DOI:
10.1039/D0AN01719A
(Paper)
Analyst, 2021,
146, 118-123
The construction of a near-infrared fluorescent probe with dual advantages for imaging carbon monoxide in cells and in vivo†
Received
27th August 2020
, Accepted 24th September 2020
First published on 28th September 2020
Abstract
As a kind of toxic gas, carbon monoxide (CO) can hinder uptake of oxygen in humans. However, more and more studies have shown that CO is an important gaseous messenger in the body and playing an indispensable role in intracellular signaling pathways. So, it is necessary to develop an analytical method to study CO in living organisms. Although there are many CO-responsive probes, most of them have the disadvantages of a small Stokes shift or short emission wavelength. In order to address the above issue, a novel probe (FDX-CO) with a large Stokes shift (190 nm) and long emission wavelength (770 nm) was firstly synthesized to detect CO. The probe shows high sensitivity and superior selectivity toward CO. Moreover, the probe was successfully used for visualizing exogenous and endogenous CO in cells by fluorescence imaging, 3D quantification analysis and flow cytometric analysis. More importantly, FDX-CO could excellently detect CO in mice, which suggests that this probe has the potential ability to image CO in vivo. This probe can be viewed as a useful tool in the research of CO.
Introduction
Carbon monoxide (CO) is a kind of colorless and odorless gas molecule and is named an “invisible killer” due to its high toxicity. This could be explained because CO is very easy to combine with hemoglobin, leading to inadequate oxygen supply.1 However, scientific studies in recent years have shown that endogenous CO can be produced by heme metabolism and it is an important gaseous messenger in the human body.2 For example, CO plays an indispensable role in various signaling pathways including vasodilation, antiapoptosis and neurotransmission.3,4 It was reported that CO is also a potential drug and has positive effects on many diseases, acting, in particular, as an anti-inflammatory.5–7 Moreover, the abnormal up-regulation or down-regulation of CO was closely connected with heart disease, tumors, Alzheimer's disease, hypertension, inflammation, etc.8–11 Therefore, it is needed to develop an analytical method to visualize CO in living organisms.
Fluorescence imaging, as a real-time and in situ observation technique, has been widely used for marking and detecting functional molecules in bio-samples.12–17 Among them, molecular fluorescent probes based on organic fluorescent dyes have the advantages of simple operation and good selectivity. To date, although there are many CO-responsive fluorescent probes, their emission wavelengths are mainly located in the visible region.18–27 For example, the Tang group has reported a new probe for the detection of CO based on the fluorine boron pyrrole dye, which successfully visualizes intracellular CO up-regulation under hypoxia by this probe.28 Moreover, the Feng group has developed a probe based on naphthalimide derivatives. This probe was successfully used to rapidly detect CO in air, aqueous solution, and living cells providing the first promising ratiometric fluorescent probe system.29 But, none of the above work is suitable for imaging CO in vivo.
Near-infrared (NIR) fluorescence imaging technology is expected to solve the above issue because it can effectively reduce the absorption and scattering of light from tissues, improve the tissue penetration depth, and enhance the spatial resolution of fluorescence imaging.30–37 Recently, some NIR fluorescent probes have been designed in order to image CO.38–45 Our group reported a NIR fluorescent probe (CyAPC) and this probe was applied for imaging CO.38 But, this probe has a small Stokes shift (30 nm), which would lead to severe crosstalk between the excitation spectrum and emission spectrum, a low signal-to-noise ratio and severe fluorescence self-quenching in imaging. This would limit its application in biological imaging. The Qi group has successfully designed a probe (DC-CO) which is applied to detect CO in living cells.39 This probe possesses a short emission wavelength (685 nm) in spite of a large Stokes shift (120 nm) (Fig. 1A). Thus, it is urgent to develop an excellent probe with dual advantages (large Stokes shift and long emission wavelength).
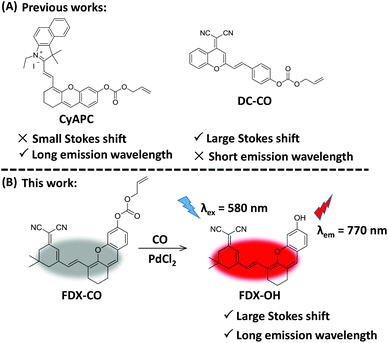 |
| Fig. 1 (A) The reported probe to detect CO. (B) The design of probe FDX-CO to CO with a large Stokes shift and long emission wavelength. | |
Herein, a NIR fluorescent probe (FDX-CO) was successfully designed (Fig. 1B). After reacting with CO and Pd2+, FDX-CO displays a large Stokes shift (190 nm) and long emission wavelength (770 nm) with a significant turn-on fluorescence response. More importantly, FDX-CO could visualize exogenous and endogenous CO in cells by fluorescence imaging, 3D quantification analysis and flow cytometric analysis. Furthermore, FDX-CO is successfully applied to detect CO in mice, which suggests that this probe has the ability to image CO in vivo. To the best of our knowledge, this structure was firstly used to design a CO-responsive NIR fluorescent probe with a large Stokes shift and long emission wavelength.
Results and discussion
Spectral response of FDX-CO to CO
Probe FDX-CO was synthesized according to the routes depicted in Scheme S1† and the 1H NMR, 13C NMR and mass spectra of probe FDX-CO are available in the ESI (Fig. S1–3†). The response of probe FDX-CO to CO was also studied. For probe FDX-CO, its own absorption is centered at 580 nm. After the probe reacted with CO (CORM-3, CO-releasing agent) and Pd2+, a boosted absorption in 580 nm is found (Fig. 2A). With the increasing concentration of CO, the fluorescence of FDX-CO is gradually increasing (Fig. 2B). As displayed in Fig. 2C, there is a good linear correlation between fluorescence intensity and CO concentrations from 1 to 30 μM (R2 = 0.9937). The detection limit is determined to be 0.33 μM (3σ/slope). These results suggest that FDX-CO could quantitatively analyse CO with high sensitivity.
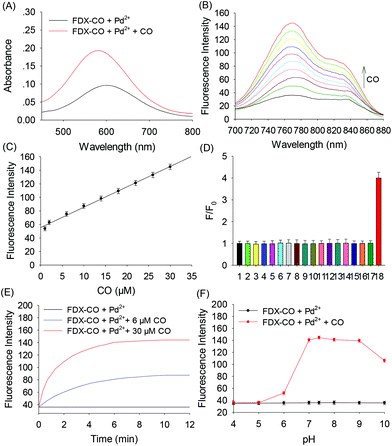 |
| Fig. 2 (A) The absorption spectra of FDX-CO (10 μM) and PdCl2 (10 μM) in the absence or presence of CO (30 μM) in PBS buffer solution (0.1 M, pH 7.4). (B) Fluorescence spectra of FDX-CO (10 μM) and PdCl2 (10 μM) upon the addition of CO (0, 1, 2, 6, 10, 14, 18, 22, 26, and 30 μM). (C) Linearity plots of the fluorescence intensity of probe FDX-CO and PdCl2 against CO concentrations. (D) Fluorescence response of FDX-CO (10 μM) and PdCl2 (10 μM) toward different analytes: 1, blank; 2, ClO−; 3, H2O2; 4, ONOO−; 5, NO2−; 6, HS−; 7, SO32−; 8, Cys; 9, Hcy; 10, GSH; 11, Ala; 12, Trp; 13, Glu; 14, Na+; 15, Ca2+; 16, Fe2+; 17, Zn2+; 18, CO. The concentration of CO is 30 μM and that of other analytes is 300 μM. (E) Time-dependent fluorescence intensity of FDX-CO (10 μM) and PdCl2 (10 μM) to CO (0, 6, and 30 μM). (F) Effect of pH on the fluorescence intensity of FDX-CO (10 μM) and PdCl2 (10 μM) before and after the addition of CO (30 μM). λex = 580 nm. | |
Moreover, the selectivity of probe FDX-CO was investigated by examining various potential interfering substances such as reactive species (ClO−, H2O2, ONOO−, NO2−, HS−, SO32−, Cys, Hcy, GSH, Ala, Trp, Glu, Na+, Ca2+, Fe2+, and Zn2+) (Fig. 2D and Fig. S4†). It could be seen that the above analytes have no obvious interference on the detection of CO. As shown in Fig. 2E, the fluorescence of FDX-CO increases with time and reaches a plateau within 8 min at various concentrations of CO (0, 6, and 30 μM). A big change of fluorescence intensity of FDX-CO for CO is achieved at pH 7.4 (Fig. 2F). Finally, the stability of probe FDX-CO was studied (Fig. S5†). There is almost no change of fluorescence after 10 days, which suggests that the probe has good stability. These results suggest that probe FDX-CO could be applied for detecting CO with outstanding preference under a physiological environment.
The reaction mechanism of FDX-CO
A possible reaction mechanism of FDX-CO with CO is proposed in Fig. 3A. FDX-CO has weak fluorescence owing to the protection of the hydroxyl group by the allyl formate unit. Once CO and PdCl2 were added, Pd2+ is firstly reduced into Pd0. Then, the allyl formate unit is cleaved from FDX-CO after Pd0 triggers the Tsuji–Trost reaction. This leads to the formation of FDX-OH and the recovery of a strong fluorescence signal.
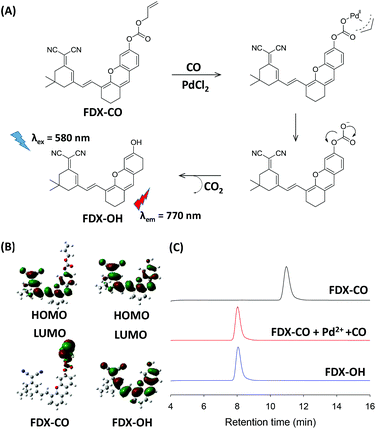 |
| Fig. 3 (A) The reaction mechanism of FDX-CO with CO. (B) Frontier molecular orbitals of FDX-CO and FDX-OH. (C) HPLC chromatograms of FDX-CO in the absence or presence of CO and Pd2+. The HPLC mobile phase was as follows: solvent A (CH3OH), solvent B (distilled water), A/B = 15/85 (V/V). | |
Then, density functional theory (DFT) calculations by the B3LYP/6-311G method using the Gaussian 09 package were carried out. The optimized structures of FDX-CO and FDX-OH are shown in Fig. S6.† The highest occupied molecular orbital (HOMO) and the lowest unoccupied molecular orbital (LUMO) of FDX-CO and FDX-OH are presented in Fig. 3B. The electron density on the HOMO of FDX-CO is located on the allyl formate group and the LUMO is positioned at the FDX unit. This shows that there is a PET process. So, FDX-CO is non-fluorescent. The electron densities on the HOMO and LUMO of FDX-OH are both primarily located on the FDX unit. No PET occurs in this system and FDX-OH shows strong fluorescence. The theory calculation well supports the change of fluorescence.
In order to verify the proposed mechanism, the mass spectrometry analysis was performed. The peak of FDX-CO is at m/z = 481.3. After reacting with CO and Pd2+, a new peak at m/z = 396.3 corresponding to FDX-OH appears (Fig. S7†). Meanwhile, HPLC experiments were also performed to confirm the reaction mechanism and the results are shown in Fig. 3C. FDX-CO itself displays a signal peak at 11.0 min. Upon adding CO and Pd2+, a decrease in the FDX-CO signal is seen along with the appearance of new signals at 8.0 min, which has the same retention time as FDX-OH. All the results display the CO-induced transformation of FDX-CO into FDX-OH.
Fluorescence imaging of exogenous CO in living cells
Before cell imaging, the cytotoxicity of probe FDX-CO was evaluated in HepG2 cells by conventional MTT assay (Fig. S8†). The result shows that the probe with different concentrations is of low cytotoxicity, which makes the probe exhibit good biocompatibility.
The capability of FDX-CO for imaging exogenous CO in HepG2 cells was investigated. As shown in Fig. 4A and B, the cells display a very weak fluorescence signal in the presence of FDX-CO, which reflects that there is no potential interference fluorescence. When treated with Pd2+, the cells did not emit a fluorescence signal. After the cells are treated with CORM-3 (a CO donor) and Pd2+, there is a remarkable fluorescence enhancement. And the fluorescence intensity gradually increases with the increase of CORM-3′ concentrations. Then, the experiment of fluorescence intensity with time was studied and the fluorescence intensity increased with time (Fig. S9†). With the purpose of observing the change of fluorescence intensity in three dimensions, 3D quantification analysis was performed and the results are shown in Fig. 4C. These results are consistent with the change of fluorescence intensity in the cells (Fig. 4D) and reflect that probe FDX-CO has the ability to detect exogenous CO in cells.
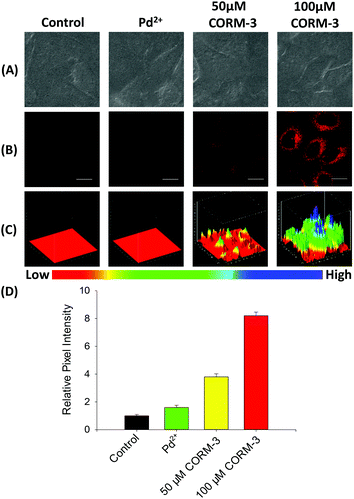 |
| Fig. 4 Fluorescence imaging of exogenous CO in cells. Control group: the cells were incubated with FDX-CO (10 μM) for 30 min. Pd2+ group: the cells were pretreated with Pd2+ (10 μM) for 30 min, and then incubated with FDX-CO (10 μM) for 30 min. 50 μM CORM-3 group: the cells were pretreated with 50 μM CORM-3 for 30 min and then treated with 10 μM FDX-CO and 10 μM PdCl2 for 30 min. 100 μM CORM-3 group: the cells were pre-incubated with 100 μM CORM-3 for 30 min, and then incubated with 10 μM FDX-CO and 10 μM PdCl2 for 30 min. λex = 568 nm, λem = 750–850 nm. Scale bar: 10 μm. (A) Bright field imaging of CO. (B) Fluorescence field imaging of CO. (C) 3D quantification analysis for the above groups. (D) Quantification analysis of (B). | |
Fluorescence imaging of endogenous CO in living cells
Probe FDX-CO was applied for detecting endogenous CO in HepG2 cells. As shown in Fig. 5A and B, the cells display a weak fluorescence signal after they were incubated with FDX-CO alone for 30 min. When the cells were treated with LPS or Heme, which would induce the generation of CO due to the over-expression of HO-1, a large increase in fluorescence is easily observed. To further confirm that the over-expression of CO was really caused by heme, zinc protoporphyrin (ZnPP) which was regarded as an inhibitor of HO-1 was chosen to incubate with cells. After the cells are incubated with ZnPP, the fluorescence intensity obviously decreases.
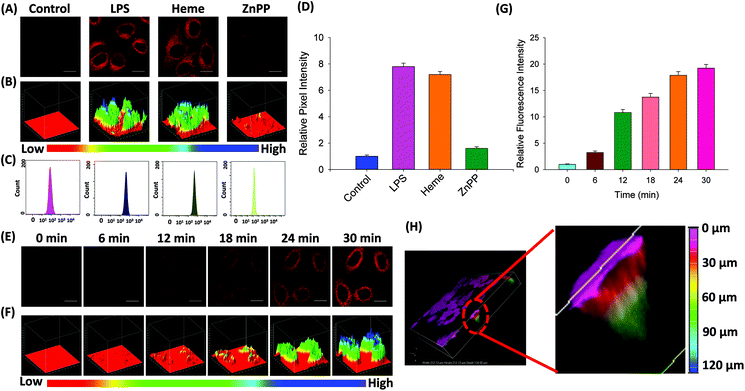 |
| Fig. 5 (A) Fluorescence imaging of endogenous CO in HepG2 cells. Control group: the cells were incubated with FDX-CO (10 μM) for 30 min. LPS group: the cells were pre-incubated with 1.0 μg mL−1 LPS for 12 h, and then incubated with 10 μM FDX-CO and 10 μM PdCl2 for 30 min. Heme group: the cells were pretreated with Heme (100 μM) for 10 h, and then incubated with FDX-CO (10 μM) and 10 μM PdCl2 for 30 min. ZnPP group: the cells were pretreated with 100 μM Heme for 2 h and 20 μM ZnPP for 2 h and then treated with 10 μM FDX-CO and 10 μM PdCl2 for 30 min. λex = 568 nm, λem = 750–850 nm. Scale bar: 10 μm. (B) 3D quantification analysis for the above groups. (C) The flow cytometric analysis for the above groups. (D) Quantification analysis of (A). (E) The time-dependent fluorescence response to CO in HepG2 cells. The cells were incubated with FDX-CO (10 μM) at different time points after pre-treatment in Heme (100 μM). (F) 3D quantification analysis of (E). (G) Quantification analysis of (E). (H) 3D fluorescence imaging of endogenous CO and the length of fluorescence imaging in HepG2 cells. | |
Similarly, 3D quantification analysis also was carried out to observe the change of fluorescence intensity in cells. In addition, flow cytometry experiments were carried out. As displayed in Fig. 5C, the movement of magnitude indicates the change of fluorescence intensity in the cells. This result was verified by quantification analysis (Fig. 5D). At the same time, the time-dependent fluorescence response to endogenous CO in cells was measured and the fluorescence was gradually enhanced (Fig. 5E), which was quantified by 3D quantification analysis (Fig. 5F) and quantification analysis (Fig. 5G). Finally, 3D fluorescence imaging of endogenous CO was firstly studied. As displayed in Fig. 5H, the cell was filled with fluorescence, which reveals that there is a certain amount of CO in the cells. More importantly, the length of fluorescence imaging was 126 μm. This result reflects that the probe has the potential ability for deep imaging. Taken together, probe FDX-CO is able to image the endogenous CO level in cells.
Fluorescence imaging of CO in mice
On the basis of NIR optical properties and excellent cell imaging, the fluorescence imaging of probe FDX-CO in vivo was performed (Fig. 6A). After the intravenous injection of FDX-CO, almost no fluorescence signal is found. When the mice were injected by CORM-3, a strong fluorescence signal is observed and increases with time. Then, 3D quantification analysis for the above groups was carried out and the results were consistent with the change of fluorescence intensity (Fig. 6B). This result was also verified by quantification analysis (Fig. 6C). The above results illustrate that probe FDX-CO is successfully applied for visualizing CO in vivo.
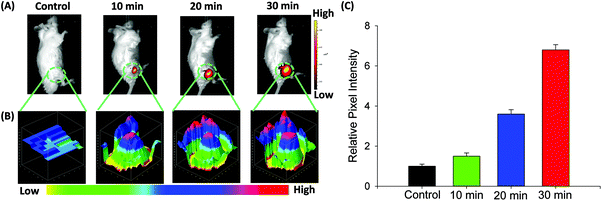 |
| Fig. 6 (A) Time-dependent fluorescence imaging of CO in mice. Control group: the mice were injected intravenously with 10 μM FDX-CO. Other groups: the mice were injected intravenously with 10 μM FDX-CO and 10 μM PdCl2, and then were injected intravenously with 10 μM CORM-3. λex = 600 nm, λem = 750–850 nm. (B) 3D quantification analysis of the fluorescence in (A). (C) Quantification analysis of (A). | |
Conclusions
In conclusion, we have reported a novel near-infrared (NIR) fluorescent probe (FDX-CO) to detect CO in solution, cells and in vivo with a large Stokes shift and long emission wavelength. Probe FDX-CO provides a low detection limit for CO (0.33 μM), which is sensitive enough for determining the CO level. The probe also shows outstanding selectivity to CO over other potential analytes under a physiological environment. Thanks to the minimal cytotoxicity of probe FDX-CO, the probe could monitor exogenous and endogenous CO in HepG2 cells by fluorescence imaging, 3D quantification analysis, and flow cytometry. In addition, the probe is successfully applied for visualizing CO in vivo. All in all, the probe FDX-CO could provide a useful tool for the research of CO in the future.
Ethical statement
All experiments were performed in accordance with the Guidelines “Regulations of Hunan Province on the administration of experimental animals” and approved by the ethics committee at Xiangtan university.
Conflicts of interest
There are no conflicts to declare.
Acknowledgements
This work was supported by the National Natural Science Foundation of China (21775133), Hunan Provincial Natural Science Foundation (2018JJ2385), Scientific Research Fund of Hunan Provincial Education Department (19A479), Degree & Postgraduate Education Reform Project of Hunan Province (2019JGYB113) and Degree & Postgraduate Education Reform Project of Hunan Province (CX20190483, XDCX2020B115).
Notes and references
- L. K. Weaver, N. Engl. J. Med., 2009, 360, 1217–1225 CrossRef CAS.
- A. Verma, D. J. Hirsch, C. E. Glatt, G. V. Ronnett and S. H. Snyder, Science, 1993, 259, 381–384 CrossRef CAS.
- E. Bathoorn, D. J. Slebos, D. Postma, G. Koeter, A. Oosterhout, M. Toorn, H. Boezen and H. Kerstjens, Eur. Respir. J., 2007, 30, 1131–1137 CrossRef CAS.
- R. Motterlini and L. E. Otterbein, Nat. Rev. Drug Discovery, 2010, 9, 728–743 CrossRef CAS.
- S. H. Heinemann, T. Hoshi, M. Westerhausen and A. Schiller, Chem. Commun., 2014, 50, 3644–3660 RSC.
- L. Yuan, W. Lin, L. Tan, K. Zheng and W. Huang, Angew. Chem., Int. Ed., 2013, 52, 1628–1630 CrossRef CAS.
- L. Y. Wu and R. Wang, Pharmacol. Rev., 2005, 57, 585–630 CrossRef CAS.
- D. R. Premkumar, M. A. Smith, P. L. Richey, R. B. Petersen, R. Castellani, R. K. Kutty, B. Wiggert, G. Perry and R. N. Kalaria, J. Neurochem., 1995, 65, 1399–1402 CrossRef CAS.
- R. A. Schroeder, C. A. Ewing, J. V. Sitzmann and P. C. Kuo, Dig. Dis. Sci., 2000, 45, 2405–2410 CrossRef CAS.
- I. T. Lee, S. F. Luo, C. W. Lee, S. W. Wang, C. C. Lin, C. C. Chang, Y. L. Chen, L. Y. Chau and C. M. Yang, Am. J. Pathol., 2009, 175, 519–532 CrossRef CAS.
- V. S. Raju, N. Imai and C. S. Liang, J. Mol. Cell. Cardiol., 1999, 31, 1581–1589 CrossRef CAS.
- C. X. Yin, F. J. Huo, J. J. Zhang, R. Martinez-Manez, Y. T. Yang, H. G. Lv and S. D. Li, Chem. Soc. Rev., 2013, 42, 6032–6059 RSC.
- H. W. Liu, L. L. Chen, C. Y. Xu, Z. Li, H. Y. Zhang, X. B. Zhang and W. H. Tan, Chem. Soc. Rev., 2018, 47, 7140–7180 RSC.
- Z. Yang, J. Cao, Y. He, J. H. Yang, T. Kim, X. Peng and J. S. Kim, Chem. Soc. Rev., 2014, 43, 4563–4601 RSC.
- Z. Guo, S. Park, J. Yoon and I. Shin, Chem. Soc. Rev., 2014, 43, 16–29 RSC.
- H. Zhu, C. Liu, C. Liang, B. Tian, H. Zhang, X. Zhang, W. Sheng, Y. Yu, S. Huang and B. Zhu, Chem. Commun., 2020, 56, 4086–4089 RSC.
- H. Zhu, C. Liang, X. Cai, H. Zhang, C. Liu, P. Jia, Z. Li, Y. Yu, X. Zhang, W. Sheng and B. Zhu, Anal. Chem., 2020, 92, 1883–1889 CrossRef CAS.
- B. W. Michel, A. R. Lippert and C. J. Chang, J. Am. Chem. Soc., 2012, 134, 15668–15671 CrossRef CAS.
- Z. Xu, J. Yan, J. Li, P. Yao, J. Tan and L. Zhang, Tetrahedron Lett., 2016, 57, 2927–2930 CrossRef CAS.
- W. Feng, D. Liu, S. Feng and G. Feng, Anal. Chem., 2016, 88, 10648–10653 CrossRef CAS.
- S. Feng, D. Liu, W. Feng and G. Feng, Anal. Chem., 2017, 89, 3754–3760 CrossRef CAS.
- K. Zheng, W. Lin, L. Tan, H. Chen and H. Cui, Chem. Sci., 2014, 5, 3439–3448 RSC.
- M. Sun, H. Yu, K. Zhang, S. Wang, T. Hayat, A. Alsaedi and D. Huang, ACS Sens., 2018, 3, 285–289 CrossRef CAS.
- W. Feng, D. Liu, Q. Zhai and G. Feng, Sens. Actuators, B, 2017, 240, 625–630 CrossRef CAS.
- S. Pal, M. Mukherjee, B. Sen, S. K. Mandal, S. Lohar, P. Chattopadhyay and K. Dhara, Chem. Commun., 2015, 51, 4410–4413 RSC.
- W. Feng, S. Feng and G. Feng, Chem. Commun., 2019, 55, 8987–8990 RSC.
- W. Feng, S. Feng and G. Feng, Anal. Chem., 2019, 91, 8602–8606 CrossRef CAS.
- Y. Li, X. Wang, J. Yang, X. Xie, M. Li, J. Niu, L. Tong and B. Tang, Anal. Chem., 2016, 88, 11154–11159 CrossRef CAS.
- W. Feng, J. Hong and G. Feng, Sens. Actuators, B, 2017, 251, 389–395 CrossRef CAS.
- X. Luo, R. Wang, C. Lv, G. Chen, J. You and F. Yu, Anal. Chem., 2020, 92, 1589–1597 CrossRef CAS.
- Y. Qi, Y. Huang, B. Li, F. Zeng and S. Wu, Anal. Chem., 2018, 90, 1014–1020 CrossRef CAS.
- A. Shao, Y. Xie, S. Zhu, Z. Guo, S. Zhu, J. Guo, P. Shi, T. D. James, H. Tian and W. Zhu, Angew. Chem., Int. Ed., 2015, 54, 7275–7280 CrossRef CAS.
- Z. Q. Mao, H. Jiang, X. J. Song, W. Hu and Z. H. Liu, Anal. Chem., 2017, 89, 9620–9624 CrossRef CAS.
- S. Gong, E. Zhou, J. Hong and G. Feng, Anal. Chem., 2019, 91, 13136–13142 CrossRef CAS.
- Y. Liu, L. Teng, L. Chen, H. Ma, H. W. Liu and X. B. Zhang, Chem. Sci., 2018, 9, 5347–5353 RSC.
- Y. Huang, Y. Qi, C. Zhan, F. Zeng and S. Wu, Anal. Chem., 2019, 91, 8085–8092 CrossRef CAS.
- J. Ouyang, L. Sun, Z. Zeng, C. Zeng, F. Zeng and S. Wu, Angew. Chem., 2020, 59, 10111–10121 CrossRef CAS.
- S. J. Li, D. Y. Zhou, Y. F. Li, B. Yang, J. Ou-Yang, J. Jie, J. Liu and C. Y. Li, Talanta, 2018, 188, 691–700 CrossRef CAS.
- L. Yan, D. Nan, C. Lin, Y. Wan, Q. Pan and Z. Qi, Spectrochim. Acta, Part A, 2018, 202, 284–289 CrossRef CAS.
- J. Hong, Q. Xia, E. Zhou and G. Feng, Talanta, 2020, 215, 120914 CrossRef CAS.
- E. Zhou, S. Gong, J. Hong and G. Feng, Spectrochim. Acta, Part A, 2020, 227, 117657 CrossRef CAS.
- E. Zhou, S. Gong and G. Feng, Sens. Actuators, B, 2019, 301, 127075 CrossRef CAS.
- Y. Deng, J. Hong, E. Zhou and G. Feng, Dyes Pigm., 2019, 170, 107634 CrossRef CAS.
- S. Gong, J. Hong, E. Zhou and G. Feng, Talanta, 2019, 201, 40–45 CrossRef CAS.
- W. Feng and G. Feng, Sens. Actuators, B, 2018, 255, 2314–2320 CrossRef CAS.
Footnote |
† Electronic supplementary information (ESI) available: Experimental section, additional spectroscopic data, cell cytotoxicity and supplemental fluorescence images in biological samples. See DOI: 10.1039/d0an01719a |
|
This journal is © The Royal Society of Chemistry 2021 |
Click here to see how this site uses Cookies. View our privacy policy here.