DOI:
10.1039/D0FO01788D
(Paper)
Food Funct., 2021,
12, 351-361
Effect of the formulation and structure of monoglyceride-based gels on the viability of probiotic Lactobacillus rhamnosus upon in vitro digestion†
Received
8th July 2020
, Accepted 8th November 2020
First published on 16th December 2020
Abstract
This research was conducted to evaluate the potential use of saturated monoglyceride (MG)-based gels in the protection of probiotics upon in vitro digestion. For this purpose, a Lactobacillus rhamnosus strain was inoculated into binary and ternary systems, containing MGs, a water phase composed of an aqueous solution at controlled pH or UHT skimmed milk, and in ternary gels, sunflower oil. Gel structure characterization was initially performed just after preparation and after 14 days of storage at 4 °C by rheological, mechanical, thermal, and microscopy analyses. Afterwards, probiotic viability upon in vitro digestion was evaluated. The results highlighted that all freshly prepared samples showed good capability to protect L. rhamnosus with the exception of the binary system containing milk. However, the digestion of samples after 14 days of storage showed that the ternary system containing skimmed milk exhibited the best protection performance ensuring a L. rhamnosus viability of almost 106 CFU g−1 at the end of the gastrointestinal passage. Confocal microscopy results demonstrated that bacterial cells were located prevalently within the aqueous domain near the monoglycerides and protein aggregates. Under these conditions, they can simultaneously achieve physical protection and find nutrients to survive environmental stresses. These findings suggest that MG-based gels can be proposed as efficient carriers of probiotic bacteria not only during food processing and storage but also upon digestion.
1 Introduction
In the complex framework of functional foods, products enriched with probiotic bacteria have received particular attention due to the well-recognized benefits of the consumption of adequate amounts of these microorganisms.1–3 According to recent statistics, from 2015 to 2025, the global probiotic supplement market is expected to increase from 3.3 to 7 US$ billion.2 However, to support this market growth, several challenges should be addressed to ensure the delivery of probiotic bacteria in adequate amounts to the target host site: today, it is well recognized that a viability higher than 106–107 UFC g−1 is needed to guarantee the claimed health benefits.4 It is a matter of fact that during food processing and storage, microbial cells encounter a number of different stresses (e.g. temperature, mechanical forces, oxygen, and pH) critically affecting their viability.2,5,6 Moreover, during the gastrointestinal transit, the strongly acidic conditions of the stomach, as well as the presence of enzymes and bile salts, are expected to significantly reduce their survival.7–9 To lessen the detrimental effects of these stressful conditions, a plethora of protection strategies based on food biopolymer structuring capacity has been proposed.6,10–12 All of them could find application in the complex world of foods because the most appropriate delivery strategy should be designed and tailored on the basis of the target food characteristics.
Recently, our research group demonstrated the capacity of monoglyceride (MG)-based structured emulsions (MSEs) to protect a probiotic L. rhamnosus strain during processing and storage.13,14 In particular, Marino et al.13 observed that MSEs containing milk as the water phase were able to keep L. rhamnosus viable for up to 56 days at 4 °C. The same system once introduced in ice creams showed a good capability of preserving probiotic viability during processing and storage without significantly impacting their sensory and quality attributes.14 The protection capacity of MSEs was attributed to the presence of a crystalline lamellar structure of saturated monoglycerides. It has been speculated that probiotic cells place themselves prevalently in the aqueous phase near the monoglyceride crystalline bilayers, where they can find protection from processing and environmental stresses. However, the roles of different system phases and their composition have not been fully elucidated.
Despite the interesting potentialities of MSEs described above their exploitation as a probiotic delivery system can be claimed only if they would be able to protect the bacteria not only during processing and storage but also during digestion. It is well known that the microbial tolerance against digestion-related stresses is strongly dependent not only on the microbial strain but also on the structure and physicochemical characteristics of the delivery system as well as the food containing them.2,6,9,11,15–18 In the last few decades, many in vitro digestion protocols have been developed to simulate the gastrointestinal fate of food components.19–24 The application of these protocols could allow selecting the best performing strategy among different delivery strategies before in vivo validation. However, they have rarely been applied to study the viability of probiotic bacteria introduced in differently structured systems.9,18,25–27
Starting from our previous study, in the present work, the aim was to understand the role of the composition and resulting structure of monoglyceride-based gels in the survival of a probiotic L. rhamnosus strain during storage at 4 °C as well as upon in vitro digestion. For this purpose, binary (hydrogel) and ternary (emulsion-gel) systems containing MGs as structuring agents, i.e., a water phase composed of an aqueous solution at controlled pH or UHT skimmed milk, and ternary gels, such as sunflower oil were considered. In this way the effects of the water phase composition (aqueous phase vs. milk) and the presence/absence of a lipid phase (hydrogel vs. emulsion gel) on both the system structure and microbial protection capacity were studied. It should be considered that the presence of surface-active milk components, especially proteins, could deeply modify the MG structuring behavior in both hydrogels and emulsion gels.28–31
After sample structural characterization (optical, polarized light and confocal microscopy, differential scanning calorimetry, mechanical and rheological analyses, and water holding capacity analysis), the probiotic protection capacity of MG-structured systems was studied during storage at 4 °C and after oral, gastric and intestinal phases. The particle size, zeta-potential and swelling capacity of the digesta were also determined to study the destructuring behavior of the gels.
2 Materials and Methods
2.1 Materials
α-Amylase from Bacillus sp. (EC 3.2.1.1), porcine pepsin (EC 3.4.23.1), porcine pancreatin (EC 232-468-9, 8×USP), porcine bile extract, NaH2PO4(2H2O), NaOH, CaCl2(H2O)2, Na2CO3, NaHCO3, NaCl, KCl, KH2PO4, MgCl2(H2O)6, (NH4)2CO3, PBS, Fast Green FCF, Nile Red, stearic acid and palmitic acid were purchased from Sigma Aldrich (Milano, Italy); HCl and NaOH were provided by JT Baker (Center Valley, USA); Hoechst was purchased from Thermo Fisher Scientific Inc. (Massachusetts, USA); and saturated monoglycerides were purchased from Kerry Ingredients and Flavour, Bristol, United Kingdom (fatty acid composition: 1.4% C14:0, 59.8% C16:0, and 38.8% C18:0; melting point: 68.05 ± 0.5 °C). Maximum Recovery Diluent (MRD), MRS agar, and MRS broth were purchased from Oxoid (Milan, Italy). L. rhamnosus (Lyofast LRB) was purchased from Sacco Srl (Cadorago, Como, Italy). Sunflower oil and UHT skimmed milk (pH = 6.70) were purchased from a local market. Deionized water (System advantage A10®, Millipore S.A.S, Molsheim, France) was used.
2.2 Culture preparation
L. rhamnosus was stored at −80 °C as 30% (v
:
v) glycerol stock-culture in MRS broth. Before each experiment, overnight cultures were prepared by sub-culturing 100 μL of stock-cultures in 100 mL of MRS broth at 37 °C for 18 h under anaerobic conditions. The cells were then recovered by centrifugation at 13 000g for 10 min at 4 °C, washed three times with PBS and resuspended in PBS to a final viability of about 109 CFU mL−1.
2.3 Monoglyceride-based system preparation
Two binary (B-) and two ternary (T-) systems were considered. All systems contained a 7.2% (w
:
w) cosurfactant–monoglyceride (CO–MG) mixture made of MG mixed with palmitic and stearic acid in a ratio of 5
:
1
:
1 (w
:
w). The composition of the systems is reported in Table 1. In the binary systems the CO–MG mixture was added to UHT skimmed milk or an aqueous solution (Milli-Q water) at pH = 10.9 adjusted with 1 mM NaOH. The ternary systems were composed of CO–MG, a water phase (UHT skimmed milk or the aqueous solution at pH 10.9) and sunflower oil. Based on the water phase composition, the binary samples were named B-water and B-milk and the ternary ones T-water and T-milk.
Table 1 Composition of binary (B-water and B-milk) and ternary (T-water and T-milk) systems
System |
Composition (% w/w) |
UHT skimmed milk |
Water |
Oil |
Monoglycerides |
Palmitic acid |
Stearic acid |
B-water |
— |
92.80 |
— |
5.14 |
1.03 |
1.03 |
B-milk |
92.80 |
— |
— |
5.14 |
1.03 |
1.03 |
T-water |
— |
56.40 |
36.40 |
5.14 |
1.03 |
1.03 |
T-milk |
56.40 |
— |
36.40 |
5.14 |
1.03 |
1.03 |
The systems were then prepared following a previously reported methodology.13 Briefly, the water phase (aqueous solution or skimmed milk) and the lipid phase containing the CO–MG mixture with (T-) or without sunflower oil (B-) were heated at 70 °C in a water bath. After complete melting of CO–MG, the two phases were mixed and, immediately after, 1 mL of L. rhamnosus suspension (about 109 CFU mL−1) was added to the resulting mixtures. The samples were then homogenized using a high-speed homogenizer at 1000g for 20 s. Subsequently, the samples were cooled in an ice bath and placed in sterile 120 mL airtight containers. All systems were prepared in two biological replicates. Analyses were performed after 1 and 14 days of storage at 4 °C.
2.4 Analytical determination
2.4.1 Image acquisition.
Images were captured using an acquisition cabinet (Immagini & Computer, Bareggio, Italy) equipped with a digital camera (EOS 550D, Canon, Milano, Italy). The samples were placed on a black background and a digital camera was placed on an adjustable stand positioned at 45 cm in front of the samples. Light was provided by 4100 W frosted photographic floodlights. Images were saved in the jpeg format, resulting in 5184 × 3456 pixels.
2.4.2 Polarized light microscopy.
Samples were analyzed at room temperature using an optical microscope (Leica DM 2000, Leica Microsystems, Heerbrugg, Switzerland) with and without polarized light connected with a Leica EC3 digital camera (Leica Microsystems). One drop of the sample was placed in the middle of a glass slide and a glass coverslip was centered above the drop. The samples were analyzed at 20 °C using 200× magnification. Images were captured using the Leica Suite LAS EZ software (Leica Microsystems, Heerbrugg, Switzerland). Images were saved in the jpeg format resulting in 2048 × 1536 pixels.
2.4.3 pH measurement.
The pH was measured using a standard pH-meter (Hanna Instruments pH 301, Padua, Italy). Calibration was performed using three different buffer solutions at pH 4, 7 and 9. All measurements were performed in duplicate at 25 °C.
2.4.4 Rheology.
The samples were characterized at 20 °C using an RS6000 Rheometer (Thermo Scientific, HAAKE RheoStress, Germany) equipped with a Peltier cell. A parallel plate geometry was used, and the measuring gap was set at 2 mm. To determine the linear viscoelastic region for each sample, stress sweep tests were performed by increasing the stress from 0.1 to 100 Pa at 1 Hz frequency. Frequency sweep tests were performed by increasing the frequency from 0.1 to 10 Hz using a fixed stress value included in the linear viscoelastic region. All measurements were conducted in triplicate on all samples at the beginning (1 day) and at the end of storage (14 days) at 4 °C.
2.4.5 Textural properties.
The samples were placed in plastic containers (4 cm internal diameter × 3 cm height) and equilibrated for 1 day at 20 °C. Textural properties were measured according to the procedure given by Chen et al. with some modifications.30 Penetration tests were performed using a TA.XT Plus Unit Texture Analyzer (Stable Micro Systems Ltd, Godalming, UK) with a cylindrical probe (diameter: 8 mm) and a 5 kg compression head at a speed of 60 mm min−1 to a total penetration distance of 15 mm. All measurements were performed on all samples at the beginning (1 day) and at the end of storage (14 days) at 4 °C. The results are expressed as the mean of at least 6 repetitions on 2 replicates.
2.4.6 Water holding capacity (WHC).
An aliquot of 1 g of sample was placed in a 2 mL Eppendorf tube and centrifuged at 10 000g at 20 °C for 30 min. The supernatant was eliminated, and the pellet obtained was weighed. The water holding capacity was calculated using eqn (1). | 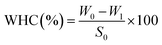 | (1) |
where W0 and W1 are the weight (g) of water in the samples before and after centrifugation, respectively. All measurements were conducted on all samples at the beginning (1 day) and at the end of storage (14 days) at 4 °C. The results are expressed as the mean of at least 3 repetitions on 2 replicates.
2.4.7 Differential scanning calorimetry.
DSC analysis was carried out using a DSC 3 STARe System differential scanning calorimeter (Mettler-Toledo, Greifensee, Switzerland). Heat flow calibration was achieved using indium (heat of fusion: 28.45 J g−1). Temperature calibration was carried out using hexane, water and indium (with melting points of −93.5 °C, 0.0 °C and 156.6 °C, respectively). Samples were prepared by carefully weighing 5–10 mg in 40 μL aluminum DSC pans, closed by hermetic sealing. The samples were heated under a nitrogen flow (20 mL min−1) during analysis. An empty pan was used as a reference in the DSC cell. The start and end of the melting transition were taken as on-set (Ton) and off-set (Toff) points of transition, and these are the points at which the extrapolated baseline intersects the extrapolated tangent of the calorimetric peak in the transition state. Total peak enthalpy was obtained by the integration of the melting curve. The machine equipment program STARe ver. 16.10 (Mettler-Toledo, Greifensee, Switzerland) was used to plot and analyze the thermal data. All measurements were conducted in duplicate.
2.4.8 Confocal laser scanning microscopy.
0.2% (w/w) aqueous solutions of Nile Red and Fast Green FCF were used to stain the lipid and protein phases of the MG-based systems, respectively. Probiotic cells were stained using 0.02% (v/v) Hoechst. After staining, the samples were dropped on a microscope slide, covered with a coverslip and analyzed on a Leica TCS SP8 confocal system (Leica Microsystems, Wetzlar, Germany) equipped with a 100×/1.4 oil immersion objective, a 405 nm diode laser (Hoechst excitation) and a tunable white light laser set to 535 and 633 nm (Nile Red and Fast Green FCF excitation, respectively). Images were recorded as a z-stack series in sequential scanning mode and are reported as maximum intensity projections.
2.5 Evaluation of the probiotic viability during storage
Aliquots of 1 g of each gel were suspended in 9 mL of MRD and homogenized for 2 min. Decimal dilutions in MRD were then spread plated on MRS agar and incubated anaerobically at 37 °C for 48 h. Viable counts in the systems were compared to those of control samples made of the water phases (aqueous solution or skimmed milk) of each binary or ternary MG-gel inoculated with L. rhamnosus (final viable count: about 108 CFU mL−1) and stored at 4 °C.
2.6
In vitro digestion
In vitro digestion was carried out on samples at the beginning (1 day) and at the end of storage (14 days) at 4 °C in accordance with the INFOGEST protocol.32 The simulated salivary (SSF), gastric (SGF) and intestinal (SIF) fluids were prepared and warmed at 37 °C. 2.5 g of sample and 2.5 mL of relative control were weighed in 50 mL Falcon tubes. The oral phase was obtained by adding 13 μL of 0.3 M CaCl2 (H2O)2, 488 μL of water and 2 mL of 6.55 mg mL−1 α-amylase solution in SSF (activity: 75 U mL−1 in the final mixture). The entire mixture was maintained at 37 °C under stirring for 2 min. Subsequently, 3 μL of 0.3 M CaCl2 (H2O)2, 347 μL of water and 4.55 mL of a 0.07 mg mL−1 pepsin solution in SGF (2000 U mL−1 in the final mixture) were added. The pH was adjusted to 3 by adding 100 μL of 6 M HCl to start the gastric phase. The chyme was maintained under stirring at 37 °C for 2 h. Finally, the gastric chyme was mixed with 20 μL of 0.3 M CaCl2 (H2O)2, 655 μL of water, 1.25 mL of 160 mM bile extract in SIF and 8 mL of 22.15 mg mL−1 pancreatin solution in SIF (100 U mL−1 in the final mixture). The pH was adjusted to 7 by adding 75 μL of 1 M NaOH and the mixture was stirred at 37 °C for 2 h. At the end of each phase, the sample was collected and put in an ice bath to stop the enzymatic reaction. L. rhamnosus viability was evaluated before digestion and at the end of each phase, as previously reported (paragraph 2.5), and compared to control samples.
2.6.1 Swelling properties.
The swelling properties during the gastric phase were measured by using the same procedure described in paragraph 2.6 but using SGF without pepsin.33 After 2 h incubation, the samples were dried to remove excess liquid and then weighed. The swelling ratio was calculated using eqn (2). | 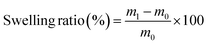 | (2) |
where m0 and m1 represent the weight of the gel before and after the gastric phase, respectively.
2.6.2 Particle size and zeta potential of digested samples.
The particle size distribution of the mixed micellar phase of digested gels and control (UHT skimmed milk) was measured by dynamic laser light scattering (Zetasizer Nano ZS, Malvern Instruments, Worcestershire, UK). The samples were diluted 1
:
100 (v/v) with deionized water and placed in a cuvette where the laser light, set at a 173° angle, was scattered by the particles. The particle size was reported as the volume-weighed mean diameter in nm. The ζ-potential was also measured by placing the diluted sample in a capillary cell equipped with two electrodes to assess particle electrophoretic mobility.
2.7 Statistical analysis
All results were expressed as the mean ± standard deviation (SD) of at least two measurements from two experimental replications. t-Test and analysis of variance (ANOVA) were performed using R v. 3.1.1 for Windows (the R foundation for statistical computing). Tukey's post-hoc test was used to assess differences between means (p < 0.05).
3 Results and discussion
3.1 Characterization of MG-based structured systems
Table 2 shows the visual appearance and micrographs under normal and polarized light of the four MG-based systems considered in this study. As expected and in agreement with the literature, all the samples presented a white color and a self-standing behavior34,35 with the pH in the range of 5.19–5.57 and a very high WHC (Table 3). In both binary and ternary systems, the self-standing behavior is due to the formation of a network of MG crystalline lamellae.35,36 In binary systems, double layers of MG were separated by water layers and the swelling capacity of the aqueous lamellar phase of non-ionic monoglycerides was enhanced by the introduction of charged groups on the surface of the lipid bilayers (in this case stearic and palmitic acids), increasing in this way the repulsive forces and thus the swelling of MG structures in water.37–39 In the ternary system, the MG crystalline lamellae surrounded the oil droplets building up the network,36 as is well evident in the micrographs (Table 2). It should be noted that bigger oil droplets were observed in the T-milk sample in comparison with T-water.
Table 2 Visual appearance, micrographs under normal and polarized light, and pH of binary (B-water and B-milk) and ternary (T-water and T-milk) systems
System |
Visual appearance |
Micrograph under normal light |
Micrograph under polarized light |
pH |
B-water |
|
|
|
5.49 ± 0.01 |
B-milk |
|
|
|
5.51 ± 0.01 |
T-water |
|
|
|
5.19 ± 0.01 |
T-milk |
|
|
|
5.57 ± 0.02 |
Table 3 Storage modulus (G′), loss modulus (G′′), hardness, and water holding capacity (WHC) of binary (B-water and B-milk) and ternary (T-water and T-milk) systems, after 1 and 14 days of storage at 4 °C
System |
Storage time (days) |
1 |
14 |
G′ (Pa) |
G′′ (Pa) |
Hardness (N) |
WHC (%) |
G′ (Pa) |
G′′ (Pa) |
Hardness (N) |
WHC (%) |
Lowercase letters (a–d) indicate significant differences among gels with the same storage time (p < 0.05). Uppercase letters (A–B) indicate significant differences between each gel just after preparation and after 14 days of storage (p < 0.05). |
B-water |
972 ± 23c,A |
309 ± 38c,A |
0.233 ± 0.012c,A |
98.9 ± 0.7a,A |
1048 ± 39c,A |
427 ± 71b,A |
0.233 ± 0.025c,A |
98.8 ± 0.2a,A |
B-milk |
652 ± 44d |
288 ± 12c |
0.161 ± 0.004d |
99.2 ± 0.2a |
— |
— |
— |
— |
T-water |
10 833 ± 933a,A |
4708 ± 543a,A |
0.914 ± 0.090a,A |
99.1 ± 0.6a,A |
10 247 ± 767a,A |
4132 ± 59a,A |
0.899 ± 0.031a,A |
99.1 ± 0.5a,A |
T-milk |
2565 ± 166b,A |
1205 ± 14b,A |
0.385 ± 0.015b,A |
99.1 ± 0.7a,A |
2025 ± 146b,B |
980 ± 77b,B |
0.387 ± 0.014b,A |
99.0 ± 0.2a,A |
To better characterize the samples, rheological analyses were performed (Table 3 and Fig. S1†). Even though all samples presented a weak-gel like behavior with the storage modulus (G′) higher than the viscous modulus (G′′) in the entire frequency range considered, the magnitude of both moduli was affected by the formulation. The ternary systems resulted in stronger gels in comparison with binary samples, indicating that the inclusion of oil reinforced the system structure. In contrast and in agreement with previous observations,13,28 the presence of milk components disturbed the MG structural organization, reducing the strength of the gel network. These results were also confirmed by the sample hardness values (Table 3): milk containing systems showed lower hardness values in comparison with the relevant water-based samples. All these results clearly showed the critical role of milk in determining the gel structure. This result is in agreement with that of Valoppi et al. (2015), reporting that the presence of milk native components, mainly proteins and salts, could interfere with the MG structural organization by changing their displacement at the water–oil interface and modifying the swelling MG behavior affecting the electrical charge in the medium.28 Moreover, the modification of the gel network characteristics could be further induced by protein–MG interactions,30,40 leading to a reduction of the availability of MG for network building. This is also true in binary samples, in which the presence of milk led to a less dense structure. This hypothesis is also corroborated by the calorimetric results showing a Ton decrease of the melting peak occurring at temperatures around 55–60 °C in systems containing milk (Table 4).
Table 4 Enthalpy (ΔH) and T onset (Ton) of binary (B-water and B-milk) and ternary (T-water and T-milk) systems, after 1 and 14 days of storage at 4 °C
System |
Storage time (days) |
1 |
14 |
ΔH (J g−1) |
T
on (°C) |
ΔH (J g−1) |
T
on (°C) |
Lowercase letters (a–c) indicate significant differences among gels with the same storage time (p < 0.05). Uppercase letters (A–B) indicate significant differences between each gel just after preparation and after 14 days of storage (p < 0.05). |
B-water |
6.43 ± 0.65a,A |
58.09 ± 0.38a,A |
6.12 ± 0.59a,A |
57.32 ± 0.88a,A |
B-milk |
4.92 ± 0.39b |
56.02 ± 0.54b |
|
|
T-water |
3.22 ± 0.30c,A |
59.19 ± 0.18b,A |
3.64 ± 0.11b,A |
55.49 ± 0.11a,A |
T-milk |
4.73 ± 0.18b,A |
55.09 ± 0.32b,A |
4.86 ± 0.02ab,A |
55.34 ± 0.37a,A |
It is interesting to note that no significant differences were detected in rheological parameters, thermal properties, hardness values and textural properties after 14 days of storage at 4 °C in samples containing water (Tables 3 and 4). In the case of those containing milk, the B-milk sample presented an evident syneresis after storage, and thus it was not considered for this and further analyses. Considering the T-milk samples, a slight reduction of the rheological parameters after 14 days of storage was recorded, evidencing a possible rearrangement of the gel network structure upon storage. These changes appeared limited and did not affect the water holding capacity (WHC) of the system (Table 3). Finally, to highlight the location of bacteria as well as the protein and MG organization, confocal microscopy was performed on the T-milk system, which was the most complex sample from a compositional point of view (Fig. 1). In Fig. 1a, the presence of oil droplets (biggest green droplets) and the MG network (small green particles) connecting them is well evident. These lipid components were dispersed in a water phase containing milk proteins (red signals), as shown in Fig. 1b. By adding an additional dye to the system, bacteria (cyan signals) were also imaged. To the best of our knowledge, live microbial cells were rarely observed in emulsified systems.41 From the images it can be noted that Lactobacillus cells were located in the aqueous domain prevalently near the MG structures (Fig. 1a) as well as protein aggregates (Fig. 1b).
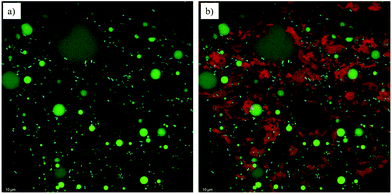 |
| Fig. 1 Confocal laser scanning micrographs of the L. rhamnosus-containing T-milk system stained with Nile Red and Fast Green FCF to visualize the lipid and protein phases, and with Hoechst to detect bacterial cells. Overlay of Nile Red and Hoechst channels only, to allow clearer visualization of bacterial localization (a) and the merged maximum intensity z-projection of all channels (b) are reported. (Green, Nile Red; red, Fast Green FCF; and cyan, Hoechst.) | |
3.2 MG-based system properties during digestion
The gels were exposed to gastrointestinal conditions to study the destructuring behavior of binary and ternary systems. To this aim, the swelling properties under gastric conditions were measured. This analysis could give insight into the destructuring phenomena occurring during digestion, giving reasons for the capacity of the system to re-absorb water and the results depend on the capacity of the network to allow water diffusion.33 The samples containing water exhibited a swelling ratio (%) of 12.95 ± 0.20 and 18.91 ± 0.05 for B- and T-water samples, respectively. On the other hand, the swelling properties of milk containing samples were impossible to measure probably due to the sensitiveness of milk proteins to environmental stresses, such as acid pH and ionic strength, which could cause protein unfolding and conformational changes.29,33 It should be noted that no significant changes in swelling properties were observed in stored samples.
To better understand the gel behavior upon digestion, the particle size distributions were determined at the end of the intestinal phase (Fig. 2). After 1-d storage (Fig. 2a), all the systems showed a main peak with an average diameter ranging from 300 to 450 nm. Based on the literature, this peak can be attributed to the presence of mixed micelles formed upon digestion, as well as for the sample containing milk, to the presence of milk proteins.42,43 In fact, the same experiment performed on a digested milk control sample showed the presence of a similar peak with a mean diameter of around 250 nm. In MG-containing samples, it is expected that MG participated in the stabilization of mixed micelles as surface active molecules.44,45 When oil was included in the system (ternary samples), the presence of smaller micelles was noted. This result can be attributed to the additional presence of digested lipids generated as a consequence of lipid digestion in the intestinal phase (e.g. mono- and di-glycerides and fatty acids).42,46 These observations were in agreement with those of Alongi et al. (2019)47 and Salvia-Trujillo et al. (2013)48 who described the decrease in droplet size with the increase of the lipid content in the digested sample. Comparing ternary systems, a narrower particle size distribution of the milk containing samples can be noted in comparison with the water-based gel. This result can be attributed to the presence of milk proteins in the digesta which, being surface active, could have an emulsion stabilizing effect locating at micelle interfaces or forming protein–lipid complexes.40 Finally, a peak with an average diameter of 4500–5000 nm can be observed in the particle size distributions. This can be attributed to bile salts, free fatty acids, undigested lipids, and digested milk proteins.43,44,47,48
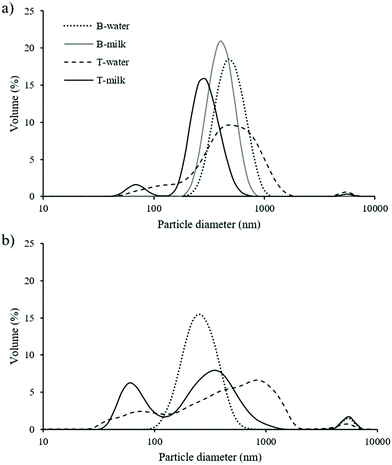 |
| Fig. 2 Particle size distribution of digested MG-based systems after 1 (a) and 14 (b) days of storage. | |
After 14 d of storage (Fig. 2b), broader distributions were observed in all samples, even if the average particle size remains almost unchanged. Interestingly, the volume of the smaller particle family (70 nm) in T-milk was higher than that observed just after 1 d of storage. This could be attributed to higher lipolysis due to the reduced gel network strength (Table 3).48
The digested samples were further analysed for their ζ-potential (Fig. 3). This parameter is correlated with the surface electrical charge of particles and provides information about the digesta stability.42 It should be noted that UHT skimmed milk digestion led to a ζ-potential of −27.69 ± 0.67 mV. The presence of MG, as expected, decreased this parameter.31 The additional presence of oil caused a further decrease of the particle charge, probably due to the increased number of surface-active molecules, mainly free fatty acids, derived from lipid digestion, as previously stated by other authors.42,46 After 14 d of storage, the same trend was observed.
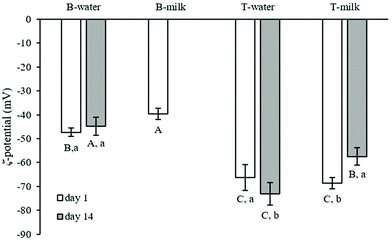 |
| Fig. 3
ζ-Potential of digested MG-based systems after 1 and 14 days of storage. a and b indicate a significant difference (p < 0.05) after 1 and 14 days of storage within the same sample. A–C indicate a significant difference (p < 0.05) between MG-based systems at the same storage time. | |
3.3
In vitro digestion of MG-based structured systems
In the last part of the research, the viability of L. rhamnosus included in the four considered MG-based gels or digested as free-cells in the relevant water domains was evaluated throughout in vitro digestion (Fig. 4). After the oral phase, only a slight viability reduction was observed in all samples, probably because this phase is characterized by a short exposure (2 min) at neutral pH without any antimicrobial substance. Instead, the samples behaved differently after the gastric and intestinal phases. After the gastric phase, the control samples showed a viability reduction of about 3.77 ± 0.27 and 3.49 ± 0.16 log CFU mL−1, respectively, and no further viability reduction was observed after the intestinal phase. These results confirmed that the gastric conditions are particularly stressful for microorganisms.20,26,49,50 In fact, the acidic environment of the stomach could be responsible for the damage of the cell membrane, DNA and cellular proteins leading to the loss of bacterial viability.51,52
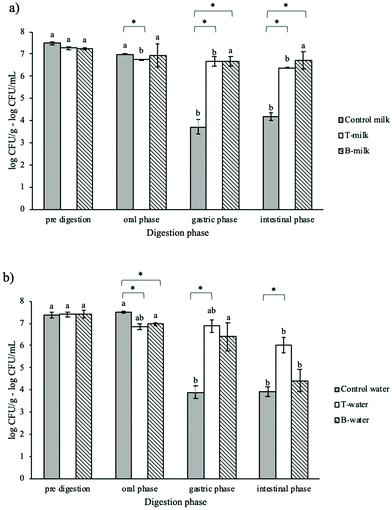 |
| Fig. 4 Viability of L. rhamnosus incorporated into systems containing milk (a) and water (b), and relative controls stored for 1 day at 4 °C before digestion and at the end of the oral, gastric and intestinal phases of in vitro digestion. * indicates a significant difference (p < 0.05) between the sample and relative control within the same digestion phase. a and b indicate a significant difference (p < 0.05) among different digestion phases within the same sample. | |
Different results were obtained upon digestion of MG-gels. All the samples containing milk (B-milk and T-milk) as well as the T-water system showed a good protective capacity with only ∼0.5 log reduction of the microbial count at the end of the gastric phase (Fig. 4a and b). No further reduction was noted after the intestinal phase, except for the B-water sample.
These results highlighted the critical role of the presence of crystalline monoglycerides during digestion in protecting bacteria. Comparing water containing systems after the intestinal phase, a reduced protective capacity of B-water was observed. This result could be attributed to the impact of the system structure and to its destructuring behavior during digestion. In fact, as reported in Table 3, the B-water gel was weaker than T-water and, upon digestion, the presence of oil induced the formation of micelles with a lower negative charge and a broader particle size distribution (Fig. 2 and 3). In this situation, the microbes were more protected.
Regarding the B- and T-milk gels, they showed similar results upon digestion (Fig. 4a). It can be hypothesized that the presence of milk proteins, undergoing unfolding, cross-linking and aggregation during the gastric phase,53 could further protect the bacteria.
Considering that a probiotic food can be stored before consumption, the L. rhamnosus viability during in vitro digestion was evaluated by submitting trial samples stored for 14 days at 4 °C to digestion (Fig. 5). In these experiments, we excluded the sample B-milk due to the evident syneresis, as described in paragraph 3.1. It should be firstly highlighted that all structured and unstructured samples showed no significant changes (p > 0.05) in the viable counts during the storage at 4 °C. Although this is a much lower temperature than the optimal growth temperature of L. rhamnosus, the pH of the medium and the duration of the storage at 4 °C did not cause any loss of vitality. After the oral phase of digestion, no viability reduction was detected, in agreement with the data discussed above. Further gastric phase conditions led to a 2–3 log reduction of the cell viability of unstructured controls as well as T- and B-water samples (Fig. 5a and b). Instead, the protective capacity of the T-milk gel was confirmed, as demonstrated by the slightly reduced viability of about 0.5 log after the gastric phase and intestinal phase. It could be supposed that the presence of milk constituents in the system during storage at 4 °C contributed to L. rhamnosus survival by helping the microbes remain in a metabolically active state. It can be speculated that bacteria in aged water containing systems presented a reduced capacity to survive the stressing conditions encountered during digestion, which has been shown for different probiotics.54,55 This could be due to the fact that, even if probiotics survive during storage, their acid and bile tolerance could decrease.56 These results are in agreement with those reported by Marino et al.13 who observed a protective effect of a MG-gel containing milk on L. rhamnosus viability for up to 56 days of storage at 4 °C. During digestion, the presence of milk proteins played a key role in protecting bacteria, confirming the above-reported considerations.
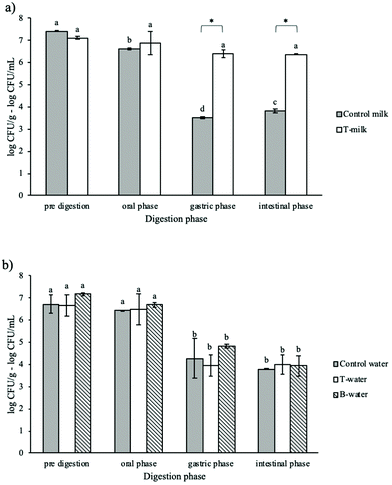 |
| Fig. 5 Viability of L. rhamnosus incorporated into systems containing milk (a) and water (b), and relative controls stored for 14 days at 4 °C before digestion and at the end of the oral, gastric and intestinal phases of in vitro digestion. * indicates a significant difference (p < 0.05) between the sample and relative control within the same digestion phase. a and b indicate a significant difference (p < 0.05) among different digestion phases within the same sample. | |
Summarizing these results, the capacity of the MG structure to confer protection to probiotic bacteria was confirmed also during digestion. The highest protective ability of MG-gels was observed for samples containing milk as the water phase, highlighting the critical role of milk components in microbial survival. As expected, the most critical phase for microbial survival was the gastric one (Fig. 4 and 5). The presence of crystalline MG structures provides protection to bacteria probably because they could find physical protection by locating themselves near lipid structures (Fig. 1). Under gastric conditions, MG crystal structures could still remain in the digesta since they undergo melting only at temperatures higher than 55 °C (Table 4). The additional protective role of milk components, mainly proteins, which are expected to undergo unfolding under gastric conditions, cannot be excluded. After the gastric phase, further system destructuring is expected to occur as this phase is involved in lipid digestion and mixed micelle formation (Fig. 2 and 3).44,45,57 However, intestinal conditions were less stressful for bacteria as demonstrated by the results of the control samples (Fig. 4 and 5). Upon digestion of aged samples, the presence of milk in the system appeared to be critical. It can be inferred that bacteria survived better upon digestion when they are “not stressed” by long storage under nonoptimal conditions. This could impair their ability to counteract the antimicrobial activities of acidic pH and bile salts.
4 Conclusions
The results obtained in this study confirmed the capability of monoglyceride self-assembled structures to protect probiotic bacteria against stresses suffered not only during food processing and storage but also during digestion. To design an efficient MG-based system it is necessary to consider both the formulation and structure. In fact, the best performing gel was the ternary system containing milk, which maintained a good protective capacity even after storage at 4 °C. In this system probiotic bacteria were located in the aqueous domain, where they found at the same time physical protection and nutrients to survive environmental stresses. The presence of milk further improved the protection capacity of the system. These findings suggest that MG-based gels can be effectively proposed as an efficient carrier of probiotic bacteria not only during food processing and storage but also upon digestion. Being emulsion gels, different possible applications in foods can be found, such as in creams and spreads, ice-creams and, also as a more challenging possibility, in bakery products.
Conflicts of interest
There are no conflicts to declare.
Acknowledgements
This work was partially supported by the University of Udine – I, Research Quality Enhancement Plan 2018. Action 3b Competitive research funding.
The authors are also grateful to Mr Federico Poles for contributing to analyses.
References
- C. Hill, F. Guarner, G. Reid, G. R. Gibson, D. J. Merenstein, B. Pot, L. Morelli, R. B. Canani, H. J. Flint, S. Salminen, P. C. Calder and M. E. Sanders, Expert Consensus Document: The International Scientific Association for Probiotics and Prebiotics Consensus Statement on the Scope and Appropriate Use of the Term Probiotic, Nat. Rev. Gastroenterol. Hepatol., 2014, 11, 506–514 CrossRef PubMed.
- A. Terpou, A. Papadaki, I. K. Lappa, V. Kachrimanidou, L. A. Bosnea and N. Kopsahelis, Probiotics in Food Systems: Significance and Emerging Strategies towards Improved Viability and Delivery of Enhanced Beneficial Value, Nutrients, 2019, 11, 1591 CrossRef CAS PubMed.
- T. Huq, A. Khan, R. A. Khan, B. Riedl and M. Lacroix, Encapsulation of Probiotic Bacteria in Biopolymeric System, Crit. Rev. Food Sci. Nutr., 2013, 53, 909–916 CrossRef CAS PubMed.
- A. Talwalkar, C. W. Miller, K. Kailasapathy and M. H. Nguyen, Effect of Packaging Materials and Dissolved Oxygen on the Survival of Probiotic Bacteria in Yoghurt, Int. J. Food Sci. Technol., 2004, 39, 605–611 CrossRef CAS.
- M. Yao, J. Xie, H. Du, D. J. McClements, H. Xiao and L. Li, Progress in Microencapsulation of Probiotics: A Review, Compr. Rev. Food Sci. Food Saf., 2020, 1–18 Search PubMed.
- H. Liu, S. W. Cui, M. Chen, Y. Li, R. Liang, F. Xu and F. Zhong, Protective Approaches and Mechanisms of Microencapsulation to the Survival of Probiotic Bacteria during Processing, Storage and Gastrointestinal Digestion: A Review, Crit. Rev. Food Sci. Nutr., 2019, 59, 2863–2878 CrossRef PubMed.
- R. D. C. S. Ranadheera, S. K. Baines and M. C. Adams, Importance of Food in Probiotic Efficacy, Food Res. Int., 2010, 43, 1–7 CrossRef CAS.
- I. Sumeri, L. Arike, J. Stekolštšikova, R. Uusna, S. Adamberg, K. Adamberg and T. Paalme, Effect of Stress Pretreatment on Survival of Probiotic Bacteria in Gastrointestinal Tract Simulator, Appl. Microbiol. Biotechnol., 2010, 86, 1925–1931 CrossRef CAS PubMed.
- S. Melchior, M. Marino, N. Innocente, S. Calligaris and M. C. Nicoli, Effect of Different Biopolymer-Based Structured Systems on the Survival of Probiotic Strains during Storage and in Vitro Digestion, J. Sci. Food Agric., 2020, 100, 3902–3909 CrossRef CAS PubMed.
- S. B. Doherty, V. L. Gee, R. P. Ross, C. Stanton, G. F. Fitzgerald and A. Brodkorb, Efficacy of Whey Protein Gel Networks as Potential Viability-Enhancing Scaffolds for Cell Immobilization of Lactobacillus Rhamnosus GG, J. Microbiol. Methods, 2010, 80, 231–241 CrossRef CAS PubMed.
- J. Burgain, C. Gaiani, M. Linder and J. Scher, Encapsulation of Probiotic Living Cells: From Laboratory Scale to Industrial Applications, J. Food Eng., 2011, 104, 467–483 CrossRef CAS.
- C. Gebara, K. S. Chaves, M. C. E. Ribeiro, F. N. Souza, C. R. F. Grosso and M. L. Gigante, Viability of Lactobacillus Acidophilus La5, in Pectin-Whey Protein Microparticles during Exposure to Simulated Gastrointestinal Conditions, Food Res. Int., 2013, 51, 872–878 CrossRef CAS.
- M. Marino, N. Innocente, S. Calligaris, M. Maifreni, A. Marangone and M. C. Nicoli, Viability of Probiotic Lactobacillus Rhamnosus in Structured Emulsions Containing Saturated Monoglycerides, J. Funct. Foods, 2017, 35, 51–59 CrossRef CAS.
- S. Calligaris, M. Marino, M. Maifreni and N. Innocente, Potential Application of Monoglyceride Structured Emulsions as Delivery Systems of Probiotic Bacteria in Reduced Saturated Fat Ice Cream, LWT–Food Sci. Technol., 2018, 96, 329–334 CrossRef CAS.
- P. Singh, B. Medronho, T. dos Santos, I. Nunes-Correia, P. Granja, M. G. Miguel and B. Lindman, On
the Viability, Cytotoxicity and Stability of Probiotic Bacteria Entrapped in Cellulose-Based Particles, Food Hydrocolloids, 2018, 82, 457–465 CrossRef CAS.
- N. H. Khan, D. R. Korber, N. H. Low and M. T. Nickerson, Development of Extrusion-Based Legume Protein Isolate-Alginate Capsules for the Protection and Delivery of the Acid Sensitive Probiotic, Bifidobacterium Adolescentis, Food Res. Int., 2013, 54, 730–737 CrossRef CAS.
- D. de L. Pedroso, M. Thomazini, R. J. B. Heinemann and C. S. Favaro-Trisndade, Protection of Bifidobacterium Lactis and Lactobacillus Acidophilus by Microencapsulation Using Spray-Chilling, Int. Dairy J., 2012, 26, 127–132 CrossRef CAS.
- N. Obradovic, I. Pajic-Lijakovic, T. Krunic, M. Belovic, M. Rakin and B. Bugarski, Effect of Encapsulated Probiotic Starter Culture on Rheological and Structural Properties of Natural Hydrogel Carriers Affected by Fermentation and Gastrointestinal Conditions, Food Biophys., 2020, 15, 18–31 CrossRef.
- H. Kumura, Y. Tanoue, M. Tsukahara, T. Tanaka and K. Shimazaki, Screening of Dairy Yeast Strains for Probiotic Applications, J. Dairy Sci., 2004, 87, 4050–4056 CrossRef CAS.
- G. K. Gbassi, T. Vandamme, S. Ennahar and E. Marchioni, Microencapsulation of Lactobacillus Plantarum Spp in an Alginate Matrix Coated with Whey Proteins, Int. J. Food Microbiol., 2009, 129, 103–105 CrossRef CAS PubMed.
- K. Sultana, G. Godward, N. Reynolds, R. Arumugaswamy, P. Peiris and K. Kailasapathy, Encapsulation of Probiotic Bacteria with Alginate-Starch and Evaluation of Survival in Simulated Gastrointestinal Conditions and in Yoghurt, Int. J. Food Microbiol., 2000, 62, 47–55 CrossRef CAS PubMed.
- B. Sánchez, M. C. Champomier-Vergès, B. Stuer-Lauridsen, P. Ruas-Madiedo, P. Anglade, F. Baraige, C. G. De Los Reyes-Gavilán, E. Johansen, M. Zagorec and A. Margolles, Adaptation and Response of Bifidobacterium Animalis Subsp. Lactis to Bile: A Proteomic and Physiological Approach, Appl. Environ. Microbiol., 2007, 73, 6757–6767 CrossRef PubMed.
- L. G. Gómez-Mascaraque, J. Ambrosio-Martín, R. Perez-Masiá and A. Lopez-Rubio, Optimization of Electrospraying Conditions for the Microencapsulation of Probiotics and Evaluation of Their Resistance during Storage and In vitro Digestion, J. Healthc. Eng., 2017, 2017, 1–6 CrossRef PubMed.
- A. Brodkorb, L. Egger, M. Alminger, P. Alvito, R. Assunção, S. Ballance, T. Bohn, C. Bourlieu-Lacanal, R. Boutrou, F. Carrière, A. Clemente, M. Corredig, D. Dupont, C. Dufour, C. Edwards, M. Golding, S. Karakaya, B. Kirkhus, S. Le Feunteun, U. Lesmes, A. Macierzanka, A. R. Mackie, C. Martins, S. Marze, D. J. McClements, O. Ménard, M. Minekus, R. Portmann, C. N. Santos, I. Souchon, R. P. Singh, G. E. Vegarud, M. S. J. Wickham, W. Weitschies and I. Recio, INFOGEST Static in Vitro Simulation of Gastrointestinal Food Digestion, Nat. Protoc., 2019, 14, 991–1014 CrossRef CAS PubMed.
- P. Singh, B. Medronho, M. G. Miguel and J. Esquena, On the Encapsulation and Viability of Probiotic Bacteria in Edible Carboxymethyl Cellulose-Gelatin Water-in-Water Emulsions, Food Hydrocolloids, 2018, 75, 41–50 CrossRef CAS.
- L. G. Gomez-Mascaraque, R. C. Morfin, R. Pérez-Masiá, G. Sanchez and A. Lopez-Rubio, Optimization of Electrospraying Conditions for the Microencapsulation of Probiotics and Evaluation of Their Resistance during Storage and In vitro Digestion, LWT–Food Sci. Technol., 2016, 69, 438–446 CrossRef CAS.
- T. Krunić, N. S. Obradović and M. B. Rakin, Application of Whey Protein and Whey Protein Hydrolysate as Protein Based Carrier for Probiotic Starter Culture, Food Chem., 2019, 293, 74–82 CrossRef PubMed.
- F. Valoppi, S. Calligaris, L. Barba and M. C. Nicoli, Compositional Phase Diagram, Rheological and Structural Properties of Systems Containing UHT Skim Milk, Sunflower Oil, Saturated Monoglycerides and Co-Surfactants, Food Biophys., 2015, 10, 94–102 CrossRef.
- L. Mao, S. Calligaris, L. Barba and S. Miao, Monoglyceride Self-Assembled Structure in O/W Emulsion: Formation, Characterization and Its Effect on Emulsion Properties, Food Res. Int., 2014, 58, 81–88 CrossRef CAS.
- H. Chen, L. Mao, Z. Hou, F. Yuan and Y. Gao, Roles of Additional Emulsifiers in the Structures of Emulsion Gels and Stability of Vitamin E, Food Hydrocolloids, 2020, 99, 105372 CrossRef CAS.
- W. Lu, A. L. Kelly and S. Miao, Improved Bioavailability of Encapsulated Bioactive Nutrients Delivered through Monoglyceride-Structured O/W Emulsions, J. Agric. Food Chem., 2017, 65, 3048–3055 CrossRef CAS PubMed.
- M. Minekus, M. Alminger, P. Alvito, S. Ballance, T. Bohn, C. Bourlieu, F. Carrière, R. Boutrou, M. Corredig, D. Dupont, C. Dufour, L. Egger, M. Golding, S. Karakaya, B. Kirkhus, S. Le Feunteun, U. Lesmes, A. MacIerzanka, A. MacKie, S. Marze, D. J. McClements, O. Ménard, I. Recio, C. N. Santos, R. P. Singh, G. E. Vegarud, M. S. J. Wickham, W. Weitschies and A. Brodkorb, A Standardised Static in Vitro Digestion Method Suitable for Food-an International Consensus, Food Funct., 2014, 5, 1113–1124 RSC.
- R. Deng, M. Mars, R. G. M. Van Der Sman, P. A. M. Smeets and A. E. M. Janssen, The Importance of Swelling for in Vitro Gastric Digestion of Whey Protein Gels, Food Chem., 2020, 330, 127182 CrossRef CAS PubMed.
- S. Calligaris, S. Da Pieve, G. Arrighetti and L. Barba, Effect of the Structure of Monoglyceride-Oil-Water Gels on Aroma Partition, Food Res. Int., 2010, 43, 671–677 CrossRef CAS.
- H. D. Batte, A. J. Wright, J. W. Rush, S. H. J. Idziak and A. G. Marangoni, Effect of Processing Conditions on the Structure of Monostearin-Oil-Water Gels, Food Res. Int., 2007, 40, 982–988 CrossRef CAS.
- H. D. Batte, A. J. Wright, J. W. Rush, S. H. J. Idziak and A. G. Marangoni, Phase Behavior, Stability, and Mesomorphism of Monostearin-Oil-Water Gels, Food Biophys., 2007, 2, 29–37 CrossRef.
- F. C. Wang and A. G. Marangoni, Effect of Intrinsic and Extrinsic Factors on the Stability of the α-Gel Phase of a Glyceryl Monostearate-Water System, RSC Adv., 2015, 5, 43121–43129 RSC.
-
N. Krog and F. V. Sparsø, in Food Emulsions, ed. J. Friberg, S. E. Larsson and K. Sjoblom, Marcel Dekker Inc., New York, 4th edn, 2004, pp. 45–91 Search PubMed.
- I. Heertje, E. C. Roijers and H. A. C. M. Hendrickx, Liquid Crystalline Phases in the Structuring of Food Products, LWT–Food Sci. Technol., 1998, 31, 387–396 CrossRef CAS.
- J. M. Leenhouts, R. A. Demel, B. De Kruijff and J. W. P. Boots, Charge-Dependent Insertion of β-Lactoglobulin into Monoglyceride Monolayers, Biochim. Biophys. Acta, Biomembr., 1997, 1330, 61–70 CrossRef CAS.
- Y. Zhang, J. Lin and Q. Zhong, The Increased Viability of Probiotic Lactobacillus Salivarius NRRL B-30514 Encapsulated in Emulsions with Multiple Lipid-Protein-Pectin Layers, Food Res. Int., 2015, 71, 9–15 CrossRef CAS.
- L. Salvia-Trujillo, S. H. E. Verkempinck, L. Sun, A. M. Van Loey, T. Grauwet and M. E. Hendrickx, Lipid Digestion, Micelle Formation and Carotenoid Bioaccessibility Kinetics: Influence of Emulsion Droplet Size, Food Chem., 2017, 229, 653–662 CrossRef CAS PubMed.
- S. Calligaris, M. Alongi, P. Lucci and M. Anese, Effect of Different Oleogelators on Lipolysis and Curcuminoid Bioaccessibility upon in Vitro Digestion of Sunflower Oil Oleogels, Food Chem., 2020, 314, 126146 CrossRef CAS PubMed.
- H. Singh, A. Ye and D. Horne, Structuring Food Emulsions in the Gastrointestinal Tract to Modify Lipid Digestion, Prog. Lipid Res., 2009, 48, 92–100 CrossRef CAS PubMed.
- P. M. Reis, T. W. Raab, J. Y. Chuat, M. E. Leser, R. Miller, H. J. Watzke and K. Holmberg, Influence of Surfactants on Lipase Fat Digestion in a Model Gastro-Intestinal System, Food Biophys., 2008, 3, 370–381 CrossRef PubMed.
- A. Ye, J. Cui and H. Singh, Effect of the Fat Globule Membrane on in Vitro Digestion of Milk Fat Globules with Pancreatic Lipase, Int. Dairy J., 2010, 20, 822–829 CrossRef CAS.
- M. Alongi, S. Calligaris and M. Anese, Fat Concentration and High-Pressure Homogenization Affect Chlorogenic Acid Bioaccessibility and α-Glucosidase Inhibitory Capacity of Milk-Based Coffee Beverages, J. Funct. Foods, 2019, 58, 130–137 CrossRef CAS.
- L. Salvia-Trujillo, C. Qian, O. Martín-Belloso and D. J. McClements, Influence of Particle Size on Lipid Digestion and β-Carotene Bioaccessibility in Emulsions and Nanoemulsions, Food Chem., 2013, 141, 1472–1480 CrossRef CAS PubMed.
- P. E. Ramos, L. Abrunhosa, A. Pinheiro, M. A. Cerqueira, C. Motta, I. Castanheira, M. V. Chandra-Hioe, J. Arcot, J. A. Teixeira and A. A. Vicente, Probiotic-Loaded Microcapsule System for Human in Situ Folate Production: Encapsulation and System Validation, Food Res. Int., 2016, 90, 25–32 CrossRef CAS PubMed.
- A. Sohail, M. S. Turner, A. Coombes, T. Bostrom and B. Bhandari, Survivability of Probiotics Encapsulated in Alginate Gel Microbeads Using a Novel Impinging Aerosols Method, Int. J. Food Microbiol., 2011, 145, 162–168 CrossRef CAS PubMed.
- O. D. Amund, Exploring the Relationship between Exposure to Technological and Gastrointestinal Stress and Probiotic Functional Properties of Lactobacilli and Bifidobacteria, Can. J. Microbiol., 2016, 62, 715–725 CrossRef CAS PubMed.
- A. C. Ouwehand, P. V. Kirjavainen, C. Shortt and S. Salminen, Probiotics: Mechanisms and Established Effects, Int. Dairy J., 1999, 9, 43–52 CrossRef.
- Q. Guo, A. Ye, H. Singh and D. Rousseau, Destructuring and Restructuring of Foods during Gastric Digestion, Compr. Rev. Food Sci. Food Saf., 2020, 19, 1658–1679 CrossRef PubMed.
- R. Bedani, A. D. S. Vieira, E. A. Rossi and S. M. I. Saad, Tropical Fruit Pulps Decreased Probiotic Survival to in Vitro Gastrointestinal Stress in Synbiotic Soy Yoghurt with Okara during Storage, LWT–Food Sci. Technol., 2014, 55, 436–443 CrossRef CAS.
- P. D. L. da Silva, M. de, F. Bezerra, K. M. O. dos Santos and R. T. P. Correia, Potentially Probiotic Ice Cream from Goat's Milk: Characterization and Cell Viability during Processing, Storage and Simulated Gastrointestinal Conditions, LWT–Food Sci. Technol., 2015, 62, 452–457 CrossRef.
- M. Saarela, I. Virkajärvi, H. L. Alakomi, P. Sigvart-Mattila and J. Mättö, Stability and Functionality of Freeze-Dried Probiotic Bifidobacterium Cells during Storage in Juice and Milk, Int. Dairy J., 2006, 16, 1477–1482 CrossRef CAS.
- D. J. McClements, E. A. Decker and Y. Park, Controlling Lipid Bioavailability through Physicochemical and Structural Approaches, Crit. Rev. Food Sci. Nutr., 2009, 49, 48–67 CrossRef PubMed.
Footnote |
† Electronic supplementary information (ESI) available. See DOI: 10.1039/d0fo01788d |
|
This journal is © The Royal Society of Chemistry 2021 |
Click here to see how this site uses Cookies. View our privacy policy here.