DOI:
10.1039/D0AN01703E
(Paper)
Analyst, 2021,
146, 305-314
A cyclodextrin-decorated plasmonic gold nanosatellite substrate for selective detection of bipyridylium pesticides†
Received
25th August 2020
, Accepted 6th October 2020
First published on 8th October 2020
Abstract
A cyclodextrin-decorated gold nanosatellite (AuNSL) substrate was developed as a surface-enhanced Raman scattering sensor for the selective sensing of bipyridylium pesticides such as paraquat (PQ), diquat (DQ), and difenzoquat (DIF). The AuNSL structure was fabricated via vacuum deposition of gold nanoparticles (AuNPs) on a gold nanopillar substrate, and a large density of hot-spots was formed for Raman signal enhancement. Thiolated β-cyclodextrin (SH-CD) was surface-modified on the AuNSL as a chemical receptor. The detection limit of PQ, DQ, and DIF on the SH-CD-coated AuNSL (CD-AuNSL) was 0.05 ppm for each, and showed linear correlation in a concentration range of 10 ppm–0.05 ppm. Then, selective bipyridylium pesticide detection was performed by comparing the Raman intensity of each pesticide with and without the washing step. After the washing step, 90% of the PQ, DQ, and DIF Raman signals were maintained on the CD-AuNSL substrate with a uniform selectivity in a mapping area of 200 μm × 200 μm. Furthermore, selective pesticide detection was performed using a ground-apple solution without pretreatment. Raman signals were clearly observed after the washing step and they showed a limit of detection down to a concentration of 0.05 ppm for each pesticide. Principal component analysis (PCA) of the binary and ternary mixtures of PQ, DQ, and DIF showed that each component could be easily identified via the typical Raman fingerprint analysis. The developed CD-AuNSL is expected to be applied for various chemical sensors, especially for pyridine-containing toxic substances in the environment and metabolite biomarkers in biofluids.
1. Introduction
Surface-Enhanced Raman Scattering (SERS) is a Raman signal amplification technology that provides intrinsic molecular vibrational signals with ultrahigh sensitivity.1,2 On a nanostructured noble metal surface, Raman scattering is maximized at the positions of “hot-spots” which are nanogaps between plasmonic nanoparticles or nanoarchitectures.3,4 Due to the advantage of Raman scattering, which enables the label-free identification of molecules, SERS has been widely utilized in chemical sensors for applications such as environmental sensing, explosive detection, drug detection, and healthcare monitoring.5–8 However, in complicated matrix media such as environmental samples, biological fluids and agricultural products, the Raman signals of the matrix molecules are enhanced together with those of the target molecule generating background Raman signals. Furthermore, competitive adhesion of the matrix and target molecules on hot-spots lowers the sensitivity of SERS sensors.9–11 Therefore, various kinds of receptors have been functionalized on the SERS sensor surface for selective molecular detection. For the direct capture and detection of chemical targets, surface modification of charged-molecules is used to induce electrostatic binding of the target molecule on the sensor surface.12–14 In addition, 2D materials such as graphene,15 hBN,5 and MoS216 are coated on the sensor surface to capture chemical targets through π–π stacking, hydrogen bonding, and hydrophobic interactions. However, charged-molecules and 2D materials sometimes generate their Raman signals as a background, and the binding for targets is not target-specific. On the other hand, a macrocycle, a cyclic framework of a molecular subunit, is coated on the sensor surface that can capture the target molecule through host–guest chemistry. Even though macrocycles are known to exhibit a high binding affinity with target molecules, preparation of macrocycle derivatives for surface functionalization requires several chemical modification steps, which limits their utilization.17,18
Cyclodextrin (CD) is one type of a representative macrocycle composed of cyclic glucose subunits. Because CDs have hydrophobic interiors and hydrophilic exteriors in their structures, they exhibit selectivity for molecules with a hydrophobic center, such as a benzene ring that is surrounded by hydrophilic functional groups.19 Compared to other macrocycles, various types of CD derivatives have been developed and they are commercially available. There are several reports on CD derivative-coated SERS materials such as Au and Ag nanoparticles,20–22 particle or template-assisted metal substrates,23–25 and so on. Many studies have focused on developing a proof-of-concept for selective sensing rather than demonstrating the use of real sample targets for practical applications.
In this work, a plasmonic gold nanosatellite (AuNSL) substrate that shows high productivity, sensitivity and signal uniformity was prepared by a vacuum deposition process, as reported previously.26 On the surface of the AuNSL substrate, mono-6-mercapto-6-deoxy-beta-cyclodextrin (SH-CD) was functionalized as a chemical receptor and utilized for selective bipyridylium pesticide detection from an apple matrix, as described in Fig. 1. Because of the large surface area and a large density of hot-spots, SH-CD was densely coated on the AuNSL surface and showed a uniform selectivity and sensitivity for bipyridylium pesticides, such as paraquat (PQ), diquat (DQ), and difenzoquat (DIF), down to a concentration of 0.05 ppm for each. Furthermore, from the ground-apple samples that were spiked with PQ, DQ, and DIF, each pesticide could be selectively detected down to a concentration of 0.05 ppm after simple dropping and washing of the substrate. From the results, the developed CD-AuNSL SERS substrate is expected to be applied for various selective SERS sensors such as toxin detection from environmental water, chemical bio-marker detection from biological fluids, and residual pesticide detection from agricultural products.
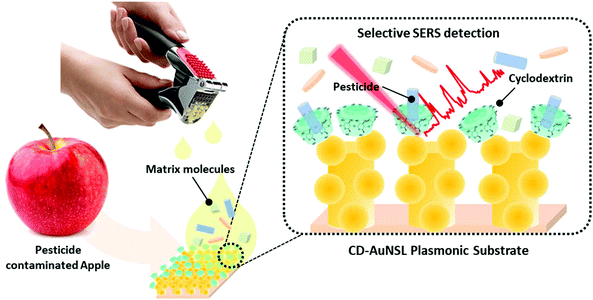 |
| Fig. 1 Schematic illustrations of the CD-AuNSL substrate for selective pesticide detection. | |
2. Experimental section
2.1. Materials
Mono-6-mercapto-6-deoxy-beta-cyclodextrin was purchased from Cosmo Bio (Tokyo, Japan). DTNB (Ellmam's Reagent) was obtained from Thermo Fisher Scientific (Waltham, MA, USA). Paraquat, diquat, difenzoquat, azinphos-methyl, benomyl, fuberidazole, thiram, mancozeb and the PFDT solution were purchased from Sigma-Aldrich (St Louis, MO, USA). The PET substrate with a thickness of 188 μm was purchased from Toray (Seoul, Korea). Apple was obtained from a local market store and used after cleaning its surface.
2.2. Preparation and surface modification of the AuNSL substrate
PET protrusions were fabricated by Ar plasma etching on a PET film with an Ar flow rate and working pressure of 5 sccm and 80 mTorr, respectively. Then, 100 nm of Au was deposited on the PET protrusions at a rate of 2.0 Å s−1 using a thermal evaporator system (LAT, Korea) to prepare the Au nanopillar substrate. On the Au nanopillar, PFDT was coated by evaporation and then decorated with satellite AuNPs using thermal evaporation with a deposition rate of 0.3 Å s−1. The surface morphology of the AuNSL was observed by field-emission scanning electron microscopy (FE-SEM, JSM-6700, JEOL). The absorbance spectra of the Au nanopillars and AuNSL were recorded using a UV-vis spectrophotometer (Agilent, USA). The as-prepared AuNSL with dimensions of 1 cm × 1 cm was immersed in 1 mL of a SH-CD solution with a concentration of 1 mM for 24 h. Then, the CD-coated AuNSL surface was cleaned three times with fresh DI water to remove the unbound SH-CD molecules. The amount of SH-CD adhering on the AuNSL surface was calculated by Ellman's assay for thiol quantification before and after the coating process, according to the manufacturer's protocol. The absorbance of the Ellman's reagent reactants was measured using a microplate absorbance reader (SpectraMaxM2 plate reader, Molecular Devices). The contact angles of the AuNSL substrate before and after the coating of SH-CD were measured using a contact angle analyzer (FEMTOFAB, SDlab-200TEZ, South Korea). For pesticide detection experiments, the CD-AuNSL was cut into 0.5 cm × 0.5 cm pieces before use.
2.3. Spectral analysis of CD and the pesticide complex
PQ, DQ, DIF, and SH-CD at a concentration of 500 μM were prepared and 50 μL of each solution was dropped on Si wafer. After complete drying, the infrared spectrum of each sample was recorded using an FT-IR instrument (FT-IR, ID5 ATR, Sinco Nicolet). Then, SH-CD and the pesticide mixture solution were prepared at a final SH-CD and pesticide concentration of 500 μM for each and then measured by FT-IR as performed above.
2.4. Binding affinity test of CD for bipyridylium pesticides
The impedance spectra were recorded from 100 kHz to 100 mHz at a sampling rate of five points per decade (AC amplitude: 10 mV) with an electrochemical cell that used screen-printed Au electrodes (working electrode), Ag/AgCl (in 3 M NaCl, reference electrode), and Pt wire (counter electrode). The SH-CD was coated on the Au electrode before measurement. The data of each layer were fitted with a Randle circuit to obtain the electron transfer resistance (Ret) values using Zview 2 software. The dissociation constant (Kd) of the CD was calculated according to the equation ΔR/R0 = (ΔR0/R0)max [C/(Kd + C)], where (ΔR0/R0)max is the maximum Ret variation and C is the target concentration.
2.5. Limit of detection test for bipyridylium pesticides
PQ, DQ, and DIF solutions at various concentrations of 10 ppm, 5 ppm, 1 ppm, 0.5 ppm, 0.1 ppm, and 0.05 ppm were prepared. On the AuNSL substrate with and without an SH-CD coating, 10 μL of each solution was dropped and dried. The Raman spectra at the various concentrations of pesticides were recorded from 10 random positions of the substrate and then averaged for plotting (n = 3). The standard curve of the Raman spectra was obtained after measuring the Raman intensity of the typical peak positions of PQ, DQ, and DIF from the background at 1640 cm−1, 1570 cm−1, and 998 cm−1, respectively. Raman spectra were recorded using a portable Raman instrument (NS200, Nanoscope Systems, Inc., Daejeon, Korea) with a wavelength, a laser power, and an exposure time of 785 nm, 5 mW and 5 s, respectively. All the spectra obtained in this work used the same Raman measurement conditions.
2.6. Selective pesticide detection test using the CD-AuNSL substrate
Ten microliters of PQ, DQ, and DIF solutions dissolved in DI water with a concentration of 10 ppm was dropped on the CD-AuNSL substrate and dried for use as samples without washing. Additionally, the same pesticide solutions were dropped on the CD-AuNSL substrate and then washed using 10 mL of DI water after 10 min to prepare the samples with the washing step. The Raman intensity values of the samples with and without washing were measured and compared for the selectivity test. The experiment was repeated three times, and the Raman signal was measured from 10 random points for each experiment and then plotted after averaging. The capture efficiency was calculated using the following formula at a typical Raman peak position for each pesticide.
The Raman mapping of PQ, DQ, and DIF on CD-AuNSL after washing was carried out for a mapping area of 200 μm × 200 μm wth a measurement interval of 10 μm.
2.7. Pesticide detection in ground-apple solution
An apple was cut into a piece (3 g) and it was ground using a garlic press. The ground-apple solution was spiked with PQ, DQ, and DIF to achieve a concentration of 10 ppm. For the selectivity test, 10 μL of the solution was dropped on the CD-AuNSL substrate for 10 min and then washed with 10 mL of fresh DI water three times. Raman spectra were obtained using a portable Raman instrument with a wavelength, a laser power, and an exposure time of 785 nm, 5 mW and 5 s, respectively. Single, binary, and ternary mixtures of PQ, DQ, and DIF were prepared with a final concentration of each pesticide at 10 ppm and then measured on the CD-AuNSL after washing. Then PCA was performed on the Raman spectra, preprocessed with a baseline correction algorithm with asymmetric least squares smoothing and normalization according to a previous study.27
3. Results and discussion
3.1. Characterization of the CD-AuNSL substrate
The AuNSL substrate was prepared according to our previous work.26 The polyethylene terephthalate (PET) film was treated by a surface etching process using Ar-plasma that forms nanoprotrusions, as shown in Fig. S1a.† An Au film was coated on the surface of the nanoprotrusions to form an Au nanopillar structure (Fig. S1b†), and then perfluorodecanethiol (PFDT) was coated as an interlayer. Satellite AuNPs were further decorated on the PFDT-treated Au nanopillar to prepare the AuNSL substrate. The surface morphology of the AuNSL was observed by FE-SEM, as shown in Fig. 2a and Fig. S1c.† The average size of the satellite AuNPs was 67 nm ± 10 nm which was determined using ImageJ software (Fig. S1d†). In Fig. 2b, the UV-vis absorbance spectra of the Au nanopillars and PFDT-treated Au nanopillars show an SPR peak position of 520 nm, which arose from the sphere-shaped Au, formed along the shape of PET protrusions.26 After fabrication of the satellite AuNPs on the Au nanopillars, the red shift of the SPR peak to 550 nm and peak broadening were observed due to the decorated particles and their size distribution. In the AuNSL structure, hot-spots are formed at the nanogaps created between the satellite AuNPs and Au nanopillars, and are also formed between the adjacent satellite AuNPs. To prepare the CD-AuNSL, the AuNSL substrate was immersed in an SH-CD solution with a concentration of 1 mM for 24 h and then washed three times with fresh DI water to remove unbound SH-CD molecules. The amount of SH-CD reaction on the AuNSL substrate was calculated by Ellman's assay, which quantifies the thiol content in the solution. SH-CD solutions at a concentration range of 2000 μM to 31.25 μM were prepared and reacted with the Ellman's reagent. After 15 min, the absorbance spectra were obtained and they clearly showed a concentration dependent absorbance peak increase at 412 nm and the disulfide formation of 5,5′-dithiobis-(2-nitrobenzoic acid (DTNB2−) with SH-CD, forming 2-nitro-5-thiobenzoate anion (TNB2−) molecules to develop a dark yellow color for quantification (Fig. 2c). The absorbance of the reactants was linearly correlated with the concentration of SH-CD. Then, the concentration changes of the supernatant SH-CD solution before and after coating on the AuNSL substrate with dimensions of 1 cm × 1 cm were calculated using the standard curve in Fig. 2c. As shown in Fig. 2d, an absorbance decrease was observed and calculated as a reduction of 99.5 nmol of SH-CD after the reaction, which can be considered as an excess amount coating of SH-CD on the AuNSL surface. There can be a physical adsorption and loss of chemicals after reaction for 24 h, but it can be considered that the surface-functionalized CDs are in a saturated state. After the coating of SH-CD on the AuNSL, the substrate properties changed from hydrophobic to hydrophilic due to the coating of CD, which contains a hydrophilic glucose subunit in its structure. The contact angle of AuNSL with 128.3° was changed to 9.7° after surface modification (Fig. 2e and f).
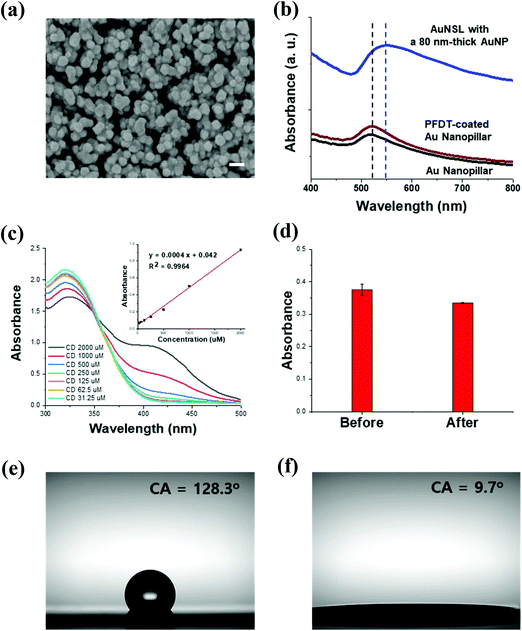 |
| Fig. 2 Characterization of AuNSL and CD functionalization. (a) SEM image of the AuNSL substrate (scale bar = 100 μm), (b) UV-vis absorbance spectra of the Au nanopillar, PFDT-coated Au nanopillar, and AuNSL, (c) UV-vis absorbance spectra of DTNB2− reacted with SH-CD at various concentrations and their standard curve (inset), (d) absorbance changes of the SH-CD/DTNB reactant before and after coating on the AuNSL, and (e) contact angles of the AuNSL and (f) CD-AuNSL. | |
3.2. SERS characterization of CD-AuNSL
The detection capability of the prepared CD-AuNSL was tested using the bipyridylium pesticides of PQ, DQ, and DIF. Each pesticide exhibits different Raman peak patterns and 1640 cm−1, 1570 cm−1, and 998 cm−1 were selected as the typical peaks of PQ, DQ and DIF, respectively. To test the detection limit, each pesticide at concentrations of 10 ppm, 5 ppm, 1 ppm, 0.5 ppm, 0.1 ppm and 0.05 ppm was prepared and 10 μL of each solution was dropped on the CD-AuNSL substrate. After drying, the Raman spectra were obtained from 10 random positions of the substrate and then averaged for plotting. As shown in Fig. 3a, the detection limit for PQ was 0.05 ppm and the Raman intensity was linearly correlated with the PQ concentrations in a range of 10 ppm–0.05 ppm with an R2 value of 0.98. Similarly, as shown in Fig. 3b for DQ and Fig. 3c for DIF, each pesticide was detected down to a concentration of 0.05 ppm and the Raman intensity depended linearly on various molecular concentrations with a R2 value of 0.97 for each DQ and DIF. The SERS detection capability of the CD-AuNSL was not significantly affected by the surface-functionalized CD according to the Raman measurement results of the AuNSL for PQ, DQ, and DIF, which showed similar detection limits of 0.05 ppm for each (Fig. S2†). Because beta-CD is a supramolecular receptor with an outer diameter of 1.54 nm and a height of 0.79 nm, hot-spot blocking by the dimensions of the receptor molecules was not significant.28,29 In addition, it was expected that the target molecules included inside the surface-functionalized CD enabled molecules to closely approach the hot-spots, maintaining the sensitivity of the SERS substrate. From these results, it is found that the CD-AuNSL substrate shows a sensitive pesticide detection capability, which can be further tested for selective molecular detection tests.
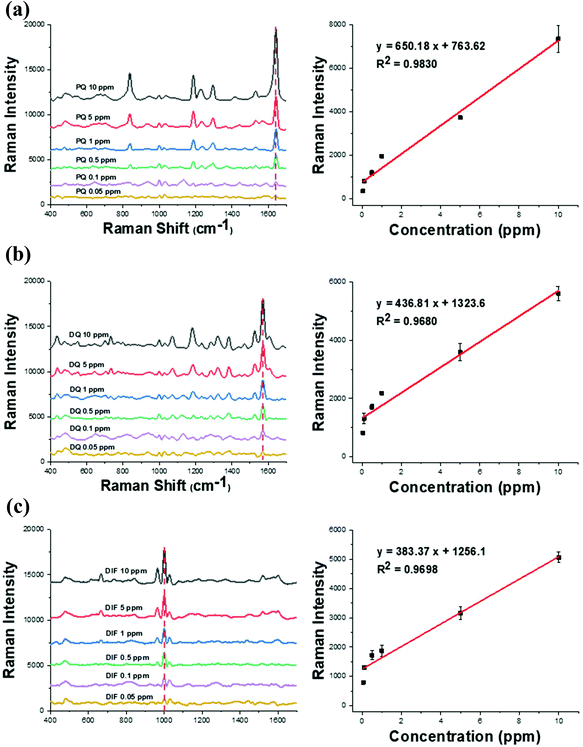 |
| Fig. 3 Raman spectra and standard curve obtained at various concentrations of the bipyridylium pesticide on the CD-AuNSL. (a) PQ (b) DQ, and (c) DIF. | |
3.3. Selectivity test of CD-AuNSL for bipyridylium pesticides
The selectivity of CD-AuNSL for bipyridylium pesticides was tested by comparing the Raman intensities before and after the washing step. PQ, DQ, and DIF were dissolved in DI water to obtain solutions with a concentration of 10 ppm, and 10 μL of each solution was dropped on the CD-AuNSL substrate. After the complete drying of the sample, Raman spectra were obtained for the samples without washing. To compare the samples prepared with the washing step, 10 ppm of each pesticide solution (10 μL) was dropped on the CD-AuNSL substrate and then, after 10 min, the substrates were washed with 10 mL of fresh DI water for 1 min. As shown in Fig. 4a, the Raman intensities of PQ, DQ, and DIF remained comparable to those of the samples without washing. The capture efficiency of the CD-AuNSL for each pesticide was calculated according to the formula in the Experimental section. Interestingly, 91.8%, 88.3%, and 92.1% of the Raman intensity were maintained for PQ, DQ, and DIF, respectively (Fig. 4b). As controls, PQ, DQ, and DIF with and without washing on the AuNSL were tested. As shown in Fig. S3a† no significant Raman peaks were observed for each pesticide after the washing step. Furthermore, the selectivity of the CD-AuNSL for other pesticides such as azinphos-methyl, benomyl, fuberidazole, thiram, and mancozeb was investigated (chemical structures are shown in Fig. S3b†). Each pesticide dissolved in DI water with a concentration of 10 ppm (5 ppm for mancozeb) was dropped on the bare AuNSL and CD-AuNSL substrates, and then, the Raman spectra were recorded. As shown in Fig. S3c–g,† on the bare AuNSL, clear Raman peaks of each pesticide were observed. On the CD-AuNSL, even though the Raman peaks for these pesticides were detected, the intensity was dramatically reduced compared to that on the bare AuNSL for both of the samples with and without washing. As target molecules for CD, other kinds of pesticides can also be captured in CD through the host–guest chemistry. However, the inclusion forming efficiency is dependent on the chemical structure of the target, reaction time, surface properties of the functionalized-surface and so on. Therefore, considering the results of Fig. 4 and Fig. S3,† CD on AuNSL acted as a receptor, more efficiently for bipyridylium pesticides in the assay method used in this work. Previously, it was shown that bipyridylium pesticides can form an inclusion complex with beta-cyclodextrin and those selective capture properties were utilized for several sensor applications.30–33 To confirm the complex formation, FT-IR analysis of SH-CD and the mixtures prepared with each pesticide at a ratio of 1
:
1 was performed. As shown in Fig. S4a–c,† PQ, DQ, DIF, and SH-CD showed their typical IR spectra and the vibrational peak assignment for each molecule is summarized in Fig. S4d.† In particular, SH-CD exhibited peaks attributed to OH stretching, CH stretching, H–O–H bending, C–C stretching, and C–O–C stretching at ∼3265 cm−1, ∼2910 cm−1, ∼1646 cm−1, ∼1151 cm−1, and ∼1021 cm−1, respectively. From the SH-CD and pesticide mixture spectra, the FT-IR peak of each pesticide disappeared while the SH-CD spectrum remained, which strongly indicated the complex formation of host molecules in the cavity of CD.34,35 Furthermore, the binding affinity of CD for PQ, DQ, and DIF was tested using impedance spectroscopy according to a previously reported method (Fig. S5†).36,37 Each pesticide was reacted at a concentration range of 0.5 ppm to 1000 ppm, and the surface impedance increase was observed due to the interruption of electron transfer on the electrode surface by the target molecules. Above the concentration of 100 ppm, the impedance change was saturated and the dissociation constant (Kd) for each pesticide was calculated to be 155 μM, 124 μM, and 136 μM, respectively. From the results, it is found that the surface-coated CD on the AuNSL functioned as a receptor for PQ, DQ, and DIF through host–guest chemistry, and the captured molecules were detected by Raman spectroscopy in a label-free method. On the other hand, the CD-AuNSL was further characterized by Raman mapping in an area of 200 μm × 200 μm with 10 μm intervals after the pesticides were dropped and washed. As shown in Fig. 4c–e, PQ, DQ, and DIF showed a uniform selectivity in the region of the measurement with a CV value of 4.3%, 7.0%, and 8.5%, respectively.
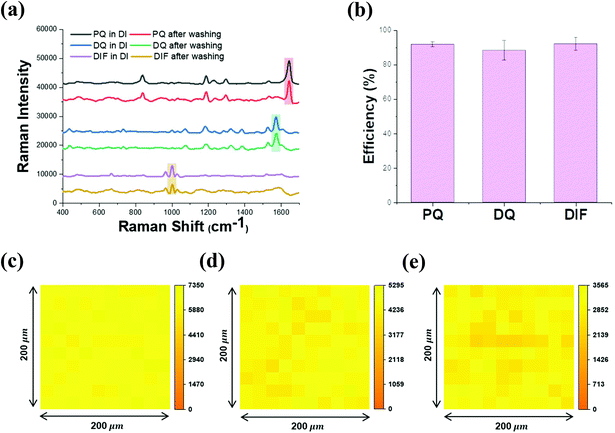 |
| Fig. 4 Selectivity test of PQ, DQ, and DIF. (a) Raman spectra of PQ, DQ, and DIF dissolved in DI water at a concentration of 10 ppm with and without the washing step, (b) pesticide capture efficiency calculated from the Raman intensity in Fig. 4a, and (c) Raman mapping of the CD-AuNSL after washing of PQ, (d) DQ, and (e) DIF. | |
3.4. Selectivity test of the CD-AuNSL using real samples
The selective bipyridylium pesticide detection capability of the CD-AuNSL substrate was tested using a ground-apple solution without any pretreatment such as dilution, extraction, centrifugation and so on. As shown in Fig. 5a, 10 μL of the ground-apple solution spiked with each pesticide (10 ppm) was dropped on the CD-AuNSL for 10 min and then washed with 10 mL of fresh DI water for 1 min. Additionally, the samples prepared without the washing step were used as controls. As shown in Fig. 5b, unlike the spectra obtained for the samples prepared without washing, the Raman spectra of PQ, DQ, and DIF were clearly observed after the washing step. The enhanced signal intensity can be explained by the removal of the apple-matrix substances during washing. Photographs of the CD-AuNSL treated with the apple matrix with and without washing are shown in Fig. S6a.† The CD-AuNSL samples prepared without washing showed a brownish color that originated from the Maillard reaction of the apple matrix, while the CD-AuNSL sample prepared with washing maintained a gold color like the substrate before the apple solution treatment. It is considered that the apple matrix covered on the CD-AuNSL surface attenuated laser penetration to the hot-spots, reducing the Raman signal. As controls, the bare AuNSL substrate was tested using the apple-ground solutions spiked with PQ, DQ, and DIF at 50 ppm concentrations. Even though the concentration of each pesticide was higher than that of the pesticides used in the experiment with the CD-AuNSL (10 ppm), the Raman intensity was significantly decreased for both of the samples with and without the washing step due to the presence of interfering apple matrix molecules that competitively adhere on the substrate (Fig. S6b†). The capture efficiencies of the CD-AuNSL for each pesticide spiked in the apple matrix solution with and without washing were compared and the results are presented in Fig. 5c. The Raman intensity of each pesticide (in DI, 10 ppm) dropped on the CD-AuNSL without washing was used as the control (described as 100% in the bar graph). PQ, DQ, and DIF spiked in the apple matrix solution with the washing step showed capture efficiency values of 44.1%, 63.6%, and 43.7% compared to the Raman intensity of the controls, respectively, while the samples without washing exhibited a capture efficiency of less than 16%. The competitive binding and hindrance of pesticides for CD molecules in the presence of the apple matrix might result in a capture efficiency that is less than that in DI water, but the resulting capture efficiency was significantly higher than that of the pesticide treated CD-AuNSL sample prepared without washing or the tests performed on the bare AuNSL. Then, the detection limit of the CD-AuNSL for the pesticides in the ground-apple solution was tested and is shown in Fig. 5d–f. Each pesticide with a volume of 10 μL was dropped on the CD-AuNSL substrate for 10 min in a concentration range of 50 ppm to 0.05 ppm followed by the washing and Raman measurement. As a result, PQ, DQ, and DIF were detected down to a concentration of 0.05 ppm. Interestingly, each pesticide with a concentration above 5 ppm showed no further increase of the Raman intensity. It can be considered that the inclusion sites of the surface-functionalized CD on the AuNSL were saturated after the reaction and washing step, especially in the presence of the apple matrix. In a concentration range of 5 ppm–0.05 ppm, the Raman intensity was linearly correlated with logarithmic concentrations of each pesticide with an R2 value of ∼ 0.99 (Fig. S7a–c†). On the other hand, the sensitivity needs to be considered together with the sample volume because the number of molecules on the substrate is dependent on the drop volume after drying of the sample. In this work, all the samples with a volume of 10 μL were dropped on the substrate and then measured using a portable Raman instrument after drying. The detection limit can be possibly improved by using a higher sample volume or using the Raman instrument with a better detection resolution. However, because the developed method is not concerned with pretreatment methods, especially dilution, the sensitivity of the CD-AuNSL is sufficient for practical applications, considering a maximum residue limit (MRL) value of 0.05 ppm for bipyridylium pesticides.38,39 On the other hand, the Raman spectra of the CD-AuNSL and bare AuNSL before and after washing with DI water were compared in Fig. S8a.† In addition, both the substrates treated with the pure apple matrix solution before and after the washing step are also presented in Fig. S8b.† Commonly, a hill-like background Raman shift in a region of 1500–1650 cm−1 was observed after washing, but the intensity did not significantly interfere with the target measurement. Furthermore, in the apple matrix solution, substances that generate background signals were not observed. However, in the case of matrices that contain pigment substances, the washing step should be involved during the assay to reduce the potential background. Then, to test the mixture component detection capability of the CD-AuNSL, it was studied using binary and ternary mixtures of PQ, DQ, and DIF. The final concentration of each pesticide in the ground-apple solution was fixed at 10 ppm and then washed after dropping of 10 μL samples on the CD-AuNSL substrate for 10 min. Because each pesticide exhibits its own Raman fingerprint spectrum, the mixed components could be identified as shown in Fig. 5g. To further differentiate the mixtures, principal component analysis (PCA) was carried out using the single and mixture spectra of PQ, DQ, and DIF (10 observations for each group). The PCA plot clearly shows the separated groups for identification as shown in Fig. 5h. These data indicated that the mixture targets could be captured simultaneously by CD-AuNSL, but each component is possibly discriminated by simple PCA. CD is known to form an inclusion complex with various molecules, such as endocrine disrupting chemicals, drugs, toxic compounds and so on.19,23,40–42 Therefore, combining the advantages of the selective and sensitive detection properties of the CD-AuNSL with those of the label-free analysis of Raman, the developed CD-AuNSL substrate can be further applied for various purposes by expanding the number of targets. From all the results, the CD-AuNSL substrate is found to be feasible for selective and label-free bipyridylium pesticide detection with a pretreatment-free procedure. The developed SERS substrate is expected to be applied for various molecular detection sensors, especially for real samples that contain complicated matrices, such as environmental, biological, and agricultural products.
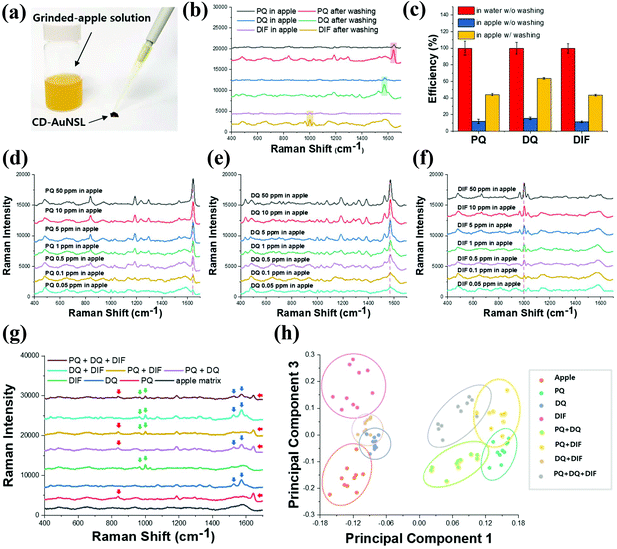 |
| Fig. 5 Selectivity test of the CD-AuNSL using the ground-apple solution spiked with pesticides. (a) Photograph of CD-AuNSL treatment with the pesticide-spiked ground-apple solution, (b) Raman spectra of PQ, DQ, and DIF before and after the washing step, (c) capture efficiency comparison of the CD-AuNSL for each pesticide spiked in DI water and the ground-apple solution with and without washing, (d) Raman spectra of PQ, (e) DQ and (f) DIF spiked at various concentrations in the ground-apple solution after washing, (g) Raman spectra of the binary and ternary mixtures of PQ, DQ, and DIF, and (h) PCA scatter plot of the single, binary, and ternary mixtures of PQ, DQ, and DIF. | |
4. Conclusion
In summary, a CD-functionalized AuNSL substrate was developed for selective and label-free bipyridylium pesticide detection. The CD-AuNSL showed a sufficient detection capability for PQ, DQ, and DIF, with a detection limit down to a concentration of 0.05 ppm which is a MRL value for bipyridylium pesticides. After the demonstration of the selectivity for the pesticides spiked in DI water, the substrate was tested using a ground-apple solution for practical applications. After simple dropwise addition for 10 min and washing for 1 min, each PQ, DQ, and DIF pesticide was detected down to a concentration of 0.05 ppm. Then, binary and ternary mixtures of the three pesticides were tested and PCA clearly differentiated the mixture components. Based on the results, the developed CD-AuNSL substrate is expected to be applied to various molecular detection sensors with high selectivity and sensitivity for use in a label-free identification method.
Conflicts of interest
The authors declare no competing financial interest.
Acknowledgements
This work was supported by the Fundamental Research Program (PNK6800) of the Korea Institute of Materials Science (KIMS) and the Ministry of Trade, Industry and Energy (Grant N0002310).
References
- J. N. Anker, P. Hall, O. Lyandres, N. C. Shah, J. Zhao and R. P. Van Duyne, Biosensing, with Plasmonic Nanosensors, Nat. Mater., 2008, 7, 442–453 CrossRef CAS.
- Y. C. Cao, R. Jin and C. A. Mirkin, Nanoparticles with Raman Spectroscopic Fingerprints for Dna and Rna Detection, Science, 2002, 297, 1536–1540 CrossRef CAS.
- K. Wang, D.-W. Sun, H. Pu, Q. Wei and L. Huang, Stable, Flexible, and High-Performance SERS Chip Enabled by a Ternary Film-Packaged Plasmonic Nanoparticle Array, ACS Appl. Mater. Interfaces, 2019, 11, 29177–29186 CrossRef CAS.
- J. W. Jeong, M. M. P. Amob, K.-M. Baek, S. Y. Lee, W.-C. Shih and Y. S. Jung, 3D Cross-Point Plasmonic Nanoarchitectures Containing Dense and Regular Hot Spots for Surface-Enhanced Raman Spectroscopy Analysis, Adv. Funct. Mater., 2016, 28, 8695–8704 CrossRef CAS.
- H. S. Jung, E. H. Koh, C. W. Mun, J. H. Min, W. Sohng, H. Chung, J.-Y. Yang, S. Lee, H. J. Kim, S.-G. Park, M.-Y. Lee and D.-H. Kim, Hydrophobic hBN-coated Surface-enhanced Raman Scattering Sponge Sensor for Simultaneous Separation and Detection of Organic pollutants, J. Mater. Chem. C, 2019, 7, 13059 RSC.
- X. Kong, Y. Xi, P. L. Duff, X. Chong, E. Li, F. Ren, G. L. Rorrer and A. X. Wang, Detecting Explosive Molecules from Nanoliter Solution: A New Paradigm of SERS Sensing on Hydrophilic Photonic Crystal Biosilica, Biosens. Bioelectron., 2017, 88, 63–70 CrossRef CAS.
- F. Sun, H.-C. Hung, A. Sinclair, P. Zhang, T. Bai, D. D. Galvan, P. Jain, B. Li, S. Jiang and Q. Yu, Hierarchical Zwitterionic Modification of a SERS Substrate Enables Real-time Drug Monitoring in Blood Plasma, Nat. Commun., 2016, 7, 13437 CrossRef CAS.
- S. Hu, Y. Gao, Y. Wu, X. Guo, Y. Ying, Y. Wen and H. Yang, Raman Tracking the Activity of Urease in Saliva for Healthcare, Biosens. Bioelectron., 2019, 129, 24–28 CrossRef CAS.
- B. Murugesan and J. Yang, Tunable Coffee Ring Formation on Polycarbonate Nanofiber Film for Sensitive SERS Detection of Phenylalanine in Urine, ACS Omega, 2019, 4, 14928–14936 CrossRef CAS.
- M. P. Cecchini, V. A. Turek, J. Paget, A. A. Kornyshev and J. B. Edel, Self-assembled Nanoparticle Arrays for Multiphase Trace Analyte Detection, Nat. Mater., 2013, 12, 165–171 CrossRef CAS.
- H. Wu, Y. Luo, C. Hou, D. Huo, W. Wang, J. Zhao and Y. Lei, Rapid and Fingerprinted Monitoring of Pesticide Methyl Parathion on the Surface of Fruits/Leaves as well as in Surface Water Enabled by Gold Nanorods Based Casting-and-sensing SERS Platform, Talanta, 2019, 200, 84–90 CrossRef CAS.
- Y. Zhu, J. Wu, H. Gao, G. Liu, Z. Tian, J. Feng, L. Guo and J. Xie, Rapid On-site Detection of Paraquat in Biologic Fluids by Iodide-facilitated Pinhole Shell-isolated Nanoparticle-enhanced Raman Spectroscopy, RSC Adv., 2016, 6, 59919 RSC.
- G. P. Szekeres and J. Kneipp, Different Binding Sites of Serum Albumins in the Protein Corona of Gold Nanoparticles, Analyst, 2018, 143, 6061 RSC.
- P. Muhammad, S. Hanif, J. Yan, F. U. Rehman, J. Wang, M. Khan, R. Chung, A. Lee, M. Zheng, Y. Wang and B. Shi, SERS-based Nanostrategy for Rapid Anemia Diagnosis, Nanoscale, 2020, 12, 1948 RSC.
- Y. Chen, Y. Zhang, F. Pan, J. Liu, K. Wang, C. Zhang, S. Cheng, L. Lu, W. Zhang, Z. Zhang, X. Zhi, Q. Zhang, G. Alfranca, J. M. de la Fuente, D. Chen and D. Cui, Breath Analysis Based on Surface-enhanced Raman Scattering Sensors Distinguishes Early and Advanced Gastric Cancer Patients from Healthy Persons, ACS Nano, 2016, 10, 8169–8179 CrossRef CAS.
- S. S. Singha, S. Mandal, T. S. Bhattacharya, L. Das, K. Sen, B. Satpati, K. Das and A. Singha, Au Nanoparticles Functionalized 3D-MoS2 Nanoflower: An Efficient SERS Matrix for Biomolecule Sensing, Biosens. Bioelectron., 2018, 119, 10–17 CrossRef CAS.
- V. Marti-Centelles, M. D. Pandey, M. I. Burguete and S. V. Luis, Macrocyclization Reactions: The Importance of Conformational, Configurational, and Template-Induced Preorganization, Chem. Rev., 2015, 115, 8736–8834 CrossRef CAS.
- H. Cong, X. L. Ni, X. Xiao, Y. Huang, Q.-J. Zhu, S.-F. Xue, Z. Tao, L. F. Lindoy and G. Wei, Synthesis and Separation of Cucurbit[n]urils and Their Derivatives, Org. Biomol. Chem., 2016, 14, 4335–4364 RSC.
- Q.-D. Hu, G.-P. Tang and P. K. Chu, Cyclodextrin-Based Host-Guest Supramolecular Nanoparticles for Delivery: From Design to Applications, Acc. Chem. Res., 2014, 47, 2017–2025 CrossRef CAS.
- D. A. Jency, M. Umadevi and G. V. Sathe, SERS Detection of Polychlorinated Biphenyls using β-cyclodextrin Functionalized Gold Nanoparticles on Agriculture Land Soil, J. Raman Spectrosc., 2015, 46, 377–383 CrossRef.
- J. Li, X. Hu, Y. Zhou, L. Zhang, Z. Ge, X. Wang and W. Xu, β-Cyclodextrin-Stabilized Au Nanoparticles for the Detection of Butyl Benzyl Phthalate, ACS Appl. Nano Mater., 2019, 2, 2743–2751 CrossRef CAS.
- L. Yang, Y. Chen, H. Li, L. Luo, Y. Zhao, H. Zhang and Y. Tian, Application of Silver Nanoparticles Decorated with b-cyclodextrin in Determination of 6-mercaptopurine by Surface-enhanced Raman Spectroscopy, Anal. Methods, 2015, 7, 6520–6527 RSC.
- C. Fang, N. M. Bandaru, A. V. Ellis and N. H. Veolcker, Beta-cyclodextrin Decorated Nanostructured SERS Substrates Facilitate Selective Detection of Endocrine Disruptor Chemicals, Biosens. Bioelectron., 2013, 42, 632–639 CrossRef CAS.
- Q. Zhou, A. K. Thokchom, D.-J. Kim and T. Kim, Inkjet-Printed Ag Micro-/Nanostructure Clusters on Cu Substrates for In-Situ Pre-concentration and Surface-enhanced Raman Scattering, Sens. Actuators, B, 2017, 243, 176–183 CrossRef CAS.
- B. Chen, G. Meng, F. Zhou, Q. Huang, C. Zhu, X. Hu and M. Kong, Ordered Arrays of Au-Nanobowls Loaded with Ag-Nanoparticles as Effective SERS Substrates for Rapid Detection of PCBs, Nanotechnology, 2014, 25, 145605 CrossRef.
- S.-G. Park, X. Xiao, J. Min, C. W. Mun, H. S. Jung, V. Giannini, R. Weissleder, S. A. Maier, H. Im and D.-H. Kim, Self-Assembly of Nanoparticle-Spiked Pillar Arrays for Plasmonic Biosensing, Adv. Funct. Mater., 2019, 29, 1904257 CrossRef CAS.
- P. H. C. Eilers, A Perfect Smoother, Anal. Chem., 2003, 75, 3631–3636 CrossRef CAS.
- E. H. Koh, C. W. Mun, C. Kim, S.-G. Park, E. J. Choi, S. H. Kim, J. Dang, J. Choo, J.-W. Oh, D.-H. Kim and H. S. Jung, M13 Bacteriophage/Silver Nanowire Surface-Enhanced Raman Scattering Sensor for Sensitive and Selective Pesticide Detection, ACS Appl. Mater. Interfaces, 2018, 10, 10388–10397 CrossRef CAS.
- Q. Ye, J. Fang and L. Sun, Surface-Enhanced Raman Scattering from Functionalized Self-Assembled Monolayers. 2. Distance Dependence of Enhanced Raman Scattering from
an Azobenzene Terminal Group, J. Phys. Chem., 1997, 101, 8221–8224 CrossRef CAS.
- H. L. Tcheumi, V. N. Tassontio, I. G. Tonle and E. Ngameni, Surface Functionalization of Smectite-type Clay by Facile Polymerization of β-cyclodextrin Using Citric Acid Cross Linker: Application as Sensing Material for the Electrochemical Determination of Paraquat, Appl. Clay Sci., 2019, 173, 97–106 CrossRef CAS.
- C. Liu, P. Wang, Z. Shen, X. Liu, Z. Zhou and D. Liu, pH-Controlled Quaternary Ammonium Herbicides Capture/Release by Carboxymethyl-b-Cyclodextrin Functionalized Magnetic Adsorbents: Mechanisms and Application, Anal. Chim. Acta, 2015, 901, 51–58 CrossRef CAS.
- C. Liu, P. Wang, X. Liu, X. Yi, Z. Zhou and D. Liu, Supramolecular Fluorescent Sensor Array for Simultaneous Qualitative and Quantitative Analysis of Quaternary Ammonium Herbicides, New J. Chem., 2018, 42, 17317–17322 RSC.
- L. Pospíšil, J. Hanzlík, R. Fuoco and M. P. Colombini, Electrochemical and Spectral Evidence of the Inclusion of the Herbicide Difenzoquat by Cyclodextrins in Aqueous Solution, J. Electroanal. Chem., 1994, 368, 149–154 CrossRef.
- S. K. S. Al-Burtomani and F. O. Suliman, Inclusion Complexes of Norepinephrine with b-Cyclodextrin, 18-crown-6 and Cucurbit[7]uril: Experimental and Molecular Dynamics Study, RSC Adv., 2017, 7, 9888–9901 RSC.
- N. Rajendiran and S. Siva, Inclusion Complex of Sulfadimethoxine with Cyclodextrins: Preparation and Characterization, Carbohydr. Polym., 2014, 101, 828–836 CrossRef CAS.
- R. Elshafey, M. Siaj and M. Zourob, DNA Aptamers Selection and Characterization for Development of Label-free Impedimetric Aptasensor for Neurotoxin Anatoxin-a, Biosens. Bioelectron., 2015, 68, 295–302 CrossRef CAS.
- R. Elshafey and A.-E. Radi, Electrochemical Impedance Sensor for Herbicide Alachlor Based on Imprinted Polymer Receptor, J. Electroanal. Chem., 2018, 813, 171–177 CrossRef CAS.
- V. T. N. Linh, J. Moon, C. W. Mun, V. Devaraj, J.-W. Oh, S.-G. Park, D.-H. Kim, J. Choo, Y.-I. Lee and H. S. Jung, A Facile Low-Cost Paper-Based SERS Substrate for Label-Free Molecular Detection, Sens. Actuators, B, 2019, 291, 369–377 CrossRef CAS.
- M. C. Carneiro, L. Puignou and M. T. Galceran, Comparison of Silica and Porous Graphitic Carbon as Solid-Phase Extraction Materials for the Analysis of Cationic Herbicides in Water by Liquid Chromatography and Capillary Electrophoresis, Anal. Chim. Acta, 2000, 408, 2363–2269 CrossRef.
- J. Wankar, N. G. Kotla, S. Gera, S. Rasala, A. Pandit and Y. A. Rochev, Recent Advances in Host-Guest Self-Assembled Cyclodextrin Carriers: Implications for Responsive Drug Delivery and Biomedical Engineering, Adv. Funct. Mater., 2020, 1909049 CrossRef CAS.
- Y. Lu, G. Yao, K. Sun and Q. Huang, b-Cyclodextrin Coated SiO2@Au@Ag Core–Shell Nanoparticles for SERS Detection of PCBs, Phys. Chem. Chem. Phys., 2015, 17, 21149–21157 RSC.
- D. M. Alzate-Sanchez, B. J. Smith, A. Alsbaiee, J. P. Hinestroza and W. R. Dichtel, Cotton Fabric Functionalized with a β-Cyclodextrin Polymer Captures Organic Pollutants from Contaminated Air and Water, Chem. Mater., 2016, 28, 8340–8346 CrossRef CAS.
Footnote |
† Electronic supplementary information (ESI) available. See DOI: 10.1039/d0an01703e |
|
This journal is © The Royal Society of Chemistry 2021 |
Click here to see how this site uses Cookies. View our privacy policy here.