DOI:
10.1039/D0FO01873B
(Paper)
Food Funct., 2021,
12, 397-407
The protective effects of the Ganoderma atrum polysaccharide against acrylamide-induced inflammation and oxidative damage in rats
Received
17th July 2020
, Accepted 25th November 2020
First published on 28th November 2020
Abstract
In this study, the protective effects of the Ganoderma atrum polysaccharide (PSG-1) on selected tissue (liver, spleen, kidneys and intestine) toxicity induced by acrylamide (AA) in SD rats were investigated. The results showed that pretreatment with PSG-1 could prevent AA-induced damage to liver and kidney functions by increasing the activities of ALT, AST and ALP and the levels of TG, BUN and CR in the serum of AA-treated rats. PSG-1 could also maintain the intestinal barrier function and permeability by preventing the reduction of the serum D-Lac and ET-1 levels in the intestine of AA-treated rats. In addition, AA-induced DNA damage, as indicated by an increase of the 8-OHdG level, was alleviated by pretreatment with PSG-1. Histological observations of the tissues confirmed the protective effects of different doses of PSG-1. Moreover, PSG-1 supplementation reduced oxidative stress and inflammation in rats by upregulating the superoxide dismutase (SOD), catalase (CAT), and glutathione peroxidase (GSH-Px) activities and IL-10 levels, and preventing the overproduction of malondialdehyde (MDA), IL-1β, IL-6, and TNF-α. Thus, these findings suggest that PSG-1 effectively prevents AA-induced damage in the liver, spleen, kidneys, and intestine of rats, partially by alleviating the inflammatory response and oxidative stress and protecting the intestinal integrity and barrier function.
1. Introduction
Acrylamide is a white solid powder that is easily soluble in water, ethanol and other solvents. It has been widely used in the water treatment industry, the textile industry, and printing and in the production of cosmetics.1 Since 2002, researchers have found that AA can be formed at high temperatures from carbohydrate-rich foods such as potatoes, bread, biscuits and grains.2 Therefore, to some extent, Chinese and western dietary habits expose residents to low doses of AA over long periods of time. Several studies, both in vivo and in vitro, have clearly revealed that it has mutagenic and neurotoxic effects in both animals and humans, and exposure to AA causes tumors of tunica vaginalis of testes and thyroid, and mammary fibroadenomas in rats.3,4 In recent years, various organ toxicities of acrylamide have been gradually reported. AA could cause inflammatory cell infiltration in the liver tissue, hepatocyte necrosis, elevated liver function enzymes, lipid accumulation, etc.5–7 It was reported that acrylamide produced significant pathological changes in the kidney of pregnant rats with acute tubular degeneration, hemorrhage, narrowing, and closure in Bowman's space.8 A study by Abdel-Daim et al.9 showed that AA intoxication induces hepatorenal injuries via oxidative stress, lipid peroxidation, DNA oxidative damage, and inflammatory responses, which is mainly because AA-induced reactive oxygen species (ROS) can attack cell membranes and cause serious damage to biomolecules, DNA, lipids and proteins. Similarly, our previous studies10 also showed that AA could induce oxidative damage by producing ROS and malondialdehyde (MDA) and by reducing the activities of superoxide dismutase (SOD) and glutathione peroxidase (GSH-Px) in IEC-6 cells. What is more, it also confirmed that AA induces apoptosis by inducing mitochondrial dysfunction, and then releases cytochrome C and activates the caspase apoptotic protein. It is known that oxidative stress plays a crucial role in AA-induced toxicity due to the overproduction of reactive oxygen species. Previous studies have shown that accumulation of AA induces oxidative damage to different organs of the body, including the brain, liver, and kidneys.11
Inhibiting the toxicity of AA and mitigating the associated risks by natural compounds has been widely investigated. Dietary supplementation of antioxidants may be a good way to combat AA toxicity. Recently, the protective effects of some antioxidant phytochemicals, such as quercetin, blueberry anthocyanin extract and crocin, on AA toxicity have been reported in vitro and in vivo because of their strong ability to scavenge ROS.1,12,13Ganoderma atrum is a traditional fungus that is edible and of medicinal value.14 Polysaccharide (PSG-1) is the major bioactive component of Ganoderma atrum. It is composed of glucose, mannose, galactose and galacturonic acid in a molar ratio of 4.91
:
1
:
1
:
28
:
0.71, and it is identified as a heteropolysaccharide formed as a main chain of β-1,3 glcp, β-1,6 glcp, α-1,4 galp, α-1,2 manp and α-1,4 manp residues substituted at the O-3 and O-6 positions as the branch points, with a high molecular weight of about 1013 kDa.15 It has been confirmed that PSG-1 has a variety of biological activities including antioxidant, immunomodulatory, intestinal protection, etc.10,16,17 Previous studies have shown that PSG-1 can improve the redox state of IEC-6 cells and reduce mitochondrial-mediated apoptosis to inhibit AA toxicity. PSG-1 also exhibits hypoglycemic and hypolipidemic effects by suppressing the expression of the Bax protein and promoting the expression of the Bcl-2 protein in the pancreatic tissue of type 2 diabetic rats.18 In addition, Zhu et al.19 showed that PSG-1 significantly improved the liver dysfunction in type 2 diabetic rats. However, whether PSG-1 has a protective effect on different organ toxicity induced by AA is still unknown.
The intestine is the major tissue for the digestion and absorption of acrylamide.20 The liver and kidneys are important organs for the detoxification and metabolism of acrylamide. As the largest immune organ of the human body, the spleen contains a variety of immune cells, such as T lymphocytes, B lymphocytes, macrophages, dendritic cells, etc. It is most suitable for observing the changes of immune cells’ response to inflammatory stimulation by acrylamide.21 Thus, the intestine, liver, kidneys and spleen have been selected as the major organs for AA induced toxicity in this study. The protective effect of PSG-1 on these tissues’ toxicities induced by AA was investigated by measuring serum hepatic and renal functional enzymes, DNA damage, intestinal permeability, oxidative damage indicators and inflammatory responses in tissues. This study will provide new evidence for the use of PSG-1 as an alternative dietary supplement against AA toxicity in vivo.
2. Materials and methods
2.1 Chemicals and reagents
PSG-1 (purity ≥99.8%) was prepared according to the laboratory method.22 Acrylamide (purity >99.0%) and N-acetylcysteine (NAC) were procured from Aladdin Chemical Co. ELISA kits for analyzing cytokines (IL-1β, IL-6, IL-10 and TNF-α) were obtained from Boster Biological Technology Co (Wuhan, China). SOD, BCA and MDA kits were bought from Beyotime Institute of Biotechnology (Nanjing, China). GSH-Px, CAT, AST, ALT, ALP, TG, BUN, CR, D-Lac, ET-1 and 8-OhdG kits were bought from the Nanjing Jiancheng Bioengineering Institute (Nanjing, China). All chemicals employed in this work were of analytical grade.
2.2 Animals and experimental procedures
A total of 60 male SD rats, weighing 160 to 180 g, were provided by Hunan SJA Laboratory Animal Co., Ltd (Certificate Number: SCXK (Xiang) 2016-0002, Hunan China). Before the beginning of the formal experiment, all rats were adapted to a light–dark cycle environment at 25 ± 2 °C for 12 hours for a week. All the experiments were in line with animal ethical standards. Sixty SD rats were randomly divided into six groups (10 per group). The control group was given normal saline, the model group was orally given 20 mg per kg bw AA, and the positive group was administered with N-acetylcysteine (NAC, 200 mg per kg bw). The other groups were administered with AA (20 mg per kg bw) and PSG-1 at doses of 50, 100, and 200 mg per kg bw for 30 days. Similarly, AA was given half an hour after oral administration of polysaccharide or NAC. The detailed experimental design is shown in Fig. 1A.
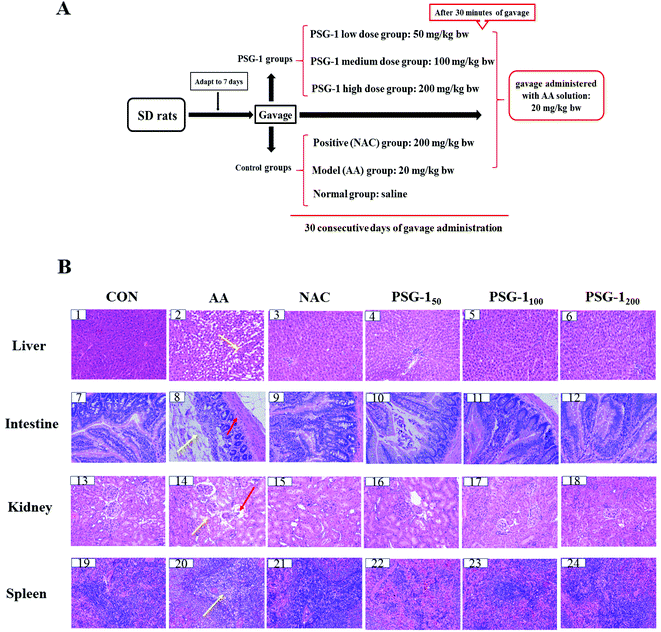 |
| Fig. 1 (A) Experimental protocol and design. (B) Histopathological examination of the liver, intestine, kidneys and spleen. Normal group (CON), acrylamide (AA) group, N-acetylcysteine (NAC) group and Ganoderma atrum polysaccharide (PSG-1, 200 mg per kg bw) group; HE staining; ×200. | |
2.3 Blood and tissue collection
At the end of the treatment, all rats were fasted for 12 h, followed by anesthetization using ether. The blood was collected from rat orbit and then the rats were sacrificed. The serum was allowed to stand at room temperature for 2 hours and centrifuged at 4000 rpm for 10 min at 4 °C. The supernatant was collected and stored at −80 °C until use. The liver, kidneys, spleen and the jejunum of the small intestine tissues were dissected and washed with ice isotonic saline buffer. Part of the tissues were fixed in the formaldehyde solution for morphological observation, and the rest of the tissues were snap-frozen in liquid nitrogen and then stored at −80 °C until use.
2.4 Histological examination
The liver, kidneys, intestine and spleen tissues of the dissected rats fixed with 10% formaldehyde solution were embedded in paraffin wax and sliced with a thickness of 6 μm. Haematoxylin and eosin (H&E) staining was performed according to the method described by Ahmed.23 A light microscope was used to observe the morphological changes.
2.5 Measurement of the liver- and kidney-related indexes
The blood samples were centrifuged at 4000 rpm for 10 min at 4 °C, and then the supernatants were obtained. The activities of the enzymes aspartate aminotransferase (AST), alanine aminotransferase (ALT), and alkaline phosphatase (ALP), and the triglyceride (TG), blood urea nitrogen (BUN), and creatinine (CR) levels were determined using commercial assay kits according to the manufacturers’ instructions.
2.6 Measurement of intestinal mucosal permeability
Serum samples were obtained to measure the levels of D-lactic acid (D-Lactate) and endothelin-1 (ET-1) using Assay Kits (Jiancheng Bioengineering Institute, Nanjing, China) according to the standard procedures provided by the manufacturers.
2.7 Evaluation of tissue lipid peroxidation and antioxidant enzyme activities
The tissue was mixed with normal saline at 1
:
10 (w/v), and a tissue breaker was used to crush the tissue for 60 s at 60 Hz. The supernatant was collected after centrifugation at 4 °C and 5000 rpm for 15 minutes. The activities of SOD, GPH-Px and CAT, and the level of MDA in the tissue samples were measured following the instructions provided in the commercial kits.
2.8 DNA oxidation
The level of the DNA oxidation biomarker (8-OHdG) in serum was determined using an 8-OhdG competitive assay kit (Jiancheng Bioengineering Institute, Nanjing, China) following the manufacturer's instructions.
2.9 Measurement of primary inflammatory cytokines
The levels of cytokines IL-1β, IL-6, TNF-α and IL-10 in serum were measured using commercially available ELISA kits (Boster Biological Co, Wuhan, China) according to the manufacturers’ instructions.
2.10 Statistical analysis
The data were reported as mean ± standard deviation (n = 8). The statistical significance of differences among groups was determined by one-way analysis of variance (ANOVA) corrected for multiple comparisons using Tukey's test. SPSS (version 22.0, IBM, Armonk, NY, USA) and GraphPad Prism 6 were used for drawing and data analysis, respectively. p < 0.05 was considered statistically significant.
3. Results
3.1 Histological results
The effects of PSG-1 and NAC on the liver, intestine, kidneys and spleen histology of AA-treated rats are shown in Fig. 1. It was observed that (Fig. 1B) the liver of the CON group had a normal histological structure, and the cells were identical in size with clear nuclei (Fig. 1B, 1). In contrast, the treatment with AA resulted in necrosis of hepatocytes, gross mononuclear infiltration, and loss of cellular boundaries of the hepatocytes and massive loss of the cytoplasm (Fig. 1B, 2). Pretreatment with 200 mg kg−1 NAC and 50 mg kg−1 PSG-1 showed slight amelioration of the histopathological morphology truncated by AA intoxication (Fig. 1B, 3 and 4). An area of localized and complete necrosis was still observed, indicating that the hepatocyte damage induced by AA was not yet fully resolved at this dose of NAC and low dosage of PSG-1 (Fig. 1B, 3 and 4). Upon pretreatment with PSG-1 at higher doses of 100 and 200 mg kg−1, normal hepatic histology was restored, and liver tissue with numerous mitotic cells was observed (Fig. 1B, 5 and 6), demonstrating hepatocyte regeneration and that PSG-1 is hepatoprotective at this dosage.
HE staining of the intestinal sections showed normal intestinal mucosa with intact intestinal villi in the control group (Fig. 1B, 7). Severe mucosal injury, manifested by necrosis of epithelial cells, wider villi spacing, and disordered intestinal villi, was observed in the AA group (Fig. 1B, 8). The severity of the intestinal mucosal injury in the rats pretreated with PSG-1 and NAC was evidently lower than that of the AA group. In particular, more regularly aligned and extensive microvilli were observed in the rats treated with PSG-1 at high doses (Fig. 1B, 11 and 12).
As shown in Fig. 1B, 13, the kidney sections of the control rats displayed a normal renal tissue structure, complete renal tubular epithelial cells and no obvious pathological changes in the glomerular or renal interstitium, whereas severe pathological damage to the kidneys such as kidney tubule cavity expansion, renal tubular epithelial cell degeneration and renal interstitial inflammatory cell infiltration was observed in the AA treated rats (Fig. 1B, 14). The higher dose PSG-1 treatment could significantly relieve the severity of renal lesion injury (Fig. 1B, 17 and 18).
The spleen sections of the control rats showed that red medulla and white medulla were well demarcated and the margins were clear and no abnormality was observed (Fig. 1B, 19). The typical pathological damage to the spleen tissue such as the disorder of the white pulp and red pulp structures, no apparent boundaries, significantly decreased lymphocytes and moderate to severe white pulp depletion was induced by AA (Fig. 1B, 20). After administration of NAC and PSG-1, the typical pathological damage was reversed (Fig. 1B, 21–24).
The above results suggested that NAC and PSG-1 could significantly reduce the pathological damage to liver, kidneys, intestine and spleen caused by AA.
3.2 Effect of PSG-1 on hepatic and kidney functions in AA-induced rats
In the current study, the level of TG and the activities of AST, ALT, and ALP were significantly increased (P < 0.01) in the serum of AA group animals, compared with the normal group, while their activities were significantly (P < 0.05) reduced after pretreatment with NAC and different doses of PSG-1 (Fig. 2a, b and c). As shown in Fig. 2d, compared with the AA group, the level of TG was also markedly decreased after PSG-1 intervention (P < 0.01). The effects were even superior to those of NAC. The above results suggested that AA could induce liver damage in rats, and that the different doses of PSG-1 had obvious protective effects on the AA induced liver damage. Similarly, after administration of AA, the BUN and CR levels were significantly (P < 0.05) increased compared with those in the normal group (Fig. 2e and f), which indicated that renal function injury occurred in rats, whereas the NAC and PSG-1 treatment could significantly alleviate this injury by reducing the serum BUN and CR levels to those comparable to the control group.
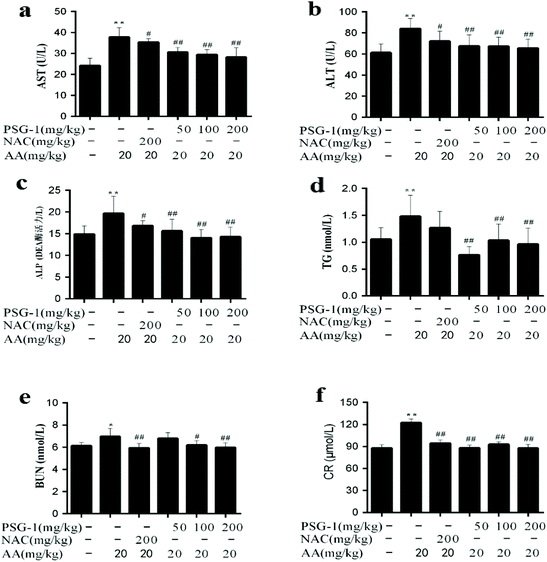 |
| Fig. 2 Effect of PSG-1 on the serum levels of AST (a), ALT (b), ALP (c), TG (d), BUN (e) and CR (f) in AA-induced rats. The data are presented as mean ± SD (n = 8). **P < 0.01, AA group versus normal group; #P < 0.05 and ##P < 0.01, NAC and PSG-1 groups versus AA group. | |
3.3 Effect of PSG-1 on the intestinal permeability in AA-induced rats
As shown in Fig. 3, compared with the control group, the levels of ET-1 and D-Lac in the serum of the AA treated group were significantly increased by 75% (P < 0.01), while PSG-1 significantly reduced the ET-1 and D-Lac levels in serum as expected at all doses (p < 0.01). In particular, the reduction effects of PSG-1 at doses of 50 and 100 mg kg−1 were comparable to that of NAC at a dose of 200 mg kg−1. These results suggested that PSG-1 could alleviate the small intestinal mucosal injury caused by AA.
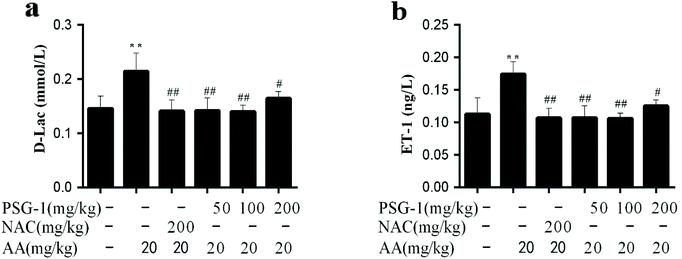 |
| Fig. 3 Effect of PSG-1 on the serum levels of D-Lac (a) and ET-1 (b) in AA-induced rats. The data are presented as mean ± SD (n = 8). **P < 0.01, AA group versus normal group; #P < 0.01 and ##P < 0.01, NAC and PSG-1 groups versus AA group. | |
3.4 Effect of PSG-1 on the DNA damage in AA-induced rats
The present study was intended to assess the oxidative DNA damage in AA-induced rats using serum 8-OHdG as a biomarker. It was found that the content of 8-OHdG in the serum of AA-treated rats was significantly higher than that in the control group, while in the NAC and PSG groups the AA-mediated increase of the 8-OHdG level was significantly prevented (Fig. 4), suggesting that the oxidative DNA damage caused by AA could be significantly relieved (P < 0.01) by NAC and PSG-1 pretreatment.
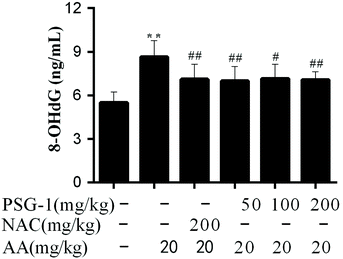 |
| Fig. 4 Effect of PSG-1 on the level of 8-OHdG induced by AA. The data are presented as mean ± SD (n = 8). **P < 0.01, AA group versus normal group; #P < 0.01 and ##P < 0.01, NAC and PSG-1 groups versus AA group. | |
3.5 Effect of PSG-1 on the activities of SOD, GSH-Px and CAT, and the MDA level in the liver, kidneys, intestine and spleen
The activities of SOD, CAT and GSH-Px enzymes and the MDA levels are often used as indicators of tissue oxidative damage. The results showed that the level of MDA was significantly increased (P < 0.01) in the liver, kidneys, intestine and spleen (Table 1), whereas the activity of SOD was significantly decreased (P < 0.01) in the liver, kidneys, intestine and spleen after AA administration (Table 1). The CAT activities of the AA treated group were also significantly decreased (P < 0.01) in the kidneys and intestine (Table 2). Similarly, the GSH-Px activities of the AA treated group were also significantly (P < 0.01) decreased in the liver and spleen (Table 2). The administration of both NAC and PSG-1 reversed the alterations in the SOD, CAT and GSH-Px activities and MDA levels. The above results suggested that AA could induce oxidative stress in rats, and that the different doses of PSG-1 had obvious protective effects on AA-induced oxidative damage.
Table 1 Effects of PSG-1 on SOD activities in the kidneys, liver, intestine and spleen of rats
|
Control |
AA |
NAC |
AA + PSG-150 |
AA + PSG-1100 |
AA + PSG-1200 |
AA: Acrylamide at a dose of 20 mg kg−1; PSG-1: Ganoderma atrum polysaccharide at doses of 50 mg kg−1, 100 mg kg−1, and 200 mg kg−1. The data are presented as mean ± SD (n = 8). **P < 0.01, AA group versus normal group; #P < 0.01 and ##P < 0.01, NAC and PSG-1 groups versus AA group. |
SOD (U mg−1 pro) |
Liver |
63.21 ± 13.72 |
37.64 ± 3.43** |
53.45 ± 5.52# |
36.19 ± 4.15 |
37.24 ± 10.15 |
47.40 ± 3.83# |
Kidneys |
46.76 ± 5.50 |
34.19 ± 2.21** |
52.37 ± 5.59## |
49.14 ± 2.34## |
48.57 ± 4.73## |
48.95 ± 7.38## |
Intestine |
96.31 ± 17.99 |
63.18 ± 6.73** |
81.29 ± 14.71## |
101.27 ± 15.06## |
90.69 ± 20.69## |
90.81 ± 14.61## |
Spleen |
23.22 ± 3.69 |
17.01 ± 2.16** |
17.20 ± 1.52 |
21.33 ± 3.55# |
26.19 ± 4.67## |
27.76 ± 4.80## |
|
MDA (U mg−1 pro) |
Liver |
0.27 ± 0.02 |
0.38 ± 0.05** |
0.24 ± 0.04## |
0.27 ± 0.03## |
0.21 ± 0.01## |
0.23 ± 0.03## |
Kidneys |
1.13 ± 0.21 |
1.65 ± 0.13** |
1.34 ± 0.22# |
1.29 ± 0.17## |
1.33 ± 0.38# |
1.15 ± 0.20## |
Intestine |
0.21 ± 0.03 |
0.29 ± 0.06** |
0.19 ± 0.01## |
0.18 ± 0.02## |
0.16 ± 0.01## |
0.13 ± 0.02## |
Spleen |
0.43 ± 0.11 |
0.59 ± 0.12** |
0.39 ± 0.04## |
0.47 ± 0.13# |
0.54 ± 0.14 |
0.47 ± 0.03# |
Table 2 Effects of PSG-1 on CAT activities in the kidneys and intestine, and GSH-Px activities in the spleen and liver of rats
Groups |
CAT (U mg−1 pro) |
GSH-Px (U mg−1 pro) |
Kidneys |
Intestine |
Liver |
Spleen |
AA: Acrylamide at a dose of 20 mg kg−1; PSG-1: Ganoderma atrum polysaccharide at doses of 50 mg kg−1, 100 mg kg−1, and 200 mg kg−1. The data are presented as mean ± SD (n = 8). **P < 0.01, AA group versus normal group; #P < 0.01 and ##P < 0.01, NAC and PSG-1 groups versus AA group. |
Control |
996.65 ± 146.31 |
518.93 ± 115.22 |
1140.97 ± 238.74 |
253.27 ± 54.83 |
AA |
705.88 ± 145.19** |
347.92 ± 118.44** |
655.65 ± 36.46** |
127.08 ± 39.02** |
NAC |
877.62 ± 156.38 |
449.87 ± 68.76# |
783.95 ± 143.49 |
206.29 ± 56.80 |
AA + PSG-150 |
977.56 ± 169.58# |
576.95 ± 50.57# |
839.24 ± 58.64# |
158.51 ± 26.56 |
AA + PSG-1100 |
948.44 ± 193.33# |
584.25 ± 60.08## |
895.77 ± 112.93## |
279.10 ± 152.25## |
AA + PSG-1200 |
993.66 ± 235.00## |
609.77 ± 105.78## |
913.63 ± 73.86## |
305.21 ± 27.21## |
3.6 Effect of PSG-1 on inflammatory cytokines in AA-induced rats
To explore the role of inflammatory cytokines in mediating the protective effect of PSG-1 on AA-induced rats, their expression in serum was quantified (Fig. 5). The oral administration of AA caused a significant increase (P < 0.01) in the levels of the pro-inflammatory cytokines TNF-a, IL-1β and IL-6 compared with the control group. The rats pretreated with PSG-1 and NAC showed a significant decrease in the production of TNF-a, IL-1b and IL-6 in serum as compared with the rats treated with AA (Fig. 5a–c). At the same time, the level of the anti-inflammatory factor IL-10 was significantly decreased (P < 0.01) in the AA group, compared with the normal group, whereas NAC and PSG-1 administration reversed the alteration of those cytokines (Fig. 5d). These results suggest that PSG-1 treatment can significantly inhibit the levels of the inflammatory factors IL-1β, IL-6 and TNF-α in serum and increase the level of the anti-inflammatory factor IL-10, which effectively prevents the AA-induced inflammatory response.
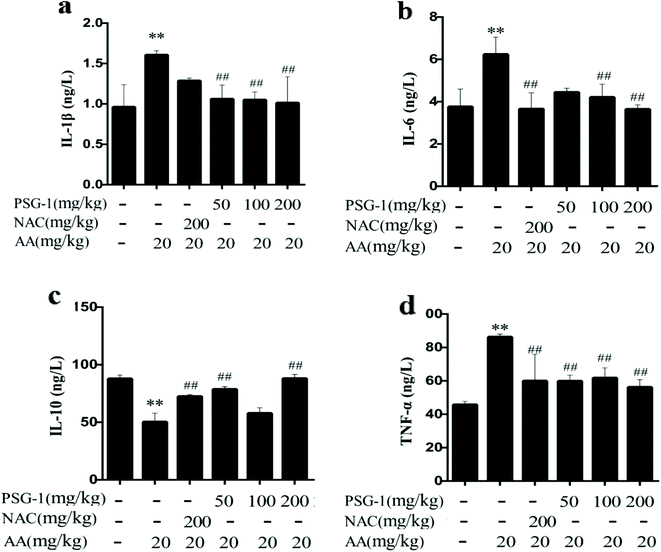 |
| Fig. 5 Effect of PSG-1 on the IL-1β (a), IL-6 (b), IL-10 (c) and TNF-α (d) levels induced by AA. The data are presented as mean ± SD (n = 8). **P < 0.01, AA group versus normal group; #P < 0.01 and ##P < 0.01, NAC and PSG-1 groups versus AA group. | |
4. Discussion
AA is a water-soluble toxic substance with strong permeability, and it can quickly reach the intestinal tract during digestion and may cause intestinal injury.20,24 It can also easily get into the bloodstream and may trigger liver and kidney damage. AA can be metabolized by two main pathways: via epoxidation by cytochrome P450 to form reactive glycidamide and the non-enzymatic and enzymatic GST catalyzed formation of conjugates with GSH.25 The latter accounts largely for the changes in the cells’ redox status induced by AA exposure and finally leads to damaged cell function and death. With the continuous intake of AA and the depletion of GSH, AA is mostly metabolized by CYP2E1 to glycidamide with greater toxicity.26 Glycidamide could also conjugate with GSH or its epoxide group may undergo hydrolysis. The excessive consumption of GSH eventually leads to an imbalance of the intracellular oxidative balance system and causes systemic oxidative tissue damage. What is more, glycidamide is believed to have a role in the genotoxicity of acrylamide by attaching itself to the DNA.27–29 Recently, a study by Elhelaly et al.30 indicated that acrylamide-induced toxicity caused damage and injury to the liver, kidneys, and brain tissues through the increase of DNA oxidative damage and the reduction of the antioxidant enzyme activities. Previously, we have reported that PSG-1 had protective effects on the oxidative damage and apoptosis of epithelial cells induced by AA. Here, we explore the overall positive effects of PSG-1 supplementation on AA induced multiple tissue (intestine, liver, kidneys, and spleen) and DNA damage, which has not been studied yet.
The serum D-lactic acid level can reflect the extent of intestinal mucosal barrier damage.31D-Lactic acid is the product of bacterial fermentation, metabolism and pyrolysis. Being a special end product of fermentation, metabolism and lysis of intestinal bacteria, intestinal D-lactic acid will be released into the blood through the damaged mucosa when the intestinal mucosa is damaged and its permeability is increased. ET-1 is believed to be an endogenous pathogenic factor released by the intestinal tissue during ischemia, hypoxia and injury. It can cause a mesenteric vein contraction reaction, gastrointestinal ischemia, and hypoxia and lead to gastrointestinal mucosal injury.32,33 Consistent with the previous observation, our data revealed that PSG-1 supplementation decreased the levels of D-Lac and ET-1 in the serum of AA treated rats and improved the morphology and histology of the intestine, indicating that PSG-1 showed positive effects on the mechanical barriers of the intestine.24
The liver is the target organ of exogenous toxicants, and it is more vulnerable and susceptible to the toxicities of these toxicants and plays a key role in the detoxification process. AST and ALT are widely distributed in tissues and are particularly active in the liver, heart muscle, skeletal muscle, and kidneys. In particular, ALT is the most specific liver injury marker and a more selective liver parenchymal enzyme.34 AST is the mitochondrial damage index, and ALT indicates the membrane damage. During the process of hepatotoxicity, the damaged liver cells release liver specific enzymes into the bloodstream, such as ALT and AST. Gedik et al.1 reported that AST and ALT activities were significantly increased in the serum of rats treated with AA. In line with the previous observation, we found that the activities of serum transaminase were significantly increased in rats treated with AA. Moreover, the results from the present study showed that TG and ALP activities were increased after AA exposure. The increased ALP activity has been associated with drug-induced cholestasis.35 It is primarily a marker of hepatobiliary function and moderate or severe cholestasis caused by liver damage.36,37 In line with our findings, a recent study by Abukhalil et al.38 showed elevated levels of serum biomarkers (such as ALP and TG) in rats with liver injury. Interestingly our histological and biochemical analysis results showed that the serum levels of those biomarker enzymes and the TG level returned to near-normal levels with the regeneration of hepatocytes. This result suggests that PSG-1 accelerates the healing process of the liver.
The kidneys are important organs that excrete metabolites and exogenous harmful substances from the body. BUN and CR are widely accepted as the most common parameters to assess renal functions.39 BUN is a major nitrogenous end product of protein and amino acid catabolism, and CR is a breakdown product of creatine phosphate in muscle which is excreted by the kidneys.40 Increased levels of CR and BUN in serum have been reported to indicate kidney dysfunction.41 Consistent with the results of the study by Erdemli et al.,7 the present study showed that the serum BUN and CR levels were significantly increased in rats with AA-induced renal injury. In this study, after supplementation of PSG-1, the levels of BUN and CR in AA-treated rats returned to levels similar to those in the control group.
Subsequently, due to kidney damage and dysfunction, more metabolites cannot be expelled from the body, resulting in systemic tissue oxidative damage. Emerging evidence has confirmed that AA could destroy the oxidant/antioxidant balance accompanied by excessive production of reactive oxygen species (ROS), thus resulting in oxidative damage.42,43 SOD, CAT and GSH-Px are enzymes that are involved in the endogenous antioxidant stress defense system and play an important role in combating the harmful physiological effects of lipid peroxidation and hydrogen peroxide.44 Consistent with the studies by Zhao et al.45 and Gangarapu et al.,46 our present study found that AA-induced oxidative stress in tissues of rats with an evident decrease in SOD, CAT and GSH-Px activities. In contrast, the level of MDA, which is an important biomarker of oxidative stress, was significantly increased after AA treatment. It is also in agreement with the findings of several other recent studies by Abdel-Daim et al.9 and Erdemli et al.,7 which have shown that AA has deleterious effects on tissues because it increases the incidence of lipid peroxidation and alters the antioxidant enzyme systems. As observed in this study, there is a reduction in the activities of SOD, GSH-Px, and CAT in tissues and a dramatic increase in MDA levels. Daily supplementation of PSG-1 in AA treated rats can improve the activities of these antioxidant enzymes, indicating that PSG-1 has a strong antioxidant capacity. Accordingly, the amount of lipid peroxidation product (MDA) was significantly reduced after PSG-1 administration. Oxidative stress could activate various transcription factors, such as AP-1, NF-κB and p53, which then caused inflammation.47 As the largest immune organ, the spleen plays a central role in mounting a response against systemic inflammation.48 However, in our present study, the spleen sections showed the disorder of red and white medulla and a significant decrease of lymphocytes, which indicate that the immune system was damaged in rats. Furthermore, lymphocytes are the single most abundant source of the inflammatory cytokine TNF-α when the spleen was injured,49 and TNF-α can act as an important initiating factor to induce a waterfall-like release of cytokines such as IL-6 and IL-1β50 in a variety of cells. In the present study, AA caused the inflammatory response in rats by up-regulating the expression levels of the pro-inflammatory cytokines IL-6, IL-1β and TNF-α and by suppressing the level of the anti-inflammatory factor IL-10, which was in accordance with the results of studies by Ince et al.47 and Ansar et al.12 PSG-1 could ameliorate the alterations of these pro-inflammatory cytokines and the anti-inflammatory cytokine. Moreover, PSG-1 also reversed a significant increase in the 8-OHdG level during AA intake. 8-OHdG, one of the byproducts of oxidative DNA damage, is physiologically formed and enhanced by chemical carcinogens. In line with our findings, a recent study by Hamdy et al.51 has demonstrated a significant increase in the serum levels of biomarkers of liver and kidney injury, oxidative DNA damage markers, and proinflammatory cytokines after AA exposure in rats.
As mentioned before, the antioxidant PSG-1 is a natural product similar to polyphenol, flavone compounds, etc. that may suppress AA toxicity via several mechanisms. The antioxidant properties of PSG-1 may be mediated by involving direct radical scavenging, improving the cellular antioxidant defense and inhibiting apoptosis in mitochondria and producing short-chain fatty acids (SCFA). It was reported that blueberry anthocyanin markedly inhibited ROS formation, relieved oxidative stress in the liver, and stabilized the mitochondrial membrane potential, which inhibited the release of cytochrome C, and then effectively alleviated cell apoptosis and ultimately weakened the toxicity of AA.45 In line with our previous research, we demonstrated that PSG-1 could also reverse the oxidative damage in IEC-6 cells caused by AA through this pathway in vitro.10 Heme oxygenase-1 (HO-1) is an important antioxidant enzyme, which shows a significant anti-oxidative stress effect.52 Furthermore, according to Elavarasan et al.53 hesperidin can up-regulate the expression of HO-1 in rats by promoting the expression of gene nuclear factor-erythrocyte 2p45 (Nrf2), which improves the antioxidant capacity. In addition, Nrf2 is essential for mediating AA-induced oxidative damage and it can increase the activities of antioxidant enzymes in the cells, such as SOD, CAT and GSH-Px.54 Thus, the powerful antioxidant PSG-1 may be similar to hesperidin, enhancing rats’ cellular antioxidant capacity by acting on Nrf2 and inducing HO-1 expression. In terms of inflammation, it was observed that treatment of breast epithelial cells with acrylamide increased the expression levels of inducible nitric oxide synthase (iNOS) and cycloogenase-2 (Cox-2) and NOS activities. PSG-1 has the capacity to block the NF-κB and MAPK signaling pathways, which are responsible for the regulation of enzymes (iNOS and COX2) and cytokines, such as TNF-α, IL-1β, and IL-6, associated with inflammation.55 In the present study, the supplementation of AA intoxicated rats with PSG-1 maintained the serum concentrations of inflammatory biomarkers and DNA oxidation markers within normal levels. A study by Zhu et al.18 indicated that PSG-1 significantly enhances short-chain fatty acid (SCFAs) excretion in the colon. SCFAs (such as acetic acid, propionic acid, and butyric acid) are major metabolites produced by microbial fermentation of complex dietary carbohydrate fibers in the gut. Some reports indicated that SCFAs can maintain liver metabolism and improve the gluconeogenesis cycle and can be used as the main energy source of intestinal epithelial cells; what is more, it is of great significance for maintaining the intestinal mucosal mechanical barrier function.56,57 On the other hand, SCFAs can participate in immune regulation by acting on mononuclear phagocytes, lymphocytes and other immune cells. In particular, butyric acid can induce T cells to differentiate into regulatory T cells, which can inhibit the inflammatory response by promoting the release of the anti-inflammatory factor IL-10 and inhibiting the proliferation and activation of effector T cells.58 In conclusion, the protective effects of PSG-1 may be closely related to the generation of SCFAs in addition to its strong antioxidant and immunomodulatory properties.
Overall, this research is the first study to comprehensively describe the organ toxicity caused by AA, and PSG-1 prophylaxis effectively alleviates its toxicity. The mechanisms of action might be closely related to the improvement of intestinal permeability, regulation of liver and kidney functions, reduction of oxidative stress, inflammatory response and DNA damage, and regulation of intestinal permeability. in rats. Thus, dietary supplementation of PSG-1 to reduce the risk associated with AA is a good approach. Further studies will reveal the molecular mechanism more systematically and comprehensively through the techniques of metabolomics and proteomics.
Ethical statement
All animal (mice) experiments in this manuscript were conducted following the approved protocol and license (SYKX-2015-0001) by the Animal Care Review Committee of Nanchang University.
Conflicts of interest
We have no conflicts of interest to declare.
Acknowledgements
The financial support from the National Key Research and Development Program of China (2019YFE0106000 and 2017YFC1600405), the National Natural Science Foundation of China (No: 31471647 and 21866021), and the Postgraduate Innovation Special Fund Project of Jiangxi Province (YC2018-S010) is gratefully acknowledged.
References
- S. Gedik, M. E. Erdemli, M. Gul, B. Yigitcan, H. G. Bag, Z. Aksungur and E. Altinoz, Hepatoprotective effects of crocin on biochemical and histopathological alterations following acrylamide-induced liver injury in Wistar rats, Biomed. Pharmacother., 2017, 95, 764–770 CrossRef CAS PubMed.
- T. Eden, R. Per, K. Patrik, E. Sune and T. R. Margareta, Analysis of acrylamide, a carcinogen formed in heated foodstuffs, J. Agric. Food Chem., 2002, 50, 4998–5006 CrossRef PubMed.
- M. A. Friedman, L. H. Dulak and M. A. Stedham, A lifetime oncogenicity study in rats with acrylamide, Toxicol. Sci., 1995, 27, 95 CrossRef CAS.
- M. E. Erdemli, M. A. Aladag, E. Altinoz, S. Demirtas, Y. Turkoz, B. Yigitcan and H. G. Bag, Acrylamide applied during pregnancy causes the neurotoxic effect by lowering BDNF levels in the fetal brain, Neurotoxicol. Teratol., 2018, 67, 37–43 CrossRef CAS PubMed.
- S. Siahkoohi, M. Anvari, M. A. Tafti and M. Hosseini-sharifabad, The effects of vitamin E on the liver integrity of mice fed with acrylamide diet, Iran. J. Pathol., 2014, 9, 89–98 Search PubMed.
- A. A. Allam, A. W. El-Ghareeb, M. Abdul-Hamid, A. E. Bakery, M. Gad and M. Sabri, Effect of prenatal and perinatal acrylamide on the biochemical and morphological changes in liver of developing albino rat, Arch. Toxicol., 2010, 84, 129–141 CrossRef CAS PubMed.
- M. E. Erdemli, E. Altinoz, Z. Aksungur, Y. Turkoz, Z. Dogan and H. G. Bag, Biochemical investigation of the toxic effects of acrylamide administration during pregnancy on the liver of mother and fetus and the protective role of vitamin E, J. Matern.-Fetal Neonat. Med., 2017, 30, 844–848 CrossRef CAS PubMed.
- E. M. Erman, A. Zeynep, G. Mehmet, Y. Birgul, B. H. Gozukara, A. Eyup and T. Yusuf, The effects of acrylamide and vitamin E on kidneys in pregnancy: an experimental study, J. Matern.-Fetal Neonat. Med., 2018, 1–10 Search PubMed.
- M. M. Abdel-Daim, F. I. A. El-Ela, F. K. Alshahrani, M. Bin-Jumah and S. Alkahtani, Protective effects of thymoquinone against acrylamide-induced liver, kidney and brain oxidative damage in rats, Environ. Sci. Pollut. Res., 2020, 27, 37709–37717 CrossRef CAS PubMed.
- G. Jiang, L. Zhang, H. Wang, Q. Chen, X. Wu, X. Yan, Y. Chen and M. Xie, Protective effects of a Ganoderma atrum polysaccharide against acrylamide induced oxidative damage via a mitochondria mediated intrinsic apoptotic pathway in IEC-6 cells, Food Funct., 2018, 9, 1133–1143 RSC.
- A. E. Elhelaly, G. Albasher, S. Alfarraj, R. Almeer and M. M. Abdel-Daim, Protective effects of hesperidin and diosmin against acrylamide-induced liver, kidney, and brain oxidative damage in rats, Environ. Sci. Pollut. Res., 2019, 26, 35151–35162 CrossRef CAS PubMed.
- S. Ansar, N. J. Siddiqi, S. Zargar, M. A. Ganaie and M. Abudawood, Hepatoprotective effect of Quercetin supplementation against Acrylamide-induced DNA damage in wistar rats, BMC Complementary Altern. Med., 2016, 16, 327 CrossRef PubMed.
- S. Gedik, M. E. Erdemli, M. Gul, B. Yigitcan, H. G. Bag, Z. Aksungur and E. Altinoz, Investigation of the protective effects of crocin on acrylamide induced small and large intestine damage in rats, Biotech. Histochem., 2018, 93, 267–276 CrossRef CAS PubMed.
- H. Wang, Q. Yu, X. Ding, X. Hu, K. Hou, X. Liu, S. Nie and M. Xie, RNA-seq based elucidation of mechanism underlying Ganoderma atrum polysaccharide induced immune activation of murine myeloid-derived dendritic cells, J. Funct. Foods, 2019, 55, 104–116 CrossRef CAS.
- H. Zhang, W. Li, S. Nie, Y. Chen, Y. Wang and M. Xie, Structural characterisation of a novel bioactive polysaccharide from
Ganoderma atrum, Carbohydr. Polym., 2012, 88, 1047–1054 CrossRef CAS.
- Q. D. Xiang, Q. Yu, H. Wang, M. M. Zhao, S. Y. Liu, S. Nie and M. Y. Xie, Immunomodulatory activity of Ganoderma atrum polysaccharide on purified T lymphocytes through Ca2+/CaN and MAPK pathway based on RNA-seq, J. Agric. Food Chem., 2017, 65, 5306–5315 CrossRef CAS PubMed.
- Y. Qiang, N. Shao-Ping, W. Jun-Qiao, H. Dan-Fei, L. Wen-Juan and X. Ming-Yong, Toll-like Receptor 4 Mediates the Antitumor Host Response Induced by Ganoderma atrum Polysaccharide, J. Agric. Food Chem., 2015, 63, 517–525 CrossRef PubMed.
- K. Zhu, S. Nie, C. Li, S. Lin, M. Xing, W. Li, D. Gong and M. Xie, A newly identified polysaccharide from Ganoderma atrum attenuates hyperglycemia and hyperlipidemia, Int. J. Biol. Macromol., 2013, 57, 142–150 CrossRef CAS PubMed.
- K. X. Zhu, S. P. Nie, L. Tan, C. Li, D. Gong and M. Y. Xie, A polysaccharide from Ganoderma atrum improves liver function in type 2 diabetic rats via antioxidant action and short-chain fatty acids excretion, J. Agric. Food Chem., 2016, 64, 1938 CrossRef CAS PubMed.
- B. Zödl, D. Schmid, G. Wassler, C. Gundacker, V. Leibetseder, T. Thalhammer and C. Ekmekcioglu, Intestinal transport and metabolism of acrylamide, Toxicology, 2007, 232, 99–108 CrossRef PubMed.
- K. H. I. N. M. Herath, J. Cho, A. Kim, H.-S. Kim, E. J. Han, H. J. Kim, M. S. Kim, G. Ahn, Y.-J. Jeon and Y. Jee, Differential modulation of immune response and cytokine profiles of Sargassum horneri ethanol extract in murine spleen with or without Concanavalin A stimulation, Biomed. Pharmacother., 2019, 110, 930–942 CrossRef CAS PubMed.
- Y. Chen, M. Y. Xie, S. P. Nie, C. Li and Y. X. Wang, Purification, composition analysis and antioxidant activity of a polysaccharide from the fruiting bodies of Ganoderma atrum, Food Chem., 2008, 107, 231–241 CrossRef CAS.
- N. G. Halford, T. Y. Curtis, Z. Chen and J. Huang, Effects of abiotic stress and crop management on cereal grain composition: implications for food quality and safety, J. Exp. Bot., 2014, 66, 1145–1156 CrossRef PubMed.
- Y. Yang, L. Zhang, G. Jiang, A. Lei, Q. Yu, J. Xie and Y. Chen, Evaluation of the protective effects of Ganoderma atrum polysaccharide on acrylamide-induced injury in small intestine tissue of rats, Food Funct., 2019, 10, 5863–5872 RSC.
- M. M. Abdel-Daim, M. A. A. Eldaim and A. G. Hassan, Trigonella foenum-graecum ameliorates acrylamide-induced toxicity in rats: Roles of oxidative stress, proinflammatory cytokines, and DNA damage, Biochem. Cell Biol., 2015, 93, 192–198 CrossRef CAS PubMed.
- S. Sumner, C. C. Williams, R. W. Snyder, W. L. Krol, B. Asgharian and T. R. Fennell, Acrylamide: a comparison of metabolism and hemoglobin adducts in rodents following dermal, intraperitoneal, oral or inhalation exposure, Toxicol. Sci., 2003, 75, 260–270 CrossRef CAS PubMed.
- S. C. Sumner, T. R. Fennell, T. A. Moore, B. Chanas, F. Gonzalez and B. I. Ghanayem, Role of cytochrome P450 2E1 in the metabolism of acrylamide and acrylonitrile in mice, Chem. Res. Toxicol., 1999, 12, 1110–1116 Search PubMed.
- B. I. Ghanayem, H. Wang and S. Sumner, Using cytochrome P-450 gene knock-out mice to study chemical metabolism, toxicity, and carcinogenicity, Toxicol. Pathol., 2000, 28, 839–850 CrossRef CAS PubMed.
- I.-D. Adler, A. Baumgartner, H. Gonda, M. Friedman and M. Skerhut, 1-Aminobenzotriazole inhibits acrylamide-induced dominant lethal effects in spermatids of male mice, Mutagenesis, 2000, 15, 133–136 CrossRef CAS PubMed.
- A. E. Elhelaly, G. Albasher, S. A. Alfarraj, R. S. Almeer, E. I. Bahbah, M. Fouda, S. G. Bungău, L. Aleya and M. M. Abdeldaim, Protective effects of hesperidin and diosmin against acrylamide-induced liver, kidney, and brain oxidative damage in rats, Environ. Sci. Pollut. Res., 2019, 26, 35151–35162 CrossRef CAS PubMed.
- Z. S. Wen, Z. Tang, L. Ma, T. L. Zhu, Y. M. Wang, X. W. Xiang and B. Zheng, Protective Effect of Low Molecular Weight Seleno-Aminopolysaccharide on the Intestinal Mucosal Oxidative Damage, Mar. Drugs, 2019, 17, 64 CrossRef CAS PubMed.
- S. Massberg, M. Boros, R. Leiderer, L. Baranyi, H. Okada and K. Messmer, Endothelin (ET)-1 induced mucosal damage in the rat small intestine: role of ET (A) receptors, Shock, 1998, 9, 177–183 CrossRef CAS PubMed.
- B. K. Oktar, M. A. Gülpinar, A. Bozkurt, S. Ghandour, S. U. Çetinel, H. Moini, B. C. Yeğen, S. Bilsel, D. N. Granger and H. Kurtel, Endothelin receptor blockers reduce I/R-induced intestinal mucosal injury: role of blood flow, Am. J. Physiol.: Gastrointest. Liver Physiol., 2002, 282, G647–G655 CrossRef CAS PubMed.
- R. B. Gurung, B. Purbe, P. Gyawali and P. Risal, The Ratio of Aspartate Aminotransferase to Alanine Aminotransferase (AST/ALT): the Correlation of Value with Underlying Severity of Alcoholic Liver Disease, Kathmandu Univ. Med. J., 2015, 11, 233–236 CrossRef PubMed.
- A. Singh, T. K. Bhat and O. P. Sharma, Clinical biochemistry of hepatotoxicity, J. Clin. Toxicol., 2011, 4, 1–9 Search PubMed.
- S. K. Ramaiah, A toxicologist guide to the diagnostic interpretation of hepatic biochemical parameters, Food Chem. Toxicol., 2007, 45, 1551–1557 CrossRef CAS.
- T. M. Wright and A. M. Vandenberg, Risperidone-and quetiapine-induced cholestasis, Ann. Pharmacother., 2007, 41, 1518–1523 CrossRef CAS.
- M. H. Abukhalil, O. E. Hussein, M. Bin-Jumah, S. A. M. Saghir, M. O. Germoush, H. A. Elgebaly, N. M. Mosa, I. Hamad, M. M. Qarmush, E. M. Hassanein, M. K. Emadeldin, R. Hernandez-Bautista and A. M. Mahmoud, Farnesol attenuates oxidative stress and liver injury and modulates fatty acid synthase and acetyl-CoA carboxylase in high cholesterol-fed rats, Environ. Sci. Pollut. Res., 2020, 27, 30118–30132 CrossRef CAS PubMed.
- G. Suresh, A. Ravi Kiran, Y. Samata, N. Purnachandrarao Naik and A. Vijay Kumar, Analysis of blood and salivary urea levels in patients undergoing haemodialysis and kidney transplant, J. Clin. Diagn. Res., 2014, 8, ZC18–ZC20 Search PubMed.
- S. Gowda, P. B. Desai, S. S. Kulkarni, V. V. Hull, A. A. Math and S. N. Vernekar, Markers of renal function tests, N. Am. J. Med. Sci., 2010, 2, 170 Search PubMed.
- A. Kamal, Estimation of blood urea (BUN) and serum creatinine level in patients of renal disorder, Indian J. Fundam. Appl. Life Sci., 2014, 4, 199–202 Search PubMed.
- L. Zhang, H. Zhang, Y. Miao, S. Wu, H. Ye and Y. Yuan, Protective effect of allicin against acrylamide-induced hepatocyte damage in vitro and in vivo, Food Chem. Toxicol., 2012, 50, 3306–3312 CrossRef CAS PubMed.
- Z. Erdemli, M. E. Erdemli, Y. Turkoz, B. Yigitcan, M. A. Aladag, Y. Cigremis, R. H. Cirik, E. Altinoz and H. G. Bag, Vitamin E effects on developmental disorders in fetuses and cognitive dysfunction in adults following acrylamide treatment during pregnancy, Biotech. Histochem., 2020 DOI:10.1080/10520295.2020.1751880.
- R. Zhu, Y. Wang, L. Zhang and Q. Guo, Oxidative stress and liver disease, Hepatol. Res., 2012, 42, 741–749 CrossRef CAS PubMed.
- M. Zhao, P. Wang, Y. Zhu, X. Liu, X. Hu and F. Chen, The chemoprotection of a blueberry anthocyanin extract against the acrylamide-induced
oxidative stress in mitochondria: unequivocal evidence in mice liver, Food Funct., 2015, 6, 3006–3012 RSC.
- V. Gangarapu, S. Gujjala, R. Korivi and I. Pala, Combined effect of curcumin and vitamin E against CCl4 induced liver injury in rats, Am. J. Life Sci., 2013, 1, 117–124 CrossRef CAS.
- S. Ince, D. Arslan-Acaroz, H. H. Demirel, N. Varol, H. A. Ozyurek, F. Zemheri and I. Kucukkurt, Taurine alleviates malathion induced lipid peroxidation, oxidative stress, and proinflammatory cytokine gene expressions in rats, Biomed. Pharmacother., 2017, 96, 263–268 CrossRef CAS PubMed.
- E. Oveland, T. V. Karlsen, H. Haslene-Hox, E. Semaeva, B. Janaczyk, O. Tenstad and H. Wiig, Proteomic evaluation of inflammatory proteins in rat spleen interstitial fluid and lymph during LPS-induced systemic inflammation reveals increased levels of ADAMST1, J. Proteome Res., 2012, 11, 5338–5349 CrossRef CAS PubMed.
- J. M. Huston, M. Ochani, M. Rosas-Ballina, H. Liao, K. Ochani, V. A. Pavlov, M. Gallowitsch-Puerta, M. Ashok, C. J. Czura and B. Foxwell, Splenectomy inactivates the cholinergic antiinflammatory pathway during lethal endotoxemia and polymicrobial sepsis, J. Exp. Med., 2006, 203, 1623–1628 CrossRef CAS PubMed.
- Y. Abe, I. N. Hines, G. Zibari, K. Pavlick, L. Gray, Y. Kitagawa and M. B. Grisham, Mouse model of liver ischemia and reperfusion injury: method for studying reactive oxygen and nitrogen metabolites in vivo, Free Radicals Biol. Med., 2009, 46, 1–7 CrossRef CAS PubMed.
- S. M. Hamdy, A. M. Shabaan, A. K. M. A. Latif, A. M. Abdel-Aziz and A. M. Amin, Protective effect of Hesperidin and Tiger nut against Acrylamide toxicity in female rats, Exp. Toxicol. Pathol., 2017, 69(8), 580–588 CrossRef CAS.
- K. C. Kim, K. A. Kang, R. Zhang, M. J. Piao, G. Y. Kim, M. Y. Kang, S. J. Lee, N. H. Lee, Y.-J. Surh and J. W. Hyun, Up-regulation of Nrf2-mediated heme oxygenase-1 expression by eckol, a phlorotannin compound, through activation of Erk and PI3 K/Akt, Int. J. Biochem. Cell Biol., 2010, 42, 297–305 CrossRef CAS PubMed.
- J. Elavarasan, P. Velusamy, T. Ganesan, S. K. Ramakrishnan, D. Rajasekaran and K. Periandavan, Hesperidin-mediated expression of Nrf2 and upregulation of antioxidant status in senescent rat heart, J. Pharm. Pharmacol., 2012, 64, 1472–1482 CrossRef CAS PubMed.
- M. Chen, H. Gu, Y. Ye, B. Lin, L. Sun, W. Deng, J. Zhang and J. Liu, Protective effects of hesperidin against oxidative stress of tert-butyl hydroperoxide in human hepatocytes, Food Chem. Toxicol., 2010, 48, 2980–2987 CrossRef CAS PubMed.
- Q. Yu, S. P. Nie, J.-Q. Wang, P.-F. Yin, D.-F. Huang, W.-J. Li and M.-Y. Xie, Toll-like receptor 4-mediated ROS signaling pathway involved in Ganoderma atrum polysaccharide-induced tumor necrosis factor-α secretion during macrophage activation, Food Chem. Toxicol., 2014, 66, 14–22 CrossRef CAS PubMed.
- L. C. Tong, Y. Wang, Z.-B. Wang, W.-Y. Liu, S. Sun, L. Li, D.-F. Su and L.-C. Zhang, Propionate ameliorates dextran sodium sulfate-induced colitis by improving intestinal barrier function and reducing inflammation and oxidative stress, Front Pharmacol, 2016, 7, 253 Search PubMed.
- A. M. White and C. S. Johnston, Vinegar ingestion at bedtime moderates waking glucose concentrations in adults with well-controlled type 2 diabetes, Diabetes Care, 2007, 30, 2814–2815 CrossRef CAS PubMed.
- C. J. Kelly, L. Zheng, E. L. Campbell, B. Saeedi, C. C. Scholz, A. J. Bayless, K. E. Wilson, L. E. Glover, D. J. Kominsky and A. Magnuson, Crosstalk between microbiota-derived short-chain fatty acids and intestinal epithelial HIF augments tissue barrier function, Cell Host Microbe, 2015, 17, 662–671 CrossRef CAS PubMed.
|
This journal is © The Royal Society of Chemistry 2021 |