DOI:
10.1039/D0FO02156C
(Paper)
Food Funct., 2021,
12, 144-153
Isoleucine increases muscle mass through promoting myogenesis and intramyocellular fat deposition†
Received
16th August 2020
, Accepted 14th November 2020
First published on 16th November 2020
Abstract
Isoleucine (Ile), as a branched-chain amino acid (BCAA), has a vital role in regulating body weight and muscle protein synthesis. However, the regulatory effect of Ile on muscle mass under high-fat diet (HFD) conditions and intramyocellular lipid deposition remains largely unclear. In this study, a feeding experiment with HFD with or without 25 g L−1 Ile was performed using 32 wild male C57BL/6J mice randomly divided into two groups. The results showed that Ile significantly increased both muscle and fat mass, as well as causing insulin resistance and meanwhile upregulating the levels of key adipogenic and myogenic proteins. More importantly, Ile damaged the mitochondrial function by vacuolation, swelling and cristae fracture in the gastrocnemius (GAS) and tibialis anterior (TA) with downregulation of mitochondrial function-related genes. Furthermore, Ile promoted myogenesis and more lipid droplet accumulation in myotubes. Compared with the control, the protein levels of myosin heavy chain (MyHC), myoblast determination protein 1 (MyoD), myogenin (MyoG), peroxisome proliferator-activated receptor gamma (PPARg) and fatty acid synthase (FAS) were upregulated in the Ile group, whereas the protein levels of adipose triglyceride lipase (ATGL) and lipoprotein lipase (LPL) were downregulated. Collectively, Ile increased muscle mass through myogenesis and intramyocellular lipid deposition. Our findings provide a new perspective for not only improving the lean juiciness of farm animals by increasing intramyocellular lipid accumulation, but also modulating myopathies under obesity.
1. Introduction
BCAAs are essential for maintaining muscle functions. Food protein contains a large amount of BCAAs which increase muscle mass through promoting protein synthesis.1,2 BCAAs include leucine (Leu), isoleucine (Ile) and valine (Val).3 They are three of the essential amino acids which cannot be synthesized by a living creature itself.4 BCAAs are mainly metabolized in muscles because branched-chain aminotransferase is not expressed in the liver, but is highly expressed in muscles,5 promoting muscle development.6 Leu acted on the mammalian target of rapamycin complex 1 (mTORC1) kinase to initiate translation and protein synthesis.7 Additionally, Leu induced a dramatic increase of biceps femoris muscle weight in fattening pig.8 Val also played a valuable role in muscle protein synthesis enhancement.9 Importantly, Ile was found to be a key regulator in immunity, inflammation and protein metabolism, as well as an activator for promoting muscle protein synthesis.10 Therefore, Ile is implicated in myogenesis and may be a potential indicator of myopathies.
BCAAs are vital emerging factors in obesity. Although BCAAs have a positive effect on muscle protein synthesis, high levels of BCAAs are detected in obese or diabetic patients.11 In recent years, studies on BCAAs have not only been limited to protein synthesis, but also to diseases such as obesity and type 2 diabetes.12 The literature shows that BCAAs modulate lipid metabolism.13 Leu affects lipid deposition in myoblasts.14 Val stimulates fatty acid intake in the skeletal muscle and lead to insulin resistance, which may be due to the accumulation of intramuscular lipids.15 Surplus dietary Ile intake increases intramuscular fat accumulation through depressing the phosphorylation of AMP-activated protein kinase α-acetyl coenzyme A carboxylase, stimulating the expression and activity of stearoyl-CoA desaturase and increasing the capability of lipogenesis in skeletal muscle.16 Although increasing evidence has shown that BCAAs are involved in the occurrence of diseases, especially in obesity, it is particularly meaningful to further explore the relationship between Ile and lipid metabolism.
It is crucial to maintain muscle functions, including the exercise, protection of internal organs and metabolism of glycolipids.17 Adequate muscle mass is essential for health, but overloading in nutrition or exercise increases in muscle mass and cause muscle hypertrophy. Nutrition level is one of the key regulators of muscle mass.18 Skeletal muscle participates in many metabolic processes, such as thermogenic functions, glucose and lipid uptake.19 Literature shows a more lipid accumulation in muscle cells in obese people.20 Previous studies found that mitochondrial reactive oxygen species production increased and mitochondrial dysfunction occurred under obesity induced by HFD.21,22 However, the effect of Ile on intramyocellular lipid deposition has been scarcely studied.
Although BCAAs are powerful factors of protein accretion, muscle mass gain and lipid metabolism, it remains unknown what role Ile plays in the fat deposition of myoblasts and how Ile regulates the muscle mass under HFD conditions and intramyocellular fat deposition. Here, we provided drinking water containing 25 g L−1 Ile to HFD-induced mice to investigate the effect of Ile on lipid deposition and muscle development. Subsequently, we found that Ile not only promoted fat deposition, but also increased muscle mass through promoting myogenesis and lipid droplet accumulation in muscle cells, implying that Ile caused obesity and myopathies under HFD conditions, whereas possibly improved the lean juiciness of farm animals.
2. Materials and methods
2.1 Animals and experimental diets
Thirty-two male C57BL/6J mice were purchased from the Experimental Animal Center of Xi'an Jiao-Tong University, and all animal experiments were approved by the Northwest A&F University Animal Care Committee (approval number NWAFU-314020038). Sixteen mice around 6 weeks old (body weight: 22.90 ± 0.83 g) were equally fed on HFD ad libitum (60% fat, #TP23300, TROPHIC Animal Feed High-tech Co., Ltd, Nantong, China). Another sixteen mice were fed on normal fat diet (10% fat, #TP23302, TROPHIC Animal Feed High-tech Co., Ltd, Nantong, China). Body weight gain was recorded weekly. After 5 weeks, six mice fed on HFD were treated with 25 g L−1 Ile (Sigma-Aldrich, City of Saint Louis, USA) in water for 5 weeks and another six mice drank water without Ile. The mice drank about 15 mL of water every day, so around 0.4 g isoleucine was taken in by the mice daily. Meanwhile, mice fed on a normal-fat diet were also divided into two groups equally and the treatments were consistent with those for the HFD groups. White adipose tissue and skeletal muscle tissue were quickly removed and stored in liquid nitrogen. Serum was used for biochemical tests. The high fat-diet composition is listed in Table S1.†
2.2 Glucose tolerance test (GTT) and insulin tolerance test (ITT)
The mice were fasted overnight for 16 h (GTT) or 4 h (ITT). After recording the weight of each mouse, about 1–2 mm was cut off the end of the tail, blood samples than collected by squeezing, and the fasting baseline blood glucose level determined with a blood glucose meter (YUWELL, Jiangsu, China). Blood glucose levels were detected at 15, 30, 60, 90 and 120 min after the intraperitoneal injection of glucose (1.5 g kg−1) or insulin (0.65 U kg−1).
2.3 Blood biochemical index test
Blood was collected at the end of the experiment and supernatant obtained by centrifugation. The biochemical index was tested in the Yangling Demonstration Zone Hospital (Yangling, Shaanxi, China).
2.4 Detection of triglyceride (TG) content
A triglyceride detection kit (Solabio, Beijing, China) was used to detect the content of TG in muscle tissue. In short, TG is extracted with isopropanol. Potassium hydroxide is saponified and TG is hydrolyzed to produce glycerol and fatty acids, and periodic acid oxidizes the glycerol to formaldehyde. Then, formaldehyde and acetylacetone are condensed into yellow substances in the presence of hydrogen ions. There is a characteristic absorption peak at 120 nm, and the color grayscale is proportional to the TG content. The absorption was determined with a Microplate Reader spectrophotometer (51119080, ThermoFisher, Waltham, USA).
2.5 Transmission electron microscope experiment
Tissue samples were put into the fixing solution. Chemicals were used to fix the cell structure, and then copper mesh was double-stained with uranium and lead. Ultra-thin sections were made and observed under a transmission electron microscope (TECNAI G2 SPIRIT BIO, FEI, Hillsboro, USA).
2.6 Cell culture
The C2C12 cells were obtained from the American Type Culture Collection (ATCC) and cultured in high glucose Dulbecco's modified Eagle's medium (DMEM; Gibco, Waltham, USA), which contained a 0.8 mM Ile basal concentration with 10% fetal bovine serum (Gibco, Waltham, USA) and 1% penicillin and streptomycin at 37 °C and 5% CO2. Cells were induced to differentiation using DMEM with 2% horse serum (Gibco, Waltham,USA). On day 7, cells were incubated for 24 h with DMEM containing 0.5% fatty acid-free bovine serum albumin (BSA), 500 μM oleate (Aladdin, Shanghai, China) and 1 mM Ile, and then harvested.
2.7 EdU staining
Diluted 5-ethynyl-2′-deoxyuridine (EdU) solution (reagent A) with the cell culture medium at a ratio of 1
:
1000 was added (100 μL) to each well of a 96-well plate, and incubated for 2 h. After washing the cells with phosphate buffered saline (PBS), 4% paraformaldehyde was added and fixed for 30 min, followed by washing with PBS, then adding 2 mg mL−1 glycine and incubating for 5 min. Finally, a DNA staining was performed.
2.8 Cell counting assay
A Cell Counting Kit-8 (CCK-8, Vazyme Biotech, Nanjing, China) was used to test cell viability. Cells were seeded in a 96-well plate at a density of 2 × 103 cells per well and cultured in 100 μL of DMEM with 1 mM Ile for 24 h. Then, 10 μL of the CCK-8 kit reagent and 90 μL of DMEM were added to each well and the cells incubated at 37 °C for 2 h. The measurement was done using a VICTOR5 Multilabel Plate Reader (PerkinElmer, Waltham, Massachusetts, USA) at 450 nm, according to the manufacturer's instructions.
2.9 Flow cytometry analysis
The cells were cultivated in a 60 mm dish and left for the cell density to reach 30%–40%. Ile treatment was for 24 h. The cells were digested using trypsin and washed three times with PBS, then collected into a 1.5 mL centrifuge tube. The cell pellet was washed with 1 mL 70% pre-cool ethanol and fixed at 4 °C overnight. Finally, a flow cytometry instrument (Becton Dickinson, Franklin Lakes, NJ, USA) was used to analyze the proliferation phase of the cells.
2.10 Oil red O staining
After treatment, the cells were washed with PBS and fixed with 4% paraformaldehyde for 30 min. After washing with PBS three times, the cells were stained with Oil Red O solution (Solabio, Beijing, China) for 20 min and then washed with PBS three times. The cells were visualized by phase-contrast microscopy (Tokyo, Japan). Oil Red O dissolved in lipid droplets was extracted with 100% isopropanol and its relative concentrations were determined by measuring the absorbance at 510 nm.
2.11 Hematoxylin and eosin (H&E) staining
Muscle tissue was fixed with 4% paraformaldehyde for a paraffin section and frozen slice. After dewaxing, the paraffin slices were stained with hematoxylin and eosin, and then observed under a microscope.
2.12 Immunofluorescence staining
The differentiated C2C12 were fixed with 4% paraformaldehyde for 20 min, then immersed in 0.5% Triton X-100, permeabilized for 20 min at room temperature and blocked with 0.5% BSA for 30 min. The mouse anti-MyHC (1
:
1000, MAB4470, R&D Systems, hournesota, USA) was incubated overnight at 4 °C and IgG-FITC (1
:
100, sc-2024, Santa Cruz Biotechnology, Dallas, Texas, USA) for 1 h at 25 °C in the dark. Nuclei were stained for 5 min in the dark using 4′,6-dimidyl-2-phenylindole (DAPI). Then, the cells were photographed on a fluorescence microscope with 100 μl PBS in each well.
2.13 BODIPY staining
Frozen skeletal tissue was fixed with 4% paraformaldehyde for 20 min and then immersed in Boron-dipyrromethene (BODIPY) solution for 30 min at 37 °C. After the samples were washed 3 times with PBS, the stained droplets were observed using a fluorescence microscope.
2.14 AdipoRed staining
The differentiated C2C12s were washed with PBS three times. AdipoRed liquid was added into plate for 20 min. Nuclei were stained for 5 min in the dark using DAPI. Then, 100 μL PBS was added to each well and photographed on a fluorescence microscope.
2.15 RNA isolation and real-time quantitative polymerase chain reaction (RT-qPCR)
Total RNA was isolated from the cells and tissues using Trizol reagent (Invitrogen, Waltham, USA) and was reversely transcribed using reverse transcription kits (Takara, Kyoto, Japan) according to the manufacturer's instruction. RT-qPCR was performed with an SYBR green kit (Vazyme, Nanjing, China) with a Bio-Rad iQ5 system (Bio-Rad, Hercules, CA, USA). The primer sequences are listed in Table S2.†
2.16 Western blotting
Total proteins of cells and tissues were extracted using RIPA extraction reagents (Applygen, Beijing, China) with added protease inhibitor cocktail (1/4 volume of RIPA). A total of 20 μg of cell or tissue protein was separated by 10% SDS-PAGE and subsequently transferred onto polyvinylidene fluoride (PVDF) membranes (Millipore, Billerica, Massachusetts, USA). The membranes were blocked with 5% skimmed milk powder/1× tris-buffered saline Tween-20 (TBST), primary antibodies diluted in 5% BSA/TBST, washed with TBST, and then incubated with HRR-conjugated secondary antibodies for 1.5 h at room temperature before visualization with chemiluminescent substrate (Millipore) and quantification was performed using Image Lab System (Bio-Rad, Hercules, California, USA). The primary antibodies used in these experiments were as follows: mouse anti-MyoD (1
:
1000, NB100-56511, Novus Biologicals, Minneapolis, Minnesota USA), mouse anti-MyoG (1
:
500, NB100-56510, Novus Biologicals, Minneapolis, Minnesota USA), mouse anti-MyHC (1
:
1000, MAB4470, R&D Systems, Minnesota, USA), rabbit anti-PPARg (1
:
100, sc-7196; Santa Cruz Biotechnology, Dallas, Texas, USA), mouse anti-FAS (1
:
100, sc-55580, Santa Cruz Biotechnology, Dallas, Texas, USA), mouse anti-aP2 (1
:
100, sc-271529; Santa Cruz Biotechnology, Dallas, Texas, USA), rabbit anti-ATGL (1
:
1000, CS-2138, Cell Signaling Technology, Massachusetts, USA), goat anti-LPL (1
:
100, sc-32382, Santa Cruz Biotechnology, Dallas, Texas, USA), rabbit anti-Cyclin D (1
:
500, BA0770, BOSTER, Wuhan, China), mouse anti-Cyclin E (1
:
100, sc-377100, Santa Cruz Biotechnology, Dallas, Texas, USA), mouse anti-p27 (1
:
200, sc-56338, Santa Cruz Biotechnology, Dallas, Texas, USA), mouse anti-β-tubulin antibody (1
:
20
000, BC000748; Proteintech, Wuhan, China), and mouse anti-β-actin antibody (1
:
2000, CW0096; CWBio, Beijing, China). Secondary antibodies included goat anti-rabbit (1
:
3000, BA1054; BOSTER, Wuhan, China), rabbit anti-goat (1
:
3000, BA1054; BOSTER, Wuhan, China), and goat anti-mouse (1
:
3000, BA1050; BOSTER, Wuhan, China).
2.17 Statistical analysis
Statistical analyses were performed in GraphPad Prism version 8 statistical software (La Jolla) using the one-way analysis of variance and multiple t test. Data are expressed as the mean ± SEM. P < 0.05 was considered as significant. * P < 0.05, ** P < 0.01.
3. Result
3.1 Ile promotes fat deposition and aggravates insulin resistance in vivo
Compared with the control, the body weight apparently increased in the Ile treatment group (Fig. 1A and B), and the inguinal white adipose tissue (iWAT) and epididymal white adipose tissue (eWAT) mass was also markedly augmented (Fig. 1C and D). In addition, the results of the serum physiological indexes showed that the glucose and low density lipoprotein (LDL) in the serum were significantly increased in the Ile treatment group, but there was a little difference on the total cholesterol (T-chol) and triglyceride content (Fig. 1E–H). Moreover, the results of the glucose tolerance test (GTT) and insulin tolerance test (ITT) indicated that Ile decreased the glucose tolerance and caused insulin resistance (Fig. 1I–L). Meanwhile, Ile supplemented in the chow diet did not promote fat deposition and aggravated insulin resistance in vivo (Fig. S1†). Taken together, Ile promoted fat deposition and aggravated insulin resistance.
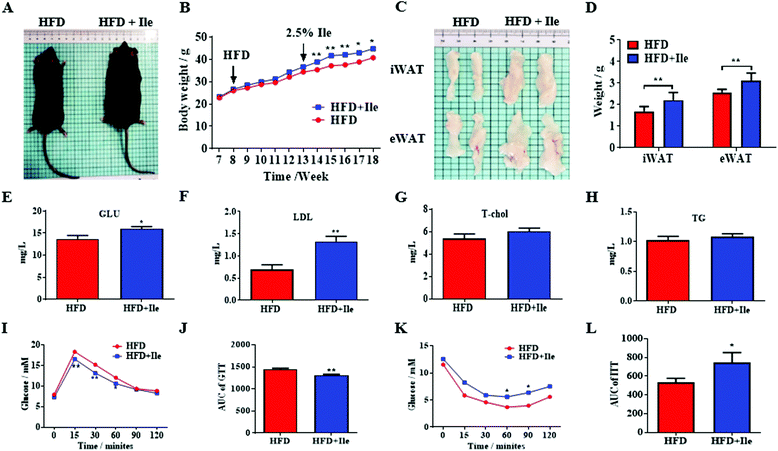 |
| Fig. 1 Ile aggravated fat deposition and caused insulin resistance. (A) Mice treated with high-fat diet (HFD) and HFD plus Ile. (B) Body weight curves. (C) Inguinal white adipose tissue (iWAT) and epididymal white adipose tissue (eWAT). (D) The weights of iWAT and eWAT. (E) Glucose (Glu) levels in serum. (F) Low-density lipoprotein (LDL) levels. (G) Total cholesterol (T-chol) levels. (H) Triglyceride (TG) levels. (I) Glucose tolerance test (GTT). (J) Area under the curve (AUC) of GTT. (K) Insulin sensitivity test (ITT). (L) AUC of ITT. The data are shown as mean ± SEM. * P < 0.05, ** P < 0.01. | |
3.2 Ile dramatically increases muscle mass and induces intramyocellular lipid deposition in vivo
As shown in Fig. 2A and B, Ile supplementation significantly enlarged GAS, TA and EDL, and remarkably increased their masses, resulting in skeletal muscle hypertrophy in Ile treatment group. However, there is no significant change between chow diet and chow diet supplement with Ile (Fig. S2†). Interestingly, the contents of TG in GAS, TA and EDL also ascended in Ile treatment group (Fig. 2C). Additionally, the results of H&E staining showed that Ile increased the muscle fiber density of GAS and TA (Fig. 2D). Furthermore, compared with the control, Ile promoted lipid accumulation of GAS and TA using BODIPY staining (Fig. 2D). The statistical results of muscle fiber size showed that Ile reduced the fiber diameter extremely significantly (Fig. 2E). Consistently, Ile upregulated the protein levels of both myogenic and adipogenic genes, including MyoG, MyHC, MyoD, PPARg and adapter protein (aP2) in GAS and TA (Fig. 2F–H), whereas downregulated the protein levels of the key lipolytic gene ATGL (Fig. 2I–K). Based on the results, Ile significantly increased muscle mass and induced intramyocellular lipid deposition in vivo. Since the liver is an important organ for fat metabolism, we also tested the level of lipid metabolism in the liver after Ile treatment. The results showed that Ile also promoted hepatic lipogenesis (Fig. S3†).
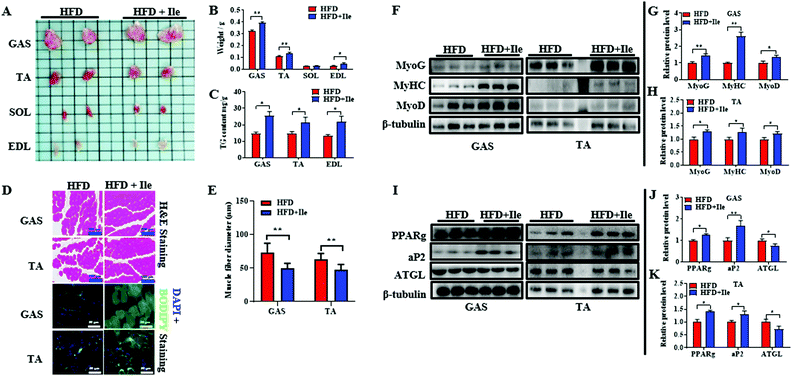 |
| Fig. 2 Ile increased muscle mass and intramyocellular lipid deposition. (A) Skeletal muscles treated with HFD and HFD plus Ile. Gastrocnemius (GAS), tibialis anterior (TA), soleus (SOL), extensor digitorum longus (EDL). (B) The tissue weights of GAS, TA, SOL and EDL. (C) TG contents of TA, GAS and EDL. (D) H&E staining (scale bars 200 μm) and BODIPY staining (scale bars 50 μm) of GAS and TA. (E) The statistics of fiber diameters of GAS and TA. (F) Western blot images of myogenic genes in GAS and TA. β-Tubulin was used as a control. (G and H) The relative protein levels of myogenic genes in GAS (G) and TA (H). (I) Western blot images of lipogenic and lipolytic genes. (J and K) The relative protein levels of lipogenic and lipolytic genes in GAS (J) and TA (K). The data are shown as mean ± SEM. * P < 0.05, ** P < 0.01. | |
3.3 Ile results in mitochondrial dysfunction of muscle fiber in vivo
To further explore fat deposition in skeletal muscles, the morphology of mitochondria in muscles was observed using a transmission electron microscope. Expectedly, compared with the control, Ile significantly changed the mitochondrial morphology, with a vacuole occurring and ridges broken or even disappeared in the GAS and TA (Fig. 3A). Moreover, the mRNA levels of cytochrome C oxidase 2 (COX2), citrate synthase (CS), peroxisome proliferator-activated receptor gamma coactivator 1-alpha (PGC-1α) and mitochondrial transcription factor A (TFAM) decreased in GAS and TA (Fig. 3B and C). Therefore, the mitochondrial dysfunction caused by Ile may be implicated intramyocellular lipid deposition.
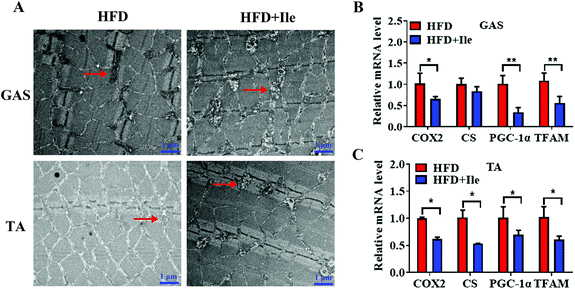 |
| Fig. 3 Ile resulted in mitochondrial dysfunction in GAS and TA. (A) Transmission electron microscope images of GAS and TA. Scale bars 1 μm. The arrows indicate the mitochondria. (B and C) The mRNA levels of cytochrome C oxidase 2 (COX2), citrate synthase (CS), peroxisome proliferator-activated receptor gamma coactivator 1-alpha (PGC-1α) and mitochondrial transcription factor A (TFAM) in GAS (B) and TA (C). The data are shown as mean ± SEM. * P < 0.05, ** P < 0.01. | |
3.4 Ile promotes proliferation and myogenic differentiation of myoblasts
To reveal the underlying regulatory role of Ile increasing the muscle mass, we assessed the effect of Ile on proliferation and myogenic differentiation in myoblasts. Compared with the basal concentrate (0.8 mM), Ile supplementation increased the newly proliferating cells (Fig. 4A and B), and the result of CCK-8 assay was consistent (Fig. 4C). Flow cytometry assay showed that the number of S-phase cells also increased (Fig. 4D and E). The levels of cell cycle genes (CyclinE and CyclinD) were upregulated, and meanwhile p27 cells were downregulated (Fig. 4F and G). These results indicated that Ile promoted myoblast proliferation. Moreover, Ile apparently facilitated myoblast fusion to form more myotubes (Fig. 4H), and meanwhile increased the protein levels of key myogenic genes, including MyHC, MyoD and MyoG (Fig. 4I and J), showing that Ile promoted myogenic differentiation in myoblasts. Taken together, Ile promotes proliferation and myogenic differentiation in myoblasts.
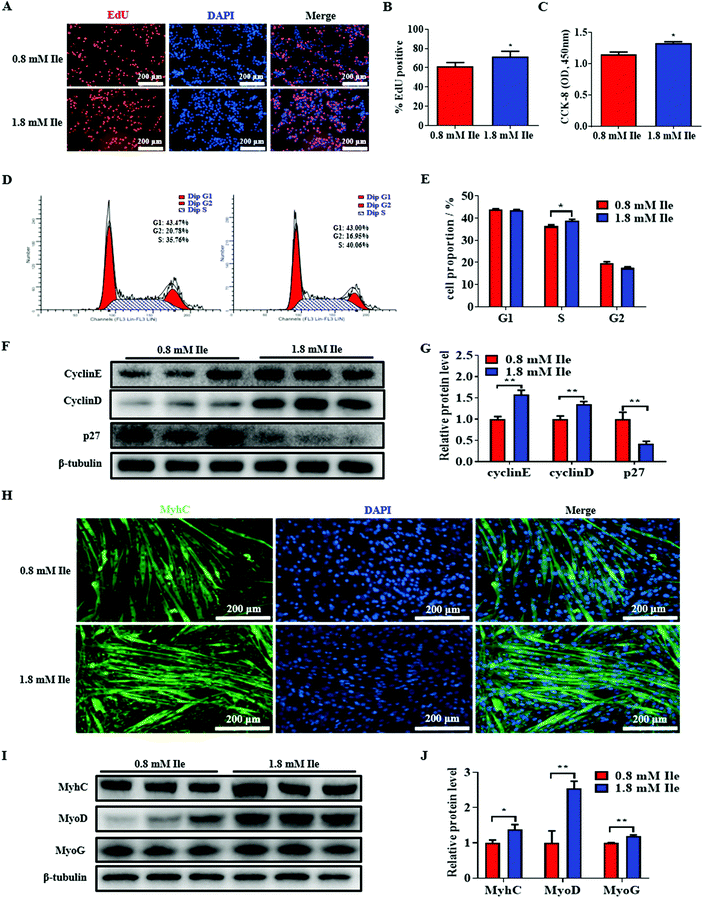 |
| Fig. 4 Ile promoted proliferation and myogenic differentiation in myoblasts. (A) EdU staining of C2C12 treated with Ile after proliferation at 24 h. (B) Percentage of EdU-positive cells. Scale bar 200 μm. (C) CCK-8 assay. (D) Flow cytometry analysis. (E) Percentage of different proliferation phages. Gap phage 1 (G1), DNA synthesis phage (S) and gap phage 2 (G2). (F) Western blot images of cell cycle genes. (G) The relative protein levels. (H) Immunofluorescence analysis of myotubes. Myotubes were stained using the heavy chain of myosin II (green) and cell nucleus were stained by DAPI (blue), respectively. Scale bars 200 μm. (I) Western blot images of myogenic genes. (J) The relative protein levels. The data are shown as mean ± SEM; * P < 0.05, ** P < 0.01. | |
3.5 Ile apparently accumulates intramyocellular lipid deposition in myotubes
To further investigate intramyocellular lipid deposition in vitro, myoblasts were treated with 500 μM oleate23 plus 1 mM Ile to induce differentiation. The results indicated that Ile significantly promoted fat formation in myotubes, from AdipoRed and Oil Red O staining analysis (Fig. 5A–C). Moreover, the protein levels of the key lipogenic and lipolytic genes were detected in the myotubes. As shown in the 1.8 mM Ile group, the protein expression levels of PPARg and FAS were significantly upregulated, while ATGL and LPL were markedly downregulated (Fig. 5D and E). Therefore, Ile apparently accumulates intramyocellular lipid deposition. In summary, our results suggested that Ile increased muscle mass through promoting myogenesis and intramyocellular fat deposition (Fig. 6).
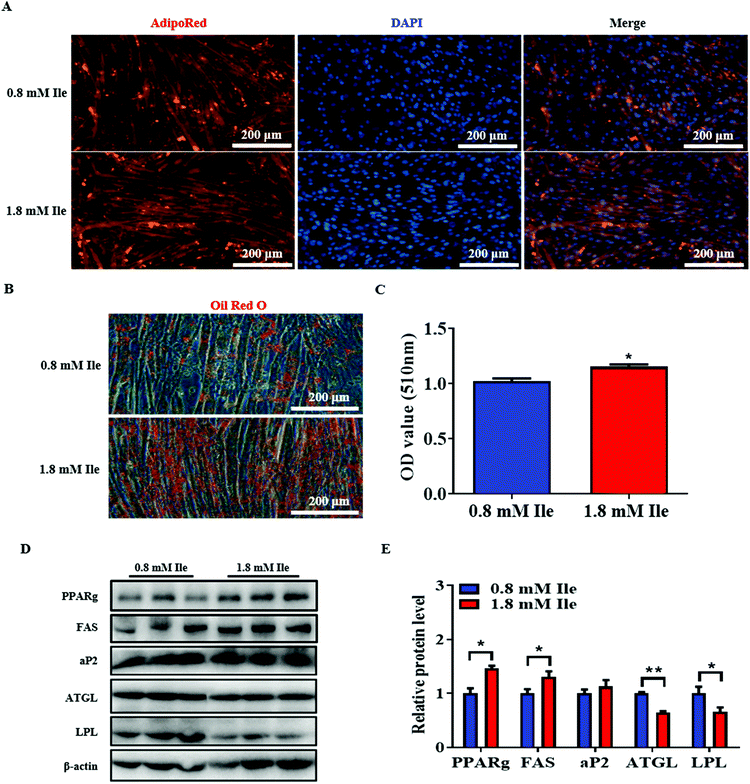 |
| Fig. 5 Ile significantly promoted intramyocellular lipid accumulation in myotubes. Myoblasts induced differentiation on day 6, and were then treated with 500 μM oleate and 1 mM Ile for 24 h. (A) AdipoRed staining of myotubes. (B) Oil Red O staining. (C) Relative absorbance of Oil Red O. (D) Western blot images of lipogenic and lipolytic genes. β-actin was used as a control. (E) The relative protein levels. Scale bars 200 μm. The data are shown as mean ± SEM; * P < 0.05, ** P < 0.01. | |
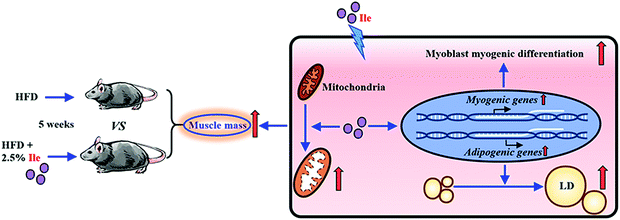 |
| Fig. 6 Schematic diagram for Ile increasing muscle mass through promoting myogenesis and intramyocellular fat deposition. | |
4. Discussion
Ile is a key nutrition factor for humans and animals because it is an important essential amino acid and can only be obtained from food.4 Meanwhile, studies revealed that Ile participated in protein synthesis and lipid metabolism,10,16 implying it has a potential function in myogenesis and fat formation. To date, it remains largely unclear as to the effect and mechanism of Ile regulating muscle development and lipid deposition under HFD conditions. In this study, Ile not only markedly promoted iWAT and eWAT deposition under HFD conditions, but also increased the GAS, TA and EDL mass with the upregulation of key myogenic genes. The lipogenesis in liver is also elevated in the Ile supplemented group. The process of skeletal muscle fiber hypertrophy is due to the active muscle protein synthesis and muscle cell differentiated to muscle fibers.24 Furthermore, we assessed the effect of Ile on the proliferation and myogenic differentiation in myoblasts. As expected, Ile promoted both the proliferation and myogenic differentiation of myoblasts, as well as increased the protein levels of important myogenic genes. Simultaneously, studies indicated that increased concentration of BCAAs led to insulin resistance under obesity,12,25 and more insulin promoted protein synthesis.26 Here, we found that Ile aggravates insulin resistance and increased the protein levels of myogenic genes under HFD conditions. Meanwhile, the changes were not significant between the chow diet group and the chow diet supplemented with the Ile control. Based on our findings, Ile promoted skeletal muscle development mainly through myogenesis and was implicated in both obesity and lipodystrophic muscular hypertrophy.
Another important reason was intramyocellular lipid accumulation in augmenting muscle mass. Increasing studies showed that BCAAs also regulated fat formation.27,28 Lack of Ile or Val leads to a reduction in white fat mass.29 Conversely, addition of excess Ile to the diet significantly promoted the production of intramuscular fat.16 It is possible that Ile promotes lipogenesis and inhibits lipolysis, resulting in fat deposition. Interestingly, we here found that Ile promoted lipid accumulation in skeletal muscle fiber of GAS and TA, and meanwhile the protein levels of the key adipogenic genes and TG content of muscles both increased. Moreover, our results further indicated that Ile resulted in more lipid accumulation in myotubes with upregulating of the protein levels of FAS and PPARg, hinting that Ile promoted intramyocellular fat deposition, which was another important reason causing the increase of skeletal muscle. Generally, when lipogenesis increases, lipolysis inevitably decreases. In this study, Ile significantly downregulated the protein levels of vital lipolytic genes including ATGL and LPL, inhibiting lipolysis in myotubes. In addition, mitochondria are important organelles which participate in the lipid synthesis and oxidative metabolism.30 In most cases, mitochondrial dysfunction is associated with metabolic abnormalities in muscle and adipose tissues.31 Besides, triglyceride synthesis increases when fatty acids cannot enter the mitochondria for β-oxidation.32 Consistently, we found that Ile made mitochondria vacuoles occur and the ridge to break or vanish to cause mitochondrial dysfunction in skeletal muscle tissue, implying that Ile promoted intramyocellular fat deposition through mitochondrial dysfunction.
The positive effect of Ile on myogenesis and intramyocellular lipid formation provided a guide to control obesity and myopathies. Recent studies identified that the level of circulating BCAAs in the body increased under pathological conditions, including obesity, type 2 diabetes and cardiovascular diseases.11 In this study, Ile not only increased fat mass, but also aggrandized skeletal muscle mass under HFD conditions, resulting in a greater body weight. However, we need to point out that there was an inconsistent result about the effect of Ile on body weight.33 The differences may be because the authors used: 10 weeks-old male mice, HFD containing 45% fat for 6 weeks, and then a 28-d Ile plus methylcellulose treatment period, whereas in our study we used: 6 weeks-old male mice, 60% fat content HFD for 10 weeks, then treatment with only Ile for 5 weeks. In addition, both obesity and the fat deposition in muscles caused insulin resistance.3,15 Here, we found that Ile resulted in lipid formation in both WAT and skeletal muscle combined with insulin resistance, indicating that Ile deteriorated obesity and myopathies under HFD conditions, but improved the lean juiciness of farm animals by promoting intramyocellular lipid deposition. Therefore, we thought that the effect of Ile was two sides of a coin in modulating human obesity and myopathies, as well as promoting the meat quality of farm animals.
5. Conclusion
Ile, as an important essential amino acid, affects multiple biological processes. In this study, the results showed that Ile significantly increased both muscle and fat mass under HFD conditions. Further research identified that Ile increased muscle mass through promoting myogenic differentiation and intramyocellular lipid accumulation. Therefore, the findings suggest that surplus dietary Ile intake is a potential method not only for improving meat yield and quality of farm animals, but also for modulating obesity and myopathies.
Conflicts of interest
Authors declare that there is no conflict of interests.
Acknowledgements
This research was granted by following funding, the National Natural Science Foundation (31872979, 31572366), the National Key Research and Development Program of China (2017YFD0502002) and the Key Research and Development Program of Shaanxi Province (2018ZDXL-NY-02-03).
References
- P. J. White and C. B. Newgard, Branched-chain amino acids in disease, Science, 2019, 363, 582–583 CrossRef CAS.
- E. Blomstrand and B. Saltin, BCAA intake affects protein metabolism in muscle after but not during exercise in humans, Am. J. Physiol. Endocrinol. Metab., 2001, 281, E365–E374 CrossRef CAS.
- M. Zhou, J. Shao, C. Y. Wu, L. Shu, W. Dong, Y. Liu, M. Chen, R. M. Wynn, J. Wang, J. Wang, W. J. Gui, X. Qi, A. J. Lusis, Z. Li, W. Wang, G. Ning, X. Yang, D. T. Chuang, Y. Wang and H. Sun, Targeting BCAA Catabolism to Treat Obesity-Associated Insulin Resistance, Diabetes, 2019, 68, 1730–1746 CrossRef CAS.
- G. Wu, Amino acids: metabolism, functions, and nutrition, Amino Acids, 2009, 37, 1–17 CrossRef CAS.
- P. Wang, S. Wu, X. Zeng, Y. Zhang, Y. Zhou, L. Su and W. Lin, BCAT1 promotes proliferation of endometrial cancer cells through reprogrammed BCAA metabolism, Int. J. Clin. Exp. Pathol., 2018, 11, 5536–5546 CAS.
- Y. Haba, T. Fujimura, K. Oyama, J. Kinoshita, T. Miyashita, S. Fushida, S. Harada and T. Ohta, Effect of Oral Branched-Chain Amino Acids and Glutamine Supplementation on Skeletal Muscle Atrophy After Total Gastrectomy in Rat Model, J. Surg. Res., 2019, 243, 281–288 CrossRef CAS.
- Y. Kamei, Y. Hatazawa, R. Uchitomi, R. Yoshimura and S. Miura, Regulation of Skeletal Muscle Function by Amino Acids, Nutrients, 2020, 12 Search PubMed.
- C. Hu, F. Li, Y. Duan, X. Kong, Y. Yan, J. Deng, C. Tan, G. Wu and Y. Yin, Leucine alone or in combination with glutamic acid, but not with arginine, increases biceps femoris muscle and alters muscle AA transport and concentrations in fattening pigs, J. Anim. Physiol. Anim. Nutr., 2019, 103, 791–800 CrossRef CAS.
- C. S. Santos and F. E. L. Nascimento, Isolated branched-chain amino acid intake and muscle protein synthesis in humans: a biochemical review, Einstein, 2019, 17, eRB4898 CrossRef.
- S. W. Olde Damink, R. Jalan, N. E. Deutz, C. H. Dejong, D. N. Redhead, P. Hynd, P. C. Hayes and P. B. Soeters, Isoleucine infusion during “simulated” upper gastrointestinal bleeding improves liver and muscle protein synthesis in cirrhotic patients, Hepatology, 2007, 45, 560–568 CrossRef CAS.
- O. Zhenyukh, E. Civantos, M. Ruiz-Ortega, M. S. Sánchez, C. Vázquez, C. Peiró, J. Egido and S. Mas, High concentration of branched-chain amino acids promotes oxidative stress, inflammation and migration of human peripheral blood mononuclear cells via mTORC1 activation, Free Radicals Biol. Med., 2017, 104, 165–177 CrossRef CAS.
- M. A. B. Siddik and A. C. Shin, Recent Progress on Branched-Chain Amino Acids in Obesity, Diabetes, and Beyond, Endocrinol. Metab., 2019, 34, 234–246 CrossRef.
- D. K. Layman, The role of leucine in weight loss diets and glucose homeostasis, J. Nutr., 2003, 133, 261s–267s CrossRef.
- T. Moro, S. M. Ebert, C. M. Adams and B. B. Rasmussen, Amino Acid Sensing in Skeletal Muscle, Trends Endocrinol. Metab., 2016, 27, 796–806 CrossRef CAS.
- C. J. Lynch and S. H. Adams, Branched-chain amino acids in metabolic signalling and insulin resistance, Nat. Rev. Endocrinol., 2014, 10, 723–736 CrossRef CAS.
- Y. Luo, X. Zhang, Z. Zhu, N. Jiao, K. Qiu and J. Yin, Surplus dietary isoleucine intake enhanced monounsaturated fatty acid synthesis and fat accumulation in skeletal muscle of finishing pigs, J. Anim. Sci. Biotechnol., 2018, 9, 88 CrossRef CAS.
- R. Kjøbsted, J. R. Hingst, J. Fentz, M. Foretz, M. N. Sanz, C. Pehmøller, M. Shum, A. Marette, R. Mounier, J. T. Treebak, J. F. P. Wojtaszewski, B. Viollet and L. Lantier, AMPK in skeletal muscle function and metabolism, FASEB J., 2018, 32, 1741–1777 CrossRef.
- M. J. Rennie, H. Wackerhage, E. E. Spangenburg and F. W. Booth, Control of the size of the human muscle mass, Annu. Rev. Physiol., 2004, 66, 799–828 CrossRef CAS.
- L. Sylow, M. Kleinert, E. A. Richter and T. E. Jensen, Exercise-stimulated glucose uptake - regulation and implications for glycaemic control, Nat. Rev. Endocrinol., 2017, 13, 133–148 CrossRef CAS.
- C. Moro, J. E. Galgani, L. Luu, M. Pasarica, A. Mairal, S. Bajpeyi, G. Schmitz, D. Langin, G. Liebisch and S. R. Smith, Influence of gender, obesity, and muscle lipase activity on intramyocellular lipids in sedentary individuals, J. Clin. Endocrinol. Metab., 2009, 94, 3440–3447 CrossRef CAS.
- J. Hong, Y. Jia, S. Pan, L. Jia, H. Li, Z. Han, D. Cai and R. Zhao, Butyrate alleviates high fat diet-induced obesity through activation of adiponectin-mediated pathway and stimulation of mitochondrial function in the skeletal muscle of mice, Oncotarget, 2016, 7, 56071–56082 CrossRef.
- M. D. Kristensen, S. M. Petersen, K. E. Møller, M. T. Lund, M. Hansen, C. N. Hansen, J. Courraud, J. W. Helge, F. Dela and C. Prats, Obesity leads to impairments in the morphology and organization of human skeletal muscle lipid droplets and mitochondrial networks, which are resolved with gastric bypass surgery-induced improvements in insulin sensitivity, Acta Physiol., 2018, 224, e13100 CrossRef CAS.
- A. Q. Xiang, G. Y. Chu, Y. B. Zhu, G. Ma, G. S. Yang and S. D. Sun, IGFBP5 suppresses oleate-induced intramyocellular lipids deposition and enhances insulin signaling, J. Cell Physiol., 2019 DOI:10.1002/jcp.28174.
- S. M. Phillips, A brief review of critical processes in exercise-induced muscular hypertrophy, Sports Med., 2014, 44, S71–S77 CrossRef.
- J. Tian, C. Liu, X. Zheng, X. Jia, X. Peng, R. Yang, X. Zhou and X. Xu, Porphyromonas gingivalis Induces Insulin Resistance by Increasing BCAA Levels in Mice, J. Dent. Res., 2020, 99, 839–846 CrossRef CAS.
- S. M. Phillips, Insulin and muscle protein turnover in humans: stimulatory, permissive, inhibitory, or all of the above?, Am. J. Physiol. Endocrinol. Metab., 2008, 295, E731 CrossRef CAS.
- C. R. Green, M. Wallace, A. S. Divakaruni, S. A. Phillips, A. N. Murphy, T. P. Ciaraldi and C. M. Metallo, Branched-chain amino acid catabolism fuels adipocyte differentiation and lipogenesis, Nat. Chem. Biol., 2016, 12, 15–21 CrossRef CAS.
- M. Zhou, J. Shao, C. Wu, L. Shu, W. Dong, Y. Liu, M. Chen, R. Wynn, J. Wang, J. Wang, W. Gui, X. Qi, J. L. Aldons, Z. Li, W. Wang, G. Ning, X. Yang, T. C. David, Y. Wang and H. Sun, Targeting BCAA Catabolism to Treat Obesity-Associated Insulin Resistance, Diabetes, 2019, 68, 1730–1746 CrossRef CAS.
- Y. Du, Q. Meng, Q. Zhang and F. Guo, Isoleucine or valine deprivation stimulates fat loss via increasing energy expenditure and regulating lipid metabolism in WAT, Amino Acids, 2012, 43, 725–734 CrossRef CAS.
- S. Melanie, T. Takashi and L. Thomas, Mitochondrial lipid transport
at a glance, J. Cell Sci., 2013, 126, 5317–5323 CrossRef.
- W. Sutham, J. Sripetchwandee, W. Minta, D. Mantor, S. Pattanakuhar, S. Palee, W. Pratchayasakul, N. Chattipakorn and S. C. Chattipakorn, Ovariectomy and obesity have equal impact in causing mitochondrial dysfunction and impaired skeletal muscle contraction in rats, Menopause, 2018, 25, 1448–1458 CrossRef.
-
Y. Zhou, Y. Tan, G. Hou, Y. Ren, Y. Deng, K. Yan, Y. Zhang, L. Lin, X. Lou and S. Liu, Pathway attenuation of fatty acid beta-oxidation in the skeletal muscle of a type 2 diabetic mouse model, Rapid Commun. Mass Spectrom., 2020, e8869 Search PubMed.
- J. Nishimura, T. Masaki, M. Arakawa, M. Seike and H. Yoshimatsu, Isoleucine prevents the accumulation of tissue triglycerides and upregulates the expression of PPARalpha and uncoupling protein in diet-induced obese mice, J. Nutr., 2010, 140, 496–500 CrossRef CAS.
Footnotes |
† Electronic supplementary information (ESI) available. See DOI: 10.1039/d0fo02156c |
‡ These authors contributed equally to this work. |
|
This journal is © The Royal Society of Chemistry 2021 |