleared by the phagocytic system in time; however, in some cases, these complexes are deposited into tissues, thus making it difficult to separate from the tissue and thereby releasing active substances such as lysozyme. These active substances, while clearing the complex, also damage surrounding tissues. The catalytic reaction produces excessive oxidants, which damage the intestinal mucosa usually manifesting as diarrhea. There have been many reports on NETs associated with intestinal diseases, but this study is the first exploration of the β-conglycinin-induced NET formation and its underlying mechanism. Our results revealed that β-conglycinin induced the formation of NET-like structures in PMNs, and these structures are extracellular chromatin decorated with histones and antimicrobial proteins, which are in line with the typical characteristics of NETs. In addition, β-conglycinin observably increased the NET formation in a dose-dependent way, which further verified that β-conglycinin indeed is a robust inducer of NETs.
Next, we explored the potential underlying mechanism of β-conglycinin-induced NETs. There are two ways in the NET formation, one is the NADPH oxidase-dependent way, and the other is the NADPH oxidase-independent way.23,24 Some studies showed that NETs are closely related to the ROS production, which is the result of NADPH oxidase activation.25 Our results showed that β-conglycinin significantly increased the ROS generation, but DPI (the inhibitor of the NADPH oxidase) markedly decreased the NET formation, which suggested that the β-conglycinin-induced NET formation was an NADPH-dependent process. It was also reported that Rac could regulate molecular models for the NADPH oxidase, and Rac is an essential component of the NADPH oxidase.26 NSC23766, as an inhibitor of Rac, markedly suppressed the formation of NETs in this study, further confirming that NET formation induced by β-conglycinin was dependent on the NADPH oxidase. PAD4 can catalyze the conversion of arginine at the end of histones to citrulline and participate in the modification of histone H3 and H4 after translation, which promotes chromatin de-densification, facilitates chromatin spine, and regulates chromatin structure and function.27–29 Our results showed that Cl-amidine, the inhibitor of PAD4, markedly decreased the formation of β-conglycinin-induced NETs, indicating that PAD4 was essential for NETs induced by β-conglycinin.
Besides, ROS could activate the MAPK signal pathway and stimulate downstream ERK1/2 and p38 via numerous cascades to promote the NET formation.30 Therefore, we further investigated the effects of β-conglycinin on ERK1/2 and p38 signaling pathways. The western blotting analysis showed that β-conglycinin observably increased the phosphorylation of ERK1/2 and p38. The ERK1/2 inhibitor U0126 and the p38 inhibitor SB202190 significantly inhibited the NET formation challenged by β-conglycinin. Therefore, β-conglycinin-induced NETs were mediated by ERK1/2 and p38 signaling pathways. In the innate immune response, the migration of PMNs to the site of inflammation by endothelial cells is the basis of anti-infection, and the β2 integrin family regulates the adsorption, exudation, and migration of PMNs.31 As the most important membrane protein of the β2 integrin family, CD11b plays a significant role in the functional regulation of PMNs.31,32 In general, CD11b in PMNs is in a state of low expression, but when PMNs are stimulated, the activity of CD11b is rapidly up-regulated and participates in the body's immune response through inducing PMNs and other immune cells to adsorb, exude and migrate.20,33 Our results showed that β-conglycinin activated and increased the expression of CD11b in a dose-dependent way, suggesting that CD11b was involved in the β-conglycinin-induced NET formation. To further illustrate the effect of LDH on β-conglycinin-induced NETs, the supernatant in β-conglycinin-stimulated-PMNs was tested, and it was found that LDH activity did not influence the NET formation.
Conclusions
This study showed that β-conglycinin robustly induced the formation of NETs. Furthermore, we found that the β-conglycinin-induced NET formation was dependent on the NADPH oxidase-derived-ROS, PAD4, ERK1/2 and p38 signaling pathways. All these findings provide new insights into β-conglycinin-related diseases, possibly being helpful to improve these diseases through inhibiting the NET formation. To further explore the detailed effects of NETs on β-conglycinin-induced allergic diseases, extensive relevant in vivo experiments need to be carried out.
List of abbreviations
NETs | Neutrophil extracellular traps |
NADPH oxidase | Nicotinamide adenine dinucleotide phosphate-oxidase |
MPO | Myeloperoxidase |
NE | Neutrophil elastase |
ROS | Reactive oxygen species |
PMN | Polymorphonuclear leukocytes |
PAD4 | Peptidyl arginine deiminase 4 |
MAPK | Mitogen-activated protein kinase |
ERK | Extracellular signal-regulated kinase |
DCF-DA | 2,7-Dichlorodihydrofluorescein diacetate |
DPI | Diphenyleneiodonium chloride |
U0126 | 1,4-Diamino-2,3-dicyano-1,4-bis[2-aminophenylthio] butadiene |
LDH | Lactate dehydrogenase |
BCA | Bicinchoninic acid |
PVDF | Polyvinylidene difluoride |
BSA | Bovine serum albumin |
PBS | Phosphate buffered saline |
ECL | Enhanced chemiluminescence |
ANOVA | One-way analysis of variance |
Conflicts of interest
There is no conflict of interest to declare.
Acknowledgements
This study was supported by grants from the National Natural Science Foundation of China (No. 31772721).
References
- C. Chatterjee, J. Liu and C. Wood,
et al., The alpha’ subunit of beta-conglycinin and various glycinin subunits of soy are not required to modulate hepatic lipid metabolism in rats, Eur. J. Nutr., 2018, 57, 1157–1168 CrossRef CAS.
- C. Peng, C. Cao and M. He,
et al., Soybean Glycinin- and beta-Conglycinin-Induced Intestinal Damage in Piglets via the p38/JNK/NF-kappaB Signaling Pathway, J. Agric. Food Chem., 2018, 66, 9534–9541 CrossRef CAS.
- C. Li and Y. M. Zhang, Molecular evolution of glycinin and beta-conglycinin gene families in soybean (Glycine max L. Merr.), Heredity, 2011, 106, 633–641 CrossRef CAS.
- K. Fujiwara, C. Cabanos and K. Toyota,
et al., Differential expression and elution behavior of basic 7S globulin among cultivars under hot water treatment of soybean seeds, J. Biosci. Bioeng., 2014, 117, 742–748 CrossRef CAS.
- Y. Tsubokura, M. Hajika and H. Kanamori,
et al., The beta-conglycinin deficiency in wild soybean is associated with the tail-to-tail inverted repeat of the alpha-subunit genes, Plant Mol. Biol., 2012, 78, 301–309 CrossRef CAS.
- C. Magni, F. Sessa and J. Capraro,
et al., Structural and functional insights into the basic globulin 7S of soybean seeds by using trypsin as a molecular probe, Biochem. Biophys. Res. Commun., 2018, 496, 89–94 CrossRef CAS.
- S. Gondaira, H. Higuchi and K. Nishi,
et al., Mycoplasma bovis escapes bovine neutrophil extracellular traps, Vet. Microbiol., 2017, 199, 68–73 CrossRef CAS.
- V. Rajendran and A. Uppoor, A perspective on NETosis in diabetes and periodontal diseases, J. Indian Soc. Periodontol., 2018, 22, 290–293 CrossRef.
- L. Badimon and G. Vilahur, Neutrophil extracellular traps: a new source of tissue factor in atherothrombosis, Eur. Heart J., 2015, 36, 1364–1366 CrossRef.
- W. Zhao, D. K. Fogg and M. J. Kaplan, A novel image-based quantitative method for the characterization of NETosis, J. Immunol. Methods, 2015, 423, 104–110 CrossRef CAS.
- B. G. Yipp and P. Kubes, NETosis: how vital is it?, Blood, 2013, 122, 2784–2794 CrossRef CAS.
- V. Brinkmann, U. Reichard and C. Goosmann,
et al., Neutrophil extracellular traps kill bacteria, Science, 2004, 303, 1532–1535 CrossRef CAS.
- P. C. White, I. J. Chicca and M. R. Ling,
et al., Characterization, Quantification, and Visualization of Neutrophil Extracellular Traps, Methods Mol. Biol., 2017, 1537, 481–497 CrossRef CAS.
- A. Carestia, T. Kaufman and M. Schattner, Platelets: New Bricks in the Building of Neutrophil Extracellular Traps, Front. Immunol., 2016, 7, 271 CrossRef.
- K. Martinod and D. D. Wagner, Thrombosis: tangled up in NETs, Blood, 2014, 123, 2768–2776 CrossRef CAS.
- C. Y. Tsai, K. J. Li and S. C. Hsieh,
et al., What’s wrong with neutrophils in lupus?, Clin. Exp. Rheumatol., 2019, 37(4), 684–693 Search PubMed.
- K. H. Lee, A. Kronbichler and D. D. Park,
et al., Neutrophil extracellular traps (NETs) in autoimmune diseases: A comprehensive review, Autoimmun. Rev., 2017, 16, 1160–1173 CrossRef CAS.
- G. T. Nguyen, E. R. Green and J. Mecsas, Neutrophils to the ROScue: Mechanisms of NADPH Oxidase Activation and Bacterial Resistance, Front. Cell. Infect. Microbiol., 2017, 7, 373 CrossRef.
- G. P. Mathias, M. D. Garcia and F. S. Neves, CD11b expression on polymorphonuclear leukocytes from patients with ankylosing spondylitis in a lipopolysaccharide-stimulated whole blood ex vivo model, Int. J. Rheum. Dis., 2017, 20, 60–67 CrossRef CAS.
- K. Zen, Y. L. Guo and L. M. Li,
et al., Cleavage of the CD11b extracellular domain by the leukocyte serprocidins is critical for neutrophil detachment during chemotaxis, Blood, 2011, 117, 4885–4894 CrossRef.
- E. Taliercio, T. M. Loveless and M. J. Turano,
et al., Identification of epitopes of the beta subunit of soybean beta-conglycinin that are antigenic in pigs, dogs, rabbits and fish, J. Sci. Food Agric., 2014, 94, 2289–2294 CrossRef CAS.
- C. Kantari, M. Pederzoli-Ribeil and V. Witko-Sarsat, The role of neutrophils and monocytes in innate immunity, Contrib. Microbiol., 2008, 15, 118–146 CAS.
- M. A. Khan, L. M. Philip and G. Cheung,
et al., Regulating NETosis: Increasing pH Promotes NADPH Oxidase-Dependent NETosis, Front. Med., 2018, 5, 19 CrossRef.
- D. N. Douda, M. A. Khan and H. Grasemann,
et al., SK3 channel and mitochondrial ROS mediate NADPH oxidase-independent NETosis induced by calcium influx, Proc. Natl. Acad. Sci. U. S. A., 2015, 112, 2817–2822 CrossRef CAS.
- C. K. Smith, A. Vivekanandan-Giri and C. Tang,
et al., Neutrophil extracellular trap-derived enzymes oxidize high-density lipoprotein: an additional proatherogenic mechanism in systemic lupus erythematosus, Arthritis Rheumatol., 2014, 66, 2532–2544 CrossRef CAS.
- G. M. Bokoch and B. A. Diebold, Current molecular models for NADPH oxidase regulation by Rac GTPase, Blood, 2002, 100, 2692–2696 CrossRef CAS.
- P. Li, M. Li and M. R. Lindberg,
et al., PAD4 is essential for antibacterial innate immunity mediated by neutrophil extracellular traps, J. Exp. Med., 2010, 207, 1853–1862 CrossRef CAS.
- P. Saha, B. S. Yeoh and X. Xiao,
et al., PAD4-dependent NETs generation is indispensable for intestinal clearance of Citrobacter rodentium, Mucosal Immunol., 2019, 12, 761–771 CrossRef CAS.
- G. Franck, T. L. Mawson and E. J. Folco,
et al., Roles of PAD4 and NETosis in Experimental Atherosclerosis and Arterial Injury: Implications for Superficial Erosion, Circ. Res., 2018, 123, 33–42 CrossRef CAS.
- R. S. Keshari, A. Verma and M. K. Barthwal,
et al., Reactive oxygen species-induced activation of ERK and p38 MAPK mediates PMA-induced NETs release from human neutrophils, J. Cell. Biochem., 2013, 114, 532–540 CrossRef CAS.
- Y. Fang, Y. Chen and S. Ge, [The role of PMN CD11b/CD18 on the increasing PMN adhesion to endothelial cells induced by severe burn injury], Zhonghua Wai Ke Za Zhi, 1997, 35, 504–506 CAS.
- Y. Fang, Y. Chen and S. Ge,
et al., [Effect of CD11b/CD18 on burn-activated PMN-mediated permeability of pulmonary microvascular in isolated perfused lung], Zhonghua Wai Ke Za Zhi, 1998, 36, 690–693 CAS.
- T. Tak, T. P. Rygiel and G. Karnam,
et al., Neutrophil-mediated Suppression of Influenza-induced Pathology Requires CD11b/CD18 (MAC-1), Am. J. Respir. Cell Mol. Biol., 2018, 58, 492–499 CrossRef CAS.
Footnote |
† These authors contributed equally to this work |
|
This journal is © The Royal Society of Chemistry 2021 |
Click here to see how this site uses Cookies. View our privacy policy here.