Hypoxia-augmented and photothermally-enhanced ferroptotic therapy with high specificity and efficiency†
Received
14th October 2019
, Accepted 12th November 2019
First published on 13th November 2019
Abstract
The rigorous reaction conditions (sufficient H2O2 and a low pH value) of an efficient Fenton reaction limit its further biomedical translation. Therefore, it is urgent to improve the efficacy of the Fenton reaction at the tumor site for efficient ferroptotic therapy. Herein, a hypoxia-responsive-Azo–BSA functionalized biomimetic nanoreactor (Fe(III)-GA/GOx@ZIF-Azo), encapsulating ultrasmall ferric-gallic acid coordination polymer nanoparticles (Fe(III)-GA) and glucose oxidase (GOx) into a zeolitic imidazolate framework (ZIF), was constructed for tumor ablation through an intensive Fenton reaction accelerated by not only sustained Fe2+ and H2O2 supply but also low pH and photothermal stimulation. Moreover, Azo achieved charge reversal in a hypoxia microenvironment caused by the sustained oxygen consumption by GOx, which resulted in selective and enhanced tumor accumulation based on the hypoxia-activated positive feedback cellular uptake. This rationally designed biomimetic nanoreactor might lay a foundation for the clinical translation of ferroptotic therapy.
Introduction
As an interesting alternative to traditional therapeutic modalities, chemodynamic therapy (CDT) has aroused intensive interest as a tumor-specific method to induce cell death without harming normal tissues.1,2 Typically, ferroptosis was characterized by a Fenton reaction employing iron ions to decompose intracellular H2O2 to produce hydroxyl radicals (˙OH),3,4 the most toxic reactive oxygen species (ROS).5,6 Owing to the higher H2O2 level in the tumor microenvironment (TME) than normal tissues, the tumor-specific in situ generation of ROS could greatly enhance the therapeutic specificity and efficiency.7,8 However, substantially caused by the limited catalytic efficiency of ferrous materials and the low endogenous H2O2 level, the unsatisfactory formation of ROS makes it difficult for the biomedical translation of ferroptotic therapy.9–12 In particular, an effective Fenton reaction needs stringent reaction conditions (pH between 3 and 4), and the slightly acidic TME is insufficient to trigger Fenton reaction-generated ROS effectively.13,14 Therefore, how to achieve a highly efficient Fenton reaction at tumor sites is extremely critical for further development of ferroptotic therapy.
One of the common strategies to enhance the efficiency of the Fenton reaction is to accelerate Fe3+/Fe2+ conversion,15 because Fe2+ can easily be oxidized to Fe3+, which exhibits distinctly a lower catalytic performance than Fe2+.16,17 Exactly, some reductants,18 such as gallic acid, present the peculiarity of the acidity-activated reduction of Fe3+ to Fe2+, and hence the incorporation of Fe3+ and GA could trigger continuous Fe2+ supply under acidic conditions. Intriguingly, in the latest studies, ultrasmall Fe(III)-gallic acid (GA) coordination polymer nanoparticles (Fe(III)-GA) with strong near-infrared light (NIR) absorbance have been introduced in various anticancer strategies,19,20 which have the potential to serve as a biocompatible Fenton catalyst. Importantly, the photothermal effects of Fe(III)-GA could facilitate the ionization process of the Fenton reaction to enhance the catalytic activity of Fe2+.21,22 In this way, the catalytic efficiency of iron ions will be significantly improved.
Glucose oxidase (GOx), oxidizing oxygen and glucose into H2O2 and gluconic acid,23,24 has been widely used for H2O2 supply in the Fenton reaction.25,26 Meanwhile, the produced gluconic acid could lower the pH to meet the strict conditions (pH 3–4) of the Fenton reaction,27–29 thereby improving the efficiency of the Fenton reaction.30 Furthermore, the sustained oxygen consumption by GOx will generate a severe hypoxia microenvironment,31 which partly goes against increasing the intracellular H2O2 level. Conversely, it could convert the negative effects of hypoxia into a positive factor by constructing a nanocarrier changeable surface property. It is known that compounds containing an azobenzene group can be specifically reduced by bioreductases under tumor hypoxia.32–36 Thus, grafting 4,4′-azonzenecarboxylic acid (Azo) on the surface of the nanocarriers could cause hypoxia-activated charge reversal from negative to positive for facilitating the cellular uptake and tumor accumulation.
Along this line, we report an Azo–BSA-functionalized hybrid zeolitic imidazolate framework (ZIF) biomimetic nanoreactor encapsulating Fe(III)-GA and GOx (Fe(III)-GA/GOx@ZIF-Azo, FGGZA) for hypoxia-activated positive feedback cellular uptake and significantly efficient ferroptotic therapy (Scheme 1). FGGZA modulates the Fenton reaction environment by accelerating Fe3+/Fe2+ conversion, lowering the pH, increasing the H2O2 level and introducing heat stimulation to generate abundant ROS for cell death via the ferroptosis pathway. Meanwhile, the repeated mutual promotion of oxygen consumption by GOx and hypoxia-enhanced cellular uptake endowed the nanoreactor with a unique effect of hypoxia-activated positive feedback cellular uptake, which will greatly improve the cell killing efficacy of FGGZA. The rationally designed FGGZA integrates the efficiency, selectivity and biocompatibility for ferroptotic therapy, and will lay the foundation for the clinical translation of ferroptosis.
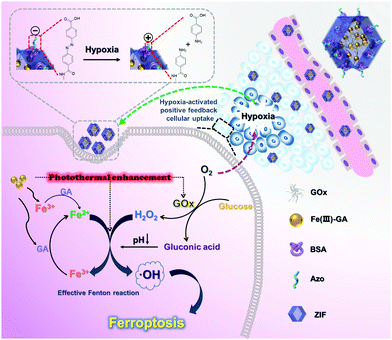 |
| Scheme 1 Schematic illustration of FGGZA as an efficient ferroptosis-inducing nanoagent for tumor elimination and detailed processes of promotion of Fe3+/Fe2+ conversion, self-supplied H2O2, low pH and the photothermal stimulation accelerated Fenton reaction and the self-generated hypoxia environment for enhanced ferroptotic therapy. | |
Results and discussion
Characterization of FGGZA
Firstly, Fe(III)-GA coordination polymer nanoparticles were assembled via strong coordination between ferric ions (Fe3+) and polyphenol groups of GA. When GA was added into a mixed solution of Fe3+ and polyvinylpyrrolidone (PVP) at a mass feeding ratio of 5
:
10
:
33 (GA/Fe/PVP), the color of the solution immediately changed from colorless to dark. The transmission electron microscopy (TEM) image (Fig. 1a) showed disperse ultrasmall nanoparticles with a diameter of ∼3 nm. Moreover, the mass ratio of Fe3+ in the Fe(III)-GA was measured to be 3.6% by ICP. Then, Fe(III)-GA and GOx were encapsulated into a ZIF for the effective ROS-producing nanoplatform. The images of TEM and scanning electron microscopy (SEM) (Fig. 1b and g) both showed a regular orthohexagonal morphology with a diameter of ∼110 nm. To prove the successful package of Fe(III)-GA into the ZIF, the corresponding elemental mapping of various elements was conducted to indicate the clear distribution of C, N, O, Fe, and Zn. And the UV-Vis spectra of FGGZ (Fig. 1d and Fig. S1, ESI†) showed the characteristic absorption peak of GOx, suggesting the successful encapsulation of GOx into the ZIF. Furthermore, thermogravimetric analysis (TGA) (Fig. 1f) reconfirmed the package of GOx into FGGZ with 1% loading, and the iron content in FGGZ was ∼0.8% as determined by ICP. In particular, the X-ray diffraction pattern of FGGZ (Fig. 1e) showed that encapsulation did not alter the crystal structure of the ZIF, ignoring the slightly reduced crystallinity.
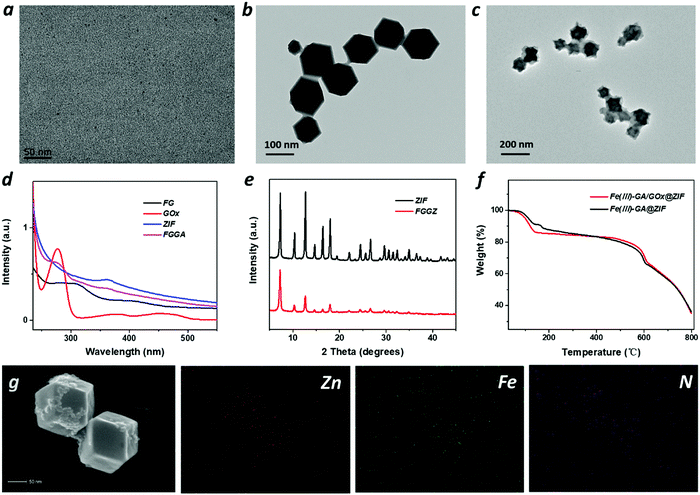 |
| Fig. 1 Characterization of FG, FGGZ and FGGZA. (a) TEM image of Fe(III)-GA, (b) FGGZ and (c) FGGZA. (d) FTIR spectra of Fe(III)-GA, GOx and FGGZ. (e) XRD patterns of ZIF and FGGZ. (f) TGA of Fe(III)-GA/GOx@ZIF and Fe(III)-GA@ZIF. (g) Corresponding elemental mapping of FGGZ. | |
To endow FGGZ with hypoxia-responsive capability, Azo was modified on the surface of FGGZ. Azo was synthesized according to a previous report,37 and Azo–BSA was synthesized by the reaction between the carboxyl group and the amino group. As shown in Fig. S2 (ESI†), the UV-Vis spectra of Azo–BSA showed the characteristic absorption peak of both Azo and BSA, which demonstrated the successful synthesis of Azo–BSA. Furthermore, the prepared Azo–BSA was modified on the surface of FGGZ via electrostatic incorporation, which was verified by the TEM image in Fig. 1c. Moreover, the changes of the zeta potential and the particle size determined using a Malvern (Fig. S3 and S4, ESI†) reconfirmed the successful preparation of FGGZA.
Photothermal performance of Fe(III)-GA and its effect on GOx
The photothermal conversion effect of Fe(III)-GA was explored owing to its absorption in the NIR region.38 The temperature variations in Fe(III)-GA aqueous solutions with different concentrations (0–100 μg mL−1) were detected under 808 nm laser irradiation (1.54 W cm−2) for 10 min. As shown in Fig. 2c and Fig. S5 (ESI†), with the addition of Fe(III)-GA aqueous solution (100 μg mL−1), the temperature increased by 16.3 °C in 10 min, and the photothermal conversion efficiency of Fe(III)-GA was calculated to be 66.5%, signifying that Fe(III)-GA possesses outstanding photothermal performance to be employed as an efficient photothermal agent. Moreover, no obvious temperature increments were found over five cycles of laser on/off (Fig. S6, ESI†), indicating the excellent photostability of Fe(III)-GA.
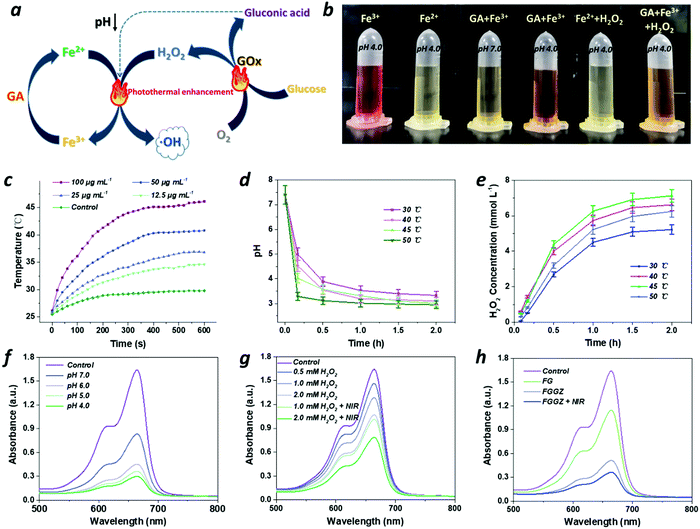 |
| Fig. 2 (a) Schematic illustration of the “all in one” system to efficiently promote the Fenton reaction. (b) Observation of the iron redox cycling by GA under acidic conditions using o-phenanthroline as a Fe2+ indicator. (c) The photothermal performance of Fe(III)-GA irradiated with a 808 nm laser (1.54 W cm−2) for 10 min. (d) The increase of H2O2 concentrations and (e) decrease of pH values after incubation with GOx (0.01 mg mL−1) solution containing glucose (1 mg mL−1) at different time intervals. (f) The degradation of MB at different pHs, (g) at different concentrations of H2O2 and (h) with Fe(III)-GA, FGGZ and FGGZ plus NIR. | |
GOx is co-loaded with Fe(III)-GA into the ZIF nanocarrier to lower the pH and generate more H2O2 for promoting the Fenton reaction. It is necessary to assess the catalytic efficiency of GOx under different conditions by monitoring the amount of generated gluconic acid (reflected by the pH value) and H2O2. As shown in Fig. 2d, e and Fig. S7 (ESI†), the acid and H2O2 yields quickly increased along with time, indicating GOx an efficient catalyst that converts glucose and oxygen into gluconic acid and H2O2. Moreover, the catalytic activity markedly increased as the temperature increases, ignoring the unusual generated H2O2 concentrations at 50 °C possibly due to the pyrolysis of H2O2. Hence, the photothermal conversion effect of Fe(III)-GA could elevate the catalytic activity of GOx for the efficient Fenton reaction.
ROS generation by the Fenton reaction
Fe2+ exhibits preferable catalytic activity compared to Fe3+ for the Fenton reaction, while a reductive agent could be beneficial for markedly elevating the conversion rate from Fe3+ to Fe2+.18 To validate the acidity-activated reduction of GA against Fe3+, o-phenanthroline was used to indicate Fe2+ with a color of red while Fe3+ with achromaticity. As shown in Fig. 2b, with the addition of GA, the color of the Fe3+ solution changed from pale yellow to orange at pH 4.0 rather than 7.0, indicating that Fe3+ could be reduced to Fe2+ under acidic conditions. After adding H2O2 to start the Fe2+-mediated Fenton reaction, the solution without GA instantly turned colorless due to the reaction conversion from Fe2+ to Fe3+, while the GA-containing solution remained orange, implying that GA could be employed to timely convert Fe3+ into Fe2+ in the Fenton reaction in an acidic environment. However, the inadequate acidity in the tumor microenvironment presents a new obstacle against the efficient Fenton reaction. Exactly, the product gluconic acid of GOx catalysis could afford acidic conditions for the above reduction reaction (Fig. 2d). For GOx, it was already certified to oxidize oxygen and glucose into H2O2 and gluconic acid, which significantly accelerated the Fenton reaction not only by lowering the pH but also by increasing the H2O2 level. To verify this deduction, methylene blue (MB), which can be degraded by ˙OH to decrease its absorbance at 665 nm, was employed as the indicator of ˙OH generation. As shown in Fig. 2f, MB greatly degraded as the pH decreases, indicating that the efficiency of the Fenton reaction relies heavily on the acidic conditions. Additionally, the characteristic absorption peak of MB obviously reduced as the H2O2 concentration increased (Fig. S8, ESI†), suggesting that the Fenton reaction exhibits a H2O2 concentration-dependent manner. Therefore, integrating Fe(III)-GA with GOx could be a versatile scheme to generate sufficient ROS for efficient ferroptotic therapy.
Furthermore, the efficiency of the Fenton reaction could be also improved by the photothermal conversion effect of Fe(III)-GA. As shown in Fig. 2g, when NIR was introduced, the production of ˙OH markedly increased, because the ionization process of the Fenton reaction could be facilitated as the temperature increases.21 Taken together, the group of FGGZ + NIR exhibited higher ˙OH production efficiency than both Fe(III)-GA and FGGZ (Fig. 2h), signifying that FGGZ could serve as a competent ROS generator to induce long-term ferroptosis.
Hypoxia-activated positive feedback cellular uptake
The negatively charged tumor cell membrane imposes a considerable obstacle to the intracellular drug delivery of nanocarriers with a surface negative charge on account of the electrostatic repulsion. Thus, charge reversal of the negatively charged FGGZA assisted by bioreductases under hypoxic conditions was designed to facilitate the cellular uptake by MCF-7 cells. Firstly, the zeta potential changes of FGGZA in the presence of azoreductase NQO1(NADPH-quinoneoxidoreductase) under hypoxia conditions were investigated. Fig. S9 (ESI†) shows the superior reactivity of FGGZA in the presence of NQO1 in a hypoxia environment than that without NQO1, demonstrating the significant role of azoreductases in the charge reversal of FGGZA. Hypoxia caused by FGGZA was observed using ROS-ID as an indicator, and the results indicated that the consumption of O2 by FGGZA could result in a hypoxia environment (Fig. 3f). To explore the outstanding internalization of FGGZA under hypoxia conditions, the intracellular Fe content was analyzed by ICP to reflect the amount of endocytosed FGGZA. As shown in Fig. 3d, the Azo–BSA-functioned FGGZ led to an obvious higher amount of accumulated Fe in the cells under hypoxic conditions, which could be attributed to the hypoxia-activated surface positive charge of FGGZA. Moreover, the hypoxia-enhanced internalization of FGGZA was studied under sealed conditions at different incubation times (2, 4 and 6 h) by flow cytometry (FCM). The results showed that the mean fluorescence intensity (MFI) at 6 h was dramatically higher than that at 4 h (Fig. 3b and c), which was probably due to the hypoxia environment formation because of the sustained oxygen consumption by GOx during the period from 4 to 6 h. As a result, the combination of the cationic behavior of Azo under hypoxia conditions and the sustained oxygen consumption by GOx could achieve the effect of hypoxia-activated positive feedback cellular uptake.
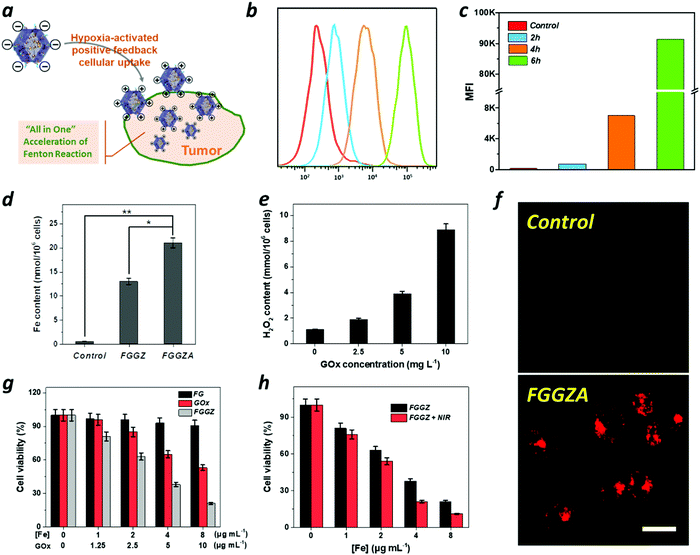 |
| Fig. 3 (a) Schematic illustration of the performance of FGGZA at tumor sites. (b) Cellular uptake of Rhodamine B-loaded FGGZA at different times in vitro and (c) the corresponding MFI values. (d) The Fe content in MCF-7 cells after 6 h of incubation with FGGZ and FGGZA. (e) The H2O2 content in MCF-7 cells after 6 h of incubation at different concentrations of GZ. (f) Intracellular hypoxia of MCF-7 cells using ROS-ID as a hypoxia indicator by CLSM (scale bar: 20 μm). (g) The cell viability of MCF-7 cells after 24 h incubation at different concentrations of Fe(III)-GA, GOx and FGGZ. (h) Cytotoxicity of FGGZ plus NIR to MCF-7 cells compared with that of FGGZ. | |
In vitro anticancer performance
Subsequently, the catalytic performance of FGGZ against MCF-7 cells was investigated. First, the ROS production behavior of Fe2+ was confirmed by utilizing 2′,7′-dichlorofluorescin diacetate (DCFH-DA), an indicator of intracellular ROS levels. As expected, the Fe2+-exposed MCF-7 cells showed stronger green fluorescence of DCF over control cells (Fig. S12, ESI†), indicating the generation of ROS in cells treated with Fe2+, which could be attributed to the occurrence of the Fenton reaction to expand the intracellular oxidative stress. As is known, Fe2+ exhibits considerably higher catalytic activity than Fe3+, whereas the conversion from Fe3+ to Fe2+ is fairly low, making it necessary to introduce a reductive agent to accelerate the Fenton reaction. As shown in Fig. S10 (ESI†), the cytotoxicity of Fe3+ in the presence of GA was distinctly higher than that of Fe3+ towards MCF-7 cells, suggesting that the ROS generation could be promoted by GA to maintain the Fe2+ supply. In addition, the intracellular H2O2 level was also responsible for the amplification of intracellular oxidative stress through the Fenton reaction, as shown in Fig. S11 (ESI†). Thus, the H2O2 content in GOx-treated cells was measured, which indicated a positive correlation with the GOx concentration (Fig. 3e). Notably, the cytotoxicity of FGGZ towards MCF-7 cells was significantly higher than that of neither Fe(III)-GA nor GOx (Fig. 3g) implying that the integration of Fe(III)-GA with GOx to FGGZ presents a potential nanoreactor for an efficient Fenton reaction. Exactly, the viability of MCF-7 cells exposed to FGGZ under NIR irradiation was markedly lower than that exposed to FGGZ without NIR irradiation (Fig. 3h), indicating that the efficiency of the Fenton reaction could be improved by the photothermal effect of Fe(III)-GA. In short, FGGZ could be employed as a competent ROS generator.
Then, the intracellular production of ROS was qualitatively and quantitatively assessed by confocal microscopy (CLSM) and FCM, respectively. As shown in Fig. 4a, c and e, the MCF-7 cells incubated with FGGZ + NIR presented a higher DCF fluorescence intensity and 1.3-fold higher amount of ROS generation than that of no-laser-treated group at the same concentration of Fe, suggesting that FGGZ could serve as a promising agent for efficient ferroptotic therapy. As for the occurrence of ferroptosis, the intact nucleus with a complete structure and shrunken mitochondria with a dense membrane in Fig. 4b and Fig. S13 (ESI†) were consistent with ferroptosis reported previously.39 Another characteristic of ferroptosis is the generation of lipid ROS, which was observed using BODIPY-C11 as a lipid ROS sensor by CLSM. As shown in Fig. 4d, MCF-7 cells treated with FGGZ + NIR exhibited stronger fluorescence (representing more lipid ROS) in the cell membrane than those exposed to other formulations, which further implied the photothermal-enhanced ferroptosis pathway. Therefore, the FGGZ could induce efficient ferroptotic therapy.
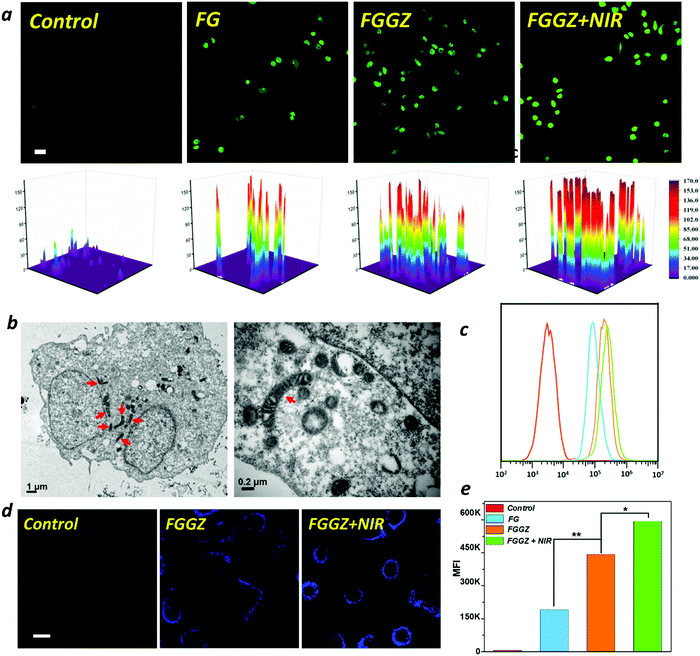 |
| Fig. 4 (a) Qualitatively analysis of generated ROS in MCF-7 cells measured by CLSM. (Scale bar, 40 nm.) (b) TEM image of MCF-7 cells treated with FGGZA plus NIR showing the intact nucleus with a complete structure and shrunken mitochondria with a dense membrane. (c) FCM of ROS production after MCF-7 cells were incubated with Fe(III)-GA, FGGZ and FGGZ plus NIR. (d) Lipid ROS stained with BODIPY-C11 in MCF-7 cells exposed to different treatments (Scale bar: 20 μm.) (e) The corresponding mean fluorescence intensity of cells after different treatments. | |
In vivo biodistribution and photothermal properties
Inspired by the in vitro results, we then investigated the biodistribution of FGGZA containing Rhodamine B with high fluorescence brightness on the MCF-7 tumor-bearing mice model with a tumor volume of 100 mm3. After the intravenous injection of Rhodamine B-loaded FGGZA, the fluorescence intensity in tumor sites gradually increased over time and reached the maximum accumulation at 6 h post-injection (Fig. 5c), which could be ascribed to the negative charge of FGGZA favorable for long circulation in the body escaping from the clearance of the mononuclear phagocyte system (MPS).40,41 The mice were sacrificed 24 h later for the fluorescence imaging of tumor and organs, and the highest fluorescence intensity was demonstrated at tumor sites (Fig. 5d), indicating the selectively and effectively tumor accumulation of FGGZA. The preference to tumor imaging was attributed to the synergism of the hypoxia solid tumor and the hypoxia-activated positive feedback cellular uptake of FGGZA.
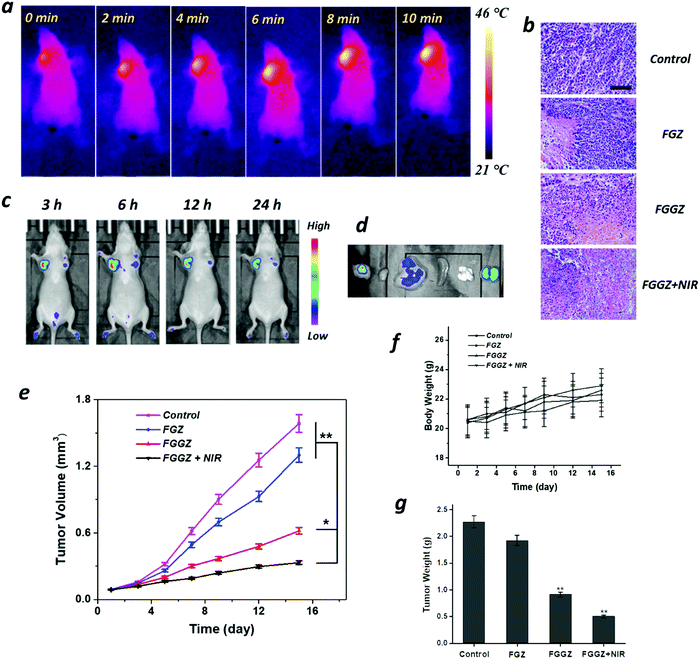 |
| Fig. 5 (a) In vivo photothermal imaging of mice after 6 h post-injection irradiated with an 808 nm laser (1 W cm−2, 10 min). (b) H&E stained images of tumor slides after various treatments (bar = 100 μm). (c) In vivo fluorescence imaging of Rhodamine B-loaded FGGZA at different time points after intravenous injection. (d) Fluorescence imaging of the Rhodamine B-loaded FGGZA in the tumor and major organs at 48 h post-injection. (e) Tumor growth curve of mice with different treatments. (f) Body weights of mice exposed to different formulations among the whole experiment. (g) Average tumor weights of MCF-7 tumor-bearing mice receiving different treatments at the end of the experiment (*p < 0.05 and **p < 0.01). | |
According to the results above, after the intravenous injection of FGGZA at 6 h, NIR was introduced to initiate the photothermal conversion of Fe(III)-GA recorded using a thermal imaging camera. As observed in Fig. 5a, the temperature in tumor sites elevated rapidly from 21 to 46 °C over time, demonstrating the photothermal performance of FGGZA. Additionally, the local low heat could escape from the cytoprotective effect derived by the hyperthermia (>50 °C).42
In vivo anticancer activity
To explore the in vivo therapeutic efficacy of FGGZA, MCF-7 tumor-bearing mice with a tumor volume of 100 mm3 were randomly divided into 4 groups (n = 5) for 2 week observation after different treatments. Various agents (I: PBS control; II: FGZA; III: FGGZA; IV: FGGZA + NIR) were intravenously injected at days 0, 5 and 10 at equivalent doses of Fe (6 mg kg−1). The body weight and tumor size were recorded every two days. It is shown that the treatment of FGGZA + NIR exhibited the most efficient tumor growth inhibition when compared with other groups. Without NIR irradiation, the treatment of FGGZA presented much better anticancer activity than that of FGZA (Fe(III)-GA@ZIF-Azo) (Fig. 5e), which could be ascribed to the increased H2O2 level and the effect of hypoxia-activated positive feedback cellular uptake induced by GOx. According to the weight of tumor tissues excised from the mice (Fig. 5g), the treatment of FGGZA + NIR showed a high tumor inhibition rate of 78%. Furthermore, the cell killing ability of various treatments was assessed by the pathological examination of tumor tissue slices. It was found that the treatment of FGGZA + NIR showed the most severe cell shrinkage and nuclear condensation (Fig. 5b). In contrast, more tumor cells showed intact morphology in other groups. The H&E staining of tumor tissue slices indicated the efficient cell killing activity of FGGZA in line with the in vitro results.
Minimal weight fluctuation was observed among the whole experiment (Fig. 5f). And the major organs, including heart, liver, spleen, kidneys, and lungs, were collected at the end of the treatment for histological examination. In comparison to the PBS control group, the H&E staining of major organs after different treatments showed no obvious pathologic abnormality (Fig. S14, ESI†). The above results demonstrated the low level of systemic toxicity in the FGGZA mediated ferroptotic therapy.
Conclusion
In summary, ferroptosis is of clinical importance due to its escape from the inevitable carriers of traditional tumor treatments. To advance the clinical translation of ferroptotic therapy, a versatile nanosystem was designed and synthesized for tumor elimination via enhanced tumor accumulation and a highly efficient Fenton reaction. Firstly, Azo can respond to a specific hypoxia tumor environment generated by the sustained oxygen consumption by GOx, resulting in a positive surface charge to promote the cellular uptake of FGGZA. Then, in the lysosomal acidic environment, Fe(III)-GA was degraded to Fe3+ and GA, and GA can reduce both the released and the Fenton reaction-generated Fe3+ to Fe2+ for effective ROS production. Meanwhile, GOx can convert oxygen into H2O2 for long-term ferroptosis via continuous ROS generation. Additionally, the photothermal conversion of Fe(III)-GA and the low pH caused by the produced gluconic acid from GOx catalysis can both improve the efficiency of the Fenton reaction, thereby leading to abundant ˙OH production for efficient ferroptotic therapy. All the results indicate that FGGZA has great potential for serving as an efficient ferroptosis-inducing nanoagent. This study presents a good paradigm of effective antitumor treatment to advance the development of ferroptotic therapy.
Experimental section
Materials
Poly(vinylpyrrolidone) (PVP, molecular weight = 8000 g mol−1), ferric chloride (FeCl3), gallic acid (GA), zinc nitrate (ZnNO3), 2-methylimidazole (99%), P-nitrobenzoic acid (98%), glucose (97%), methylene blue (MB, 82%) and hydrogen peroxide (H2O2, 30%) were purchased from Sigma-Aldrich. Glucose oxidase (GOx) was purchased from Shanghai Hepeng Biological Co., Ltd. Fetal bovine serum (FBS) was obtained from Shanghai Ponsure Biotechnology Co., Ltd (Shanghai, China). RPMI-1640 medium was purchased from Gibco Laboratories (NY, USA). 2′,7′-Dichlorodihydrofluorescein diacetate (DCFDA) was purchased from Beyotime Institute of Biotechnology (Nantong, China). All other chemicals were used without further purification.
Synthesis of Fe(III)-gallic acid (GA) nanoparticles
Fe(III)-GA nanoparticles were synthesized by simply mixing the raw materials at a mass ratio of 5
:
10
:
33 (GA/Fe/PVP). Specifically, FeCl3 (0.2 mL, 100 mg mL−1) and PVP (66 mg, 1 mg mL−1) were homogeneously mixed and stirred for 1 h, followed by the addition of the GA solution (1 mL, 10 mg mL−1). After stirring overnight, the mixture was dialysed (MWCO = 25 kDa) against deionized water. The resulting ultrasmall nanoparticles were obtained by freeze-drying.
Synthesis of Fe(III)-GA/GOx@ZIF
First, 7.4 mg of ZnNO3 was dissolved in 10 mL of methanol, followed by the addition of 1 mg of Fe(III)-GA nanoparticles and 0.5 mg of GOx under magnetic stirring. After stirring for 10 min, a 2-methylimidazole methanol solution (2 mL, 2.1 mg mL−1) was added and the mixture was stirred for another 3 h. Finally, the FGGZ nanoparticles were obtained by centrifugation (6000 rpm, 15 min).
Synthesis of Azo–BSA
First, 4,4′-azobenzenecarboxylic acid was synthesized according to a previous report.371H NMR (DMSO-d6): δ 7.99 (d, 4H), δ 8.13 (d, 4H).
Then, to obtain Azo–BSA conjugation, 50 mg of Azo was dissolved in 10 mL DMSO, followed by the addition of 271.7 mg of EDC and 163.1 mg of NHS in 2 mL DMSO. After the addition of 643 μL TEA, the mixture was stirred for 24 h in the dark. Subsequently, a BSA aqueous solution (10 mL, 10 mg mL−1, pH 9.6) was added to the above mix solution, and the mixture was stirred for another 24 h in the dark. Finally, the mixture was dialysed (MWCO = 15 kDa) against deionized water, and the Azo–BSA conjugation was obtained by lyophilization.
Synthesis of Fe(III)-GA/GOx@ZIF-Azo
To modify the Azo–BSA on the surface of FGGZ, the pH of a BSA aqueous solution (5 mL, 0.6 mg mL−1) was adjusted to 9 with NaOH aqueous solution. Next, an FGGZ methanol solution (2 mL, 0.3 mg mL−1) was added to the above solution under magnetic stirring. After reaction for 30 min, the FGGZA nanoparticles were obtained by centrifugation (5500 rpm, 15 min).
Measurement of the catalytic activity of GOx
To assess the catalytic activity of GOx under different temperatures, GOx solutions (10 μg mL−1) containing glucose (1 mg mL−1) were treated with continuous oxygen supply at different temperatures. At desired time points, the samples were collected to measure pH and H2O2 concentrations, which were detected using a pH meter and addition of TiOSO4, respectively. After adding TiOSO4 (0.03 mol L−1) to the samples, the produced yellow complexes with absorbance at 405 nm were measured to determine the H2O2 concentration in comparison with the standard calibration.
˙OH generation by the Fenton reaction
MB solutions (6 μg mL−1) containing 0.5 mM FeCl2 and H2O2 were exposed to different treatments and allowed to incubate for 30 min. The absorbance change of MB at 665 nm was measured.
Cellular uptake assessment
MCF-7 cells seeded in 6-well plates were incubated with RPMI-1640 medium for 24 h. Then the cells were treated with various formulations containing Rhodamine B for desired time durations. Then the cells were collected, followed by the detection of the Fe content by ICP and the intracellular Rhodamine B fluorescence intensity by flow cytometry (FCM).
In vitro cytotoxicity
MCF-7 cells seeded in 96-well plates were incubated in RPMI-1640 medium for 24 h. Then the cells were treated with different formulations with or without NIR irradiation and incubated for another 24 h. Finally, the cell viability was detected by MTT assay.
Reactive oxygen species (ROS) measurements
MCF-7 cells seeded in 6-well plates were incubated in RPMI-1640 medium for 24 h. Then the cells were treated with various formulations for 6 h. Then DCFH-DA was added into the plates, followed by further incubation for 20 min. Subsequently, the intracellular ROS was qualitatively and quantitatively analyzed by confocal laser scanning microscopy (CLSM) and FCM, respectively.
Transmission electron microscopy (TEM) analysis
First, MCF-7 cells seeded in 6-well plates were incubated in RPMI-1640 medium for 24 h. After treatment with FGGZA for 6 h, the cells were collected and fixed with 2.5% glutaraldehyde overnight at 4 °C. After rinsing with phosphate buffer, the sample was dehydrated with a gradient concentration of ethanol, followed by treatment with pure acetone for 20 min. Then, the sample was treated with an embedding agent and acetone, followed by embedding and heated at 70 °C overnight. Finally, after staining with lead citrate solution and uranyl acetate, the section was observed by TEM.
In vivo anticancer activity
MCF-7 tumor-bearing mice with a tumor volume of 100 mm3 were randomly divided into 4 groups (n = 5). Then the mice were intravenously injected with various formulations (I: PBS control; II: FGZA; III: FGGZA; IV: FGGZA + NIR). And the tumor focus was exposed to 808 nm laser irradiation (1.54 W cm−2) for 10 min at 12 h post-injection. The various formulations were intravenously injected at days 0, 5 and 10 at equivalent doses of Fe (6 mg kg−1). The body weight and tumor size were recorded every two days. After the treatments, the mice were sacrificed and the pathological examination of tumor and main organs (heart, spleen, lungs and kidneys) and ultrathin sections were stained by H&E staining.
Statement
All animal experiments in this research work complied with the Regulations on the Administration of Laboratory Animals and the relevant national laws and regulations, and were performed under the guidance approved by the Laboratory Animal Ethics Committee at School of Medicine, Southeast University (Nanjing, China).
Conflicts of interest
The authors declare no conflict of interest.
Acknowledgements
We are highly grateful to the International S&T Cooperation Program of China (No. 2015DFG42240), and acknowledge the financial support from the National Natural Science Foundation of China (Grant No. 21628101, 21905138 and 21371031), the China Postdoctoral Science Foundation (No. 2019M651841) and the Priority Academic Program Development (PAPD) of Jiangsu Higher Education Institutions.
References
- L.-S. Lin, T. Huang, J. Song, X.-Y. Ou, Z. Wang, H. Deng, R. Tian, Y. Liu, J.-F. Wang, Y. Liu, G. Yu, Z. Zhou, S. Wang, G. Niu, H.-H. Yang and X. Chen, Synthesis of Copper Peroxide Nanodots for H2O2 Self-Supplying Chemodynamic Therapy, J. Am. Chem. Soc., 2019, 141, 9937–9945 CrossRef CAS
.
- L. Sun, Y. Xu, Y. Gao, X. Huang, S. Feng, J. Chen, X. Wang, L. Guo, M. Li, X. Meng, J. Zhang, J. Ge, X. An, D. Ding, Y. Luo, Y. Zhang, Q. Jiang and X. Ning, Synergistic Amplification of Oxidative Stress-Mediated Antitumor Activity via Liposomal Dichloroacetic Acid and MOF-Fe2, Small, 2019, 15, e1901156 CrossRef
.
- Z. Shen, T. Liu, Y. Li, J. Lau, Z. Yang, W. Fan, Z. Zhou, C. Shi, C. Ke, V. I. Bregadze, S. K. Mandal, Y. Liu, Z. Li, T. Xue, G. Zhu, J. Munasinghe, G. Niu, A. Wu and X. Chen, Fenton-Reaction-Acceleratable Magnetic Nanoparticles for Ferroptosis Therapy of Orthotopic Brain Tumors, ACS Nano, 2018, 12, 11355–11365 CrossRef CAS
.
- S. Ma, R. F. Dielschneider, E. S. Henson, W. Xiao, T. R. Choquette, A. R. Blankstein, Y. Chen and S. B. Gibson, Ferroptosis and Autophagy Induced Cell Death Occur Independently after Siramesine and Lapatinib Treatment in Breast Cancer Cells, PLoS One, 2017, 12, e0182921 CrossRef
.
- X. Nie, L. Xia, H.-L. Wang, G. Chen, B. Wu, T.-Y. Zeng, C.-Y. Hong, L.-H. Wang and Y.-Z. You, Photothermal Therapy Nanomaterials Boosting Transformation of Fe(III) into Fe(II) in Tumor Cells for Highly Improving Chemodynamic Therapy, ACS Appl. Mater. Interfaces, 2019, 11, 31735–31742 CrossRef CAS
.
- Q. Chen, J. Zhou, Z. Chen, Q. Luo, J. Xu and G. Song, Tumor-Specific Expansion of Oxidative Stress by Glutathione Depletion and Use of a Fenton Nanoagent for Enhanced Chemodynamic Therapy, ACS Appl. Mater. Interfaces, 2019, 11, 30551–30565 CrossRef CAS
.
- X. Wang, X. Zhong, H. Lei, Y. Geng, Q. Zhao, F. Gong, Z. Yang, Z. Dong, Z. Liu and L. Cheng, Hollow Cu2Se Nanozymes for Tumor Photothermal-Catalytic Therapy, Chem. Mater., 2019, 31, 6174–6186 CrossRef CAS
.
- W. Feng, X. Han, R. Wang, X. Gao, P. Hu, W. Yue, Y. Chen and J. Shi, Nanocatalysts-Augmented and Photothermal-Enhanced Tumor-Specific Sequential Nanocatalytic Therapy in Both NIR-I and NIR-II Biowindows, Adv. Mater., 2019, 31, e1805919 Search PubMed
.
- Y. Li, Z. Gao, F. Chen, C. You, H. Wu, K. Sun, P. An, K. Cheng, C. Sun, X. Zhu and B. Sun, Decoration of Cisplatin on 2D Metal-Organic Frameworks for Enhanced Anticancer Effects through Highly Increased Reactive Oxygen Species Generation, ACS Appl. Mater. Interfaces, 2018, 10, 30930–30935 CrossRef CAS
.
- H. Bi, Y. Dai, P. Yang, J. Xu, D. Yang, S. Gai, F. He, B. Liu, C. Zhong, G. An and J. Lin, Glutathione Mediated Size-Tunable UCNPs-Pt(IV)-ZnFe2O4 Nanocomposite for Multiple Bioimaging Guided Synergetic Therapy, Small, 2018, 14, e1703809 CrossRef
.
- Z. Tang, Y. Liu, M. He and W. Bu, Chemodynamic Therapy: Tumour Microenvironment-Mediated Fenton and Fenton-like Reactions, Angew. Chem., Int. Ed., 2019, 58, 946–956 CrossRef CAS
.
- C. You, Z. Gao, H. Wu, K. Sun, L. Ning, F. Lin, B. Sun and F. Wang, Reactive Oxygen Species Mediated Theranostics Using a Fenton Reaction Activable Lipo-polymersome, J. Mater. Chem. B, 2019, 7, 314–323 RSC
.
- B. Ma, S. Wang, F. Liu, S. Zhang, J. Duan, Z. Li, Y. Kong, Y. Sang, H. Liu, W. Bu and L. Li, Self-Assembled Copper-Amino Acid Nanoparticles for in Situ Glutathione “AND” H2O2 Sequentially Triggered Chemodynamic Therapy, J. Am. Chem. Soc., 2019, 141, 849–857 CrossRef CAS
.
- Y. Liu, W. Zhen, L. Jin, S. Zhang, G. Sun, T. Zhang, X. Xu, S. Song, Y. Wang, J. Liu and H. Zhang, All-in-One Theranostic Nanoagent with Enhanced Reactive Oxygen Species Generation and Modulating Tumor Microenvironment Ability for Effective Tumor Eradication, ACS Nano, 2018, 12, 4886–4893 CrossRef CAS
.
- L. Zhang, S.-S. Wan, C.-X. Li, L. Xu, H. Cheng and X.-Z. Zhang, An Adenosine Triphosphate-Responsive Autocatalytic Fenton Nanoparticle for Tumor Ablation with Self-Supplied H2O2 and Acceleration of Fe(III)/Fe(II) Conversion, Nano Lett., 2018, 18, 7609–7618 CrossRef CAS
.
- X. S. Nguyen, G. Zhang and X. Yang, Mesocrystalline Zn-Doped Fe3O4 Hollow Submicrospheres: Formation Mechanism and Enhanced Photo-Fenton Catalytic Performance, ACS Appl. Mater. Interfaces, 2017, 9, 8900–8909 CrossRef CAS
.
- J. Bolobajev, M. Trapido and A. Goi, Interaction of Tannic Acid with Ferric Iron to Assist 2,4,6-trichlorophenol Catalytic Decomposition and Reuse of Ferric Sludge as a Source of Iron Catalyst in Fenton-Based Treatment, Appl. Catal., B, 2016, 187, 75–82 CrossRef CAS
.
- T. Liu, W. Liu, M. Zhang, W. Yu, F. Gao, C. Li, S.-B. Wang, J. Feng and X.-Z. Zhang, Ferrous-Supply-Regeneration Nanoengineering for Cancer-Cell-Specific Ferroptosis in Combination with Imaging-Guided Photodynamic Therapy, ACS Nano, 2018, 12, 12181–12192 CrossRef CAS
.
- F. Liu, X. He, H. Chen, J. Zhang, H. Zhang and Z. Wang, Gram-Scale Synthesis of Coordination Polymer Nanodots with Renal Clearance Properties for Cancer Theranostic Applications, Nat. Commun., 2015, 6, 8003 CrossRef CAS
.
- J. Zeng, M. Cheng, Y. Wang, L. Wen, L. Chen, Z. Li, Y. Wu, M. Gao and Z. Chai, pH-Responsive Fe(III)-Gallic Acid Nanoparticles for In Vivo Photoacoustic-Imaging-Guided Photothermal Therapy, Adv. Healthcare Mater., 2016, 5, 772–780 CrossRef CAS
.
- Q. Chen, Y. Luo, W. Du, Z. Liu, S. Zhang, J. Yang, H. Yao, T. Liu, M. Ma and H. Chen, Clearable Theranostic Platform with a pH-Independent Chemodynamic Therapy Enhancement Strategy for Synergetic Photothermal Tumor Therapy, ACS Appl. Mater. Interfaces, 2019, 11, 18133–18144 CrossRef CAS
.
- Y. Liu, W. Zhen, Y. Wang, J. Liu, L. Jin, T. Zhang, S. Zhang, Y. Zhao, S. Song, C. Li, J. Zhu, Y. Yang and H. Zhang, One-Dimensional Fe2 P Acts as a Fenton Agent in Response to NIR II Light and Ultrasound for Deep Tumor Synergetic Theranostics, Angew. Chem., Int. Ed., 2019, 58, 2407–2412 CrossRef CAS
.
- W. Ke, J. Li, F. Mohammed, Y. Wang, K. Tou, X. Liu, P. Wen, H. Kinoh, Y. Anraku, H. Chen, K. Kataoka and Z. Ge, Therapeutic Polymersome Nanoreactors with Tumor-Specific Activable Cascade Reactions for Cooperative Cancer Therapy, ACS Nano, 2019, 13, 2357–2369 CAS
.
- Z. Yao, B. Zhang, T. Liang, J. Ding, Q. Min and J.-J. Zhu, Promoting Oxidative Stress in Cancer Starvation Therapy by Site-Specific Startup of Hyaluronic Acid-Enveloped Dual-Catalytic Nanoreactors, ACS Appl. Mater. Interfaces, 2019, 11, 18995–19005 CrossRef CAS
.
- M. Huo, L. Wang, Y. Chen and J. Shi, Tumor-Selective Catalytic Nanomedicine by Nanocatalyst Delivery, Nat. Commun., 2017, 8, 357 CrossRef
.
- L. Zhang, S.-S. Wan, C.-X. Li, L. Xu, H. Cheng and X.-Z. Zhang, An Adenosine Triphosphate-Responsive Autocatalytic Fenton Nanoparticle for Tumor Ablation with Self-Supplied H2O2 and Acceleration of Fe(III)/Fe(II) Conversion, Nano Lett., 2018, 18, 7609–7618 CrossRef CAS
.
- Y. Duan, F. Ye, Y. Huang, Y. Qin, C. He and S. Zhao, One-Pot Synthesis of a Metal-Organic Framework-Based Drug Carrier for Intelligent Glucose-Responsive Insulin Delivery, Chem. Commun., 2018, 54, 5377–5380 RSC
.
- W.-H. Chen, G.-F. Luo, M. Vázquez-González, R. Cazelles, Y. S. Sohn, R. Nechushtai, Y. Mandel and I. Willner, Glucose-Responsive Metal-Organic-Framework Nanoparticles Act as “Smart” Sense-and-Treat Carriers, ACS Nano, 2018, 12, 7538–7545 CrossRef CAS
.
- Y. Zhang, J. Wang, J. Yu, Di Wen, A. R. Kahkoska, Y. Lu, X. Zhang, J. B. Buse and Z. Gu, Bioresponsive Microneedles with a Sheath Structure for H2O2 and pH Cascade-Triggered Insulin Delivery, Small, 2018, 14, e1704181 CrossRef
.
- Y. Liu, X. Ji, W. W. L. Tong, D. Askhatova, T. Yang, H. Cheng, Y. Wang and J. Shi, Engineering Multifunctional RNAi Nanomedicine To Concurrently Target Cancer Hallmarks for Combinatorial Therapy, Angew. Chem., Int. Ed., 2018, 57, 1510–1513 CrossRef CAS
.
- R. Zhang, L. Feng, Z. Dong, L. Wang, C. Liang, J. Chen, Q. Ma, Q. Chen, Y. Wang and Z. Liu, Glucose & Oxygen Exhausting Liposomes for Combined Cancer Starvation and Hypoxia-Activated Therapy, Biomaterials, 2018, 162, 123–131 CrossRef CAS
.
- J. Lee, E.-T. Oh, H. Yoon, C. W. Kim, Y. Han, J. Song, H. Jang, H. J. Park and C. Kim, Mesoporous Nanocarriers with a Stimulus-Responsive Cyclodextrin Gatekeeper for Targeting Tumor Hypoxia, Nanoscale, 2017, 9, 6901–6909 RSC
.
- S. Li, X. Jiang, R. Zheng, S. Zuo, L. Zhao, G. Fan, J. Fan, Y. Liao, X. Yu and H. Cheng, An Azobenzene-Based Heteromeric Prodrug for Hypoxia-Activated Chemotherapy by Regulating Subcellular Localization, Chem. Commun., 2018, 54, 7983–7986 RSC
.
- X. Zhang, M. Wu, J. Li, S. Lan, Y. Zeng, X. Liu and J. Liu, Light-Enhanced Hypoxia-Response of Conjugated Polymer Nanocarrier for Successive Synergistic Photodynamic and Chemo-Therapy, ACS Appl. Mater. Interfaces, 2018, 10, 21909–21919 CrossRef CAS
.
- K. Kiyose, K. Hanaoka, D. Oushiki, T. Nakamura, M. Kajimura, M. Suematsu, H. Nishimatsu, T. Yamane, T. Terai, Y. Hirata and T. Nagano, Hypoxia-sensitive fluorescent probes for in vivo real-time fluorescence imaging of acute ischemia, J. Am. Chem. Soc., 2010, 132, 15846–15848 CrossRef CAS
.
- S. Zbaida and W. G. Levine, A novel application of cyclic voltammetry for direct investigation of metabolic intermediates in microsomal azo reduction, Chem. Res. Toxicol., 1991, 4, 82–88 Search PubMed
.
- D. Tarn, D. P. Ferris, J. C. Barnes, M. W. Ambrogio, J. F. Stoddart and J. I. Zink, A Reversible Light-Operated Nanovalve on Mesoporous Silica Nanoparticles, Nanoscale, 2014, 6, 3335–3343 RSC
.
- Z. Gao, T. Shi, Y. Li, C. You, K. Cheng and B. Sun, Mesoporous Silica-Coated Gold Nanoframes as Drug Delivery System for Remotely Controllable Chemo-Photothermal Combination Therapy, Colloids Surf., B, 2019, 176, 230–238 CrossRef CAS
.
- D.-W. Zheng, Q. Lei, J.-Y. Zhu, J.-X. Fan, C.-X. Li, C. Li, Z. Xu, S.-X. Cheng and X.-Z. Zhang, Switching Apoptosis to Ferroptosis: Metal-Organic Network for High-Efficiency Anticancer Therapy, Nano Lett., 2017, 17, 284–291 CrossRef CAS
.
- C. He, Y. Hu, L. Yin, C. Tang and C. Yin, Effects of Particle Size and Surface Charge on Cellular Uptake and Biodistribution of Polymeric Nanoparticles, Biomaterials, 2010, 31, 3657–3666 CrossRef CAS
.
- T. Sun, Y. S. Zhang, B. Pang, D. C. Hyun, M. Yang and Y. Xia, Engineered Nanoparticles for Drug Delivery in Cancer Therapy, Angew. Chem., Int. Ed., 2014, 53, 12320–12364 CAS
.
- Y. Liu, G. Shu, X. Li, H. Chen, B. Zhang, H. Pan, T. Li, X. Gong, H. Wang, X. Wu, Y. Dou and J. Chang, Human HSP70 Promoter-Based Prussian Blue Nanotheranostics for Thermo-Controlled Gene Therapy and Synergistic Photothermal Ablation, Adv. Funct. Mater., 2018, 28, 1802026 CrossRef
.
Footnote |
† Electronic supplementary information (ESI) available. See DOI: 10.1039/c9tb02268f |
|
This journal is © The Royal Society of Chemistry 2020 |