An Au@NH2-MIL-125(Ti)-based multifunctional platform for colorimetric detections of biomolecules and Hg2+†
Received
5th October 2019
, Accepted 8th November 2019
First published on 28th November 2019
Abstract
In this report, a novel nanocomposite of highly dispersed Au nanoparticles deposited on NH2-MIL-125(Ti) was designed and proposed as a peroxidase-like mimic. Compared with individual Au nanoparticles and NH2-MIL-125(Ti), the peroxidase-like nanozyme exhibits enhanced peroxidase-like activity. Moreover, kinetic analysis reveals that the as-prepared nanozyme has lower Km values and stronger affinity towards both substrates than the natural horseradish peroxidase (HRP). The detection limits of the multifunctional platform for H2O2, cysteine and Hg2+ are down to 0.24 μM, 0.14 μM and 100 nM, respectively. This work provides rapid and sensitive colorimetric detection strategies for small biomolecules and Hg2+, which have great potential for application in biosensors and biotechnology.
1. Introduction
H2O2 and cysteine play crucial roles in biological systems and their abnormal levels have been proved to be risk factors of diverse diseases. Moreover, even a low content of mercury(II) ions (Hg2+) can lead to damage in the brain, kidney, nerve and endocrine system.1–3 Thus, sensitive detection of these target molecules is highly desirable. Among various reported detection strategies for these target molecules and metal ions, the enzyme-based colorimetric sensing technique has been practiced as one of the most powerful means for the point-of-care detection of these species through a change in color, due to its rapid visual determination, high sensitivity and high-throughput.4–8 However, its practical applications in colorimetric sensing have been severely blocked by the considerable drawbacks of natural enzymes, such as high-cost, low stability and sensitivity to harsh environments. To overcome these drawbacks, nanozymes, nanoparticle-based enzyme mimics, have been investigated intensively in the past decade in virtue of their various intriguing advantages such as high-stability, low-cost and adjustable-activity.8–11 To date, various nanomaterials including Fe3O4,12 nanoceria,13,14 graphene oxide,15 carbon nanodots,16 metal metallic nanoparticles,17–19etc. have been explored to mimic natural enzymes. Among the various developed nanomaterial-based enzyme mimics, metallic Au nanoparticles have been extensively recognized as peroxidase or oxidase-like biomimetics.18–20 However, their practical applications have been inevitably blocked by intrinsic drawbacks such as easy aggregation and limited catalytic efficiency.
To address these issues, various strategies have been developed to improve the catalytic properties of metallic Au-based nanozymes. Among the developed strategies, combing Au with other support materials, such as graphene and metal organic frameworks, is promising and attractive, because the resulting composites may exhibit higher activities owing to the synergistic effect between the single components.21–23 Due to the intrinsic advantages of metal organic frameworks, several Au/MOF composites have been fabricated and applied in catalysis and sensing.22–28 For example, Liu et al. reported the detection of DNA using an Au@Fe-MIL-88 hybrid as a peroxidase-like nanozyme, and the nanohybrid exhibited enhanced activity compared to pure Fe-MIL-88 and Au NPs.23 Hu et al. developed a highly sensitive detection strategy for p-phenylenediamine using Au/MIL-101 as the SERS substrate.25 These studies demonstrated that Au/MOF composites have great potential for application in biomedical analysis and environmental monitoring as mimic enzymes. However, among the various reported Au@MOF composites, only Au@Fe-MIL-88 and Au/MIL-101 have been applied as nanozymes. Moreover, only Au@Fe-MIL-88 was used as a nanozyme for colorimetric sensing (DNA).
NH2-MIL-125(Ti), a porous amino-functionalized titanium-based MOF, has been proved to be an efficient and stable support for metallic nanoparticles (Pd, Pt, and Au) and the resulting composites exhibited excellent heterogeneous catalytic performance in Suzuki cross coupling (Pd),29 electrocatalytic oxidation of hydrazine (Au)26 and photocatalytic alcohol oxidation (Pt and Au).30 However, none of the M@NH2-MIL-125 composites have been explored as nanozymes and applied in the colorimetric sensing of biomolecules and metal ions. Taken together, to the best of our knowledge, neither the enzyme mimic characteristic nor the application in colorimetric assays of the Au@NH2-MIL-125(Ti) composite has been explored.
Inspired by the above considerations, as depicted in Scheme 1, a composite was prepared by depositing Au nanoparticles on NH2-MIL-125(Ti) and its peroxidase-like characteristic and enzymatic kinetics were firstly investigated in detail. Based on the excellent peroxidase-like activity and inhibition effect of cysteine, a multifunctional platform for rapid, convenient and sensitive colorimetric detection of H2O2, cysteine and Hg2+ with very low detection limits has been developed.
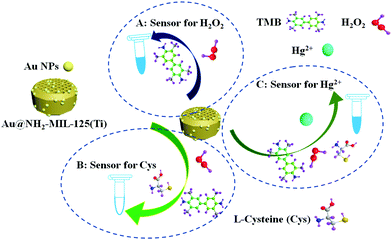 |
| Scheme 1 Schematic illustration for the preparation of Au@NH2-MIL-125(Ti) nanocomposite and colorimetric detections of H2O2, cysteine and Hg2+. | |
2. Experimental
2.1. Preparation of NH2-MIL-125(Ti)
NH2-MIL-125(Ti) was synthesized by a conventional solvothermal procedure similar to reported methods.31 Generally, 1.63 g of 2-aminoterephthalate (9 mmol) was first dissolved in a dehydrated mixed solution containing 27 ml of DMF and 3 ml of MeOH in a 50 ml Teflon-lined autoclave. Consequently, 0.72 ml of tetrabutyl titanate (2.25 mmol) was dropped into the mixture under sonication in order to obtain a uniform solution. Afterward, the autoclave was sealed and heated to 150 °C in an oven for 48 h. After cooling down, the powder was separated by centrifugation. After washing with DMF and MeOH, the powder was dried at 60 °C for 6 h in a vacuum oven.
2.2. Loading of Au nanoparticles into NH2-MIL-125(Ti)
Typically, 100 mg of NH2-MIL-125(Ti) was first dispersed in 20 ml of ultrapure water and then 1.00 ml of HAuCl4 (1%) aqueous solution was dropped into the mixture. After 5 min of sonication, 6 mg of sodium borohydride was added to reduce the gold ions into metallic nanoparticles. After sonicating for 20 min, the solution was centrifuged, and the solid was washed several times with methanol.
2.3. Characterization
The crystal structures of the materials were examined by X-ray powder diffraction (XRD) in the 2θ range from 5 to 80 degree using a Bruker D4 Endeavour equipped with Cu Kα radiation (λ = 0.15405 nm). The structure and morphology of the materials were analyzed by scanning electronic microscopy (SEM, Hitachi S-5500). X-ray photoelectron spectroscopy (XPS) measurement was carried out using a PHI ESCA-5000C electron spectrometer. The Au contents in NH2-MIL-125 samples were tested using an inductively coupled plasma mass spectrometer (ICP-MS, Thermo NexION 1000).
2.4. Peroxidase-like catalytic activities of Au@NH2-MIL-125(Ti)
The oxidation of a color substrate 3,3′,5,5′-tetramethylbenzidine (TMB) with H2O2 was selected as a model reaction to examine the peroxidase-like activities of Au@NH2-MIL-125(Ti) and related materials. Catalyst aqueous suspensions (1 mg ml−1) were prepared in advance by dispersing materials in water under sonication for 10 min. Typically, 0.1 ml of the catalyst suspension and 0.1 ml of TMB solution (10 mM in DMSO) were mixed well with 4.3 ml of acetate buffer solution (pH = 4.0) in a quartz cell. Finally, 0.5 ml of H2O2 (50 mM) was injected into the above mixture to start the reaction. The peroxidase-like activities were monitored by recording the UV-vis spectra or absorbance at 652 nm of the reaction mixture for a total of 10 min. To compare the enzymatic kinetics, we measured the steady-state kinetics for both TMB and H2O2 as substrates under optimum conditions. The kinetic data were obtained by changing one substrate concentration and fixing that to the other substrate. The Michaelis Menten parameters were calculated from the Lineweaver Burk plots
1/V = 1/Vmax + Km/(Vmax[S]) |
where V and Vmax represent the initial and maximum velocity, respectively, [S] is the substrate concentration, and Km is the Michaelis constant.
2.5. Radical trapping experiments
Typically, scavengers (50 mM), such as terephthalic acid (TA) and isopropanol (TPA) as ˙OH scavengers, and benzoquinone (BQ) as an ˙O2− scavenger, were added into the H2O2
+
TMB + Au@NH2-MIL-125(Ti) system while keeping other conditions fixed.
2.6. Colorimetric detection of H2O2 and cysteine
Typically, a colorimetric reaction was performed as follows to detect H2O2, 0.5 ml of H2O2 at varying concentrations of 0.1–80 mM was injected into a mixture containing 0.1 ml of TMB (5 mM) and 0.1 ml of the catalyst suspension (1 mg ml−1) in 4.3 ml of acetate buffer solution (100 mM, pH = 4.0). The successive concentration-dependent changes of the absorbance at 652 nm of the reaction mixtures were evaluated to calculate their initial velocities and further to calculate the kinetic parameters. The colorimetric detection of cysteine is the same as the sensing of H2O2. Briefly, cysteine at different concentrations was mixed with 4.3 ml of acetate buffer solution (pH = 4) containing 0.1 ml of Au@NH2-MIL-125 aqueous suspension (1 mg ml−1), 0.1 ml of TMB solution (5 mM in DMSO) and 0.5 ml of H2O2 (50 mM). Then the ΔA at 652 nm was measured by UV-vis absorption spectroscopy. To study the selectivity for cysteine towards other biomolecules found in biosystems, Au@NH2-MIL-125 was incubated with various amino acids, such as phenylalanine (Phe), histidine (His), glucose (Glu), tryptophan (Trp), methionine (Met), valine (Val), ascorbic acid (AA), and glutathione (GSH) instead of cysteine. And their ΔA values at 652 nm were measured to be the same as the detection of cysteine.
The feasibility of the developed assay for cysteine detection in biological samples was evaluated by spiking cysteine in fetal bovine serum, which was diluted with acetate buffer (pH = 4.0, 100 mM) at a ratio of 1
:
100. The added cysteine (4.0 μM, 7.0 μM, and 9.0 μM) in real samples was analyzed following the same procedure as described above.
2.7. Colorimetric detection of Hg2+
For Hg2+ detection, Hg2+ in various amounts was introduced into the acetate buffer solution (pH = 4.0) containing cysteine (60 μm) and Au@NH2-MIL-125(Ti) (20 μg ml−1). One minute later, H2O2 (5 mM) and TMB (0.1 mM) were added. After reacting for 10 min, the absorbances at 652 nm were recorded. Subsequently, to investigate the selectivity of Hg2+ towards other metal ions, the changes of the absorbance at 652 nm were determined to be the same as the detection of Hg2+, except for adding various other metal ions of the same concentrations instead of Hg2+. Moreover, in order to eliminate the interference of other ions, EDTA (1 mM) and NaCl (0.5 mM), acting as masking agents, were added together with the metal ions into the reaction mixture. The practical applicability of this colorimetric assay for the detection of Hg2+ in tap water was further investigated following the same procedures.
3. Results and discussion
3.1. Characterization of the synthesized materials
Scheme 1 shows a schematic illustration of the fabrication of Au@NH2-MIL-125(Ti). Briefly, NH2-MIL-125(Ti) was first synthesized by a solvothermal reaction. Subsequently, Au@NH2-MIL-125(Ti) was fabricated by impregnation followed by reduction with sodium borohydride. The crystalline structures of the as-synthesized NH2-MIL-125(Ti) and Au@NH2-MIL-125(Ti) were analyzed first by means of the X-ray diffraction (XRD). As shown in Fig. 1a, the XRD pattern of prepared NH2-MIL-125 shows remarkable characteristic peaks at 2θ ranging from 5 to 20°, which are in perfect agreement with those of the simulated patterns shown in Fig. 1b of NH2-MIL-125(Ti).31–33 Furthermore, the loading of Au NPs on NH2-MIL-125(Ti) does not affect its crystalline structure. As shown in Fig. 1d, broad and weak peaks attributed to the Au NPs are observed, probably due to the uniform dispersion of the gold nanoparticles on NH2-MIL-125.
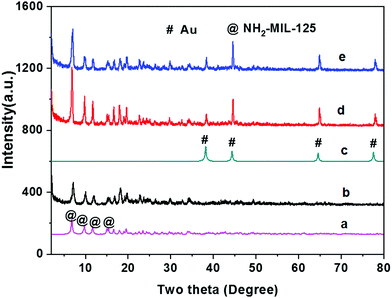 |
| Fig. 1 XRD patterns of materials. (a) As-prepared NH2-MIL-125(Ti); (b) simulated NH2-MIL-125(Ti); (c) simulated Au; (d) Au@NH2-MIL-125(Ti). | |
The morphologies, structures and composition of the as-prepared NH2-MIL-125(Ti) and Au@NH2-MIL-125(Ti) were further investigated by TEM and SEM techniques. As can be observed in both SEM and TEM images (Fig. 2), the obtained NH2-MIL-125 sample is exclusively characteristic of plate shape. The plates have an average diameter of 500 nm and a thickness of 300 nm (Fig. 2b). As shown in the TEM and SEM images, small Au nanoparticles with an average diameter less than 5 nm were observed both on the surface and in the internal pores. In addition, the incorporation of Au did not affect the shape and the structure of NH2-MIL-125. SEM-EDX measurement was further applied to explore the existence of Au and surface composition in Au@NH2-MIL-125(Ti). As shown in Fig. 2g, peaks assigned to both Ti and Au elements were observed in the spectra. The Au loading amount was quantitatively determined by ICP-MS, and the weight percent was calculated to be about 3%. All the results described above well demonstrated that Au nanoparticles were incorporated on and into NH2-MIL-125(Ti) without destroying its crystalline structure and shape.
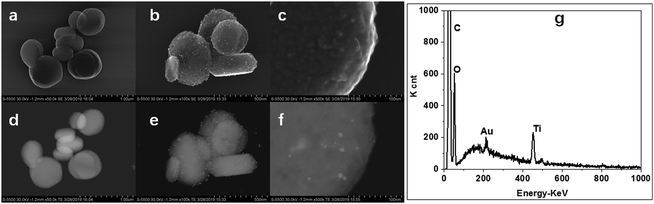 |
| Fig. 2 TEM, SEM images and SEM-EDX spectra. (a) SEM images of NH2-MIL-125(Ti); (b and c) Au@NH2-MIL-125(Ti); TEM images of (d) NH2-MIL-125(Ti) and (e and f) Au@NH2-MIL-125(Ti); and SEM-EDX spectra (g) of Au@NH2-MIL-125(Ti). | |
The surface compositions and chemical states of the NH2-MIL-125(Ti) and Au@NH2-MIL-125(Ti) composites were further investigated by X-ray photoelectron spectroscopy (XPS) measurements. Fig. 3a shows the survey XPS spectra of the NH2-MIL-125(Ti) (red line) and Au@NH2-MIL-125(Ti) (black line) samples. The results clearly reveal that the Au@NH2-MIL-125(Ti) composite mainly consists of C, N, O, Ti and Au atoms while there are no Au atoms in the NH2-MIL-125, which is in good agreement with their SEM-EDX results. The Ti 2p spectrum of the Au@NH2-MIL-125(Ti) sample shows two contributions, located respectively at 458.8 and 464.5 eV, which are assigned to be the binding energy values of Ti 2p3/2 and Ti 2p1/2, indicating that titanium bound to oxygen remains in oxidation state IV for the titanium–oxo cluster.33 As shown in Fig. 3c, the presence of the reduced form of Au0 in the Au@NH2-MIL-125(Ti) was evidenced by the Au 4f7/2 and Au 4f5/2 peaks located respectively at 83.9 and 87.6 eV. Furthermore, the shift of the N 1s binding energy of Au@NH2-MIL-125 when compared with that of pristine NH2-MIL-125(Ti) provides evidence of an electronic interaction between the N of the amine functionality and the deposited Au nanoparticles.33
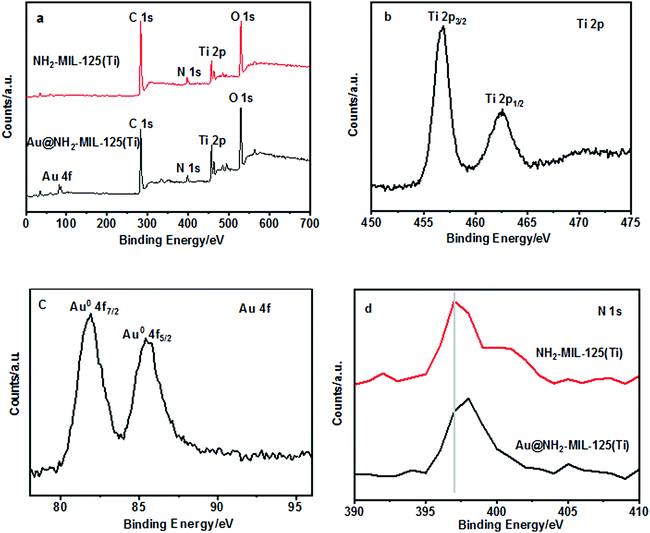 |
| Fig. 3 XPS spectra of NH2-MIL-125(Ti) and Au@NH2-MIL-125(Ti). (a) Survey; (b) Ti 2p; (c) Au 4f; and (d) N 1s. | |
3.2. Peroxidase-like catalytic activity
In order to explore the peroxidase-like catalytic properties of the as-prepared Au@NH2-MIL-125(Ti), the oxidation of TMB with the aid of H2O2 was tested. TMB is a chromogenic substrate which would display blue color in the presence of peroxidase and H2O2. The concentration of the oxidized product ox-TMB can be quantitatively determined by measuring the absorbance values at 652 nm. Therefore, the peroxidase-like activities were monitored by recording the time-dependent absorbance UV-vis spectra or absorbance at 652 nm of the reaction mixture at a 1 min interval for a total of 10 min. As shown in Fig. 4a, the absorbance of the reacted solutions at 652 nm increased linearly in the time-dependent absorbance spectra, resulting from the formation of a charge transfer complex and a radical cation. And then, we conducted a series of control experiments to further explore the peroxidase-like activity of the Au@NH2-MIL-125 hybrid. As shown in Fig. 4b, TMB and H2O2, TMB with Au@NH2-MIL-125(Ti), and H2O2 with Au@NH2-MIL-125(Ti) systems are colorless or light blue and display weak absorbance peaks at 652 nm, while TMB, H2O2 and Au@NH2-MIL-125(Ti) system display a dark blue color and a strong absorbance peak at 652 nm, indicating that the Au@NH2-MIL-125(Ti) nanohybrid is an efficient peroxidase-like biomimetic which can catalyze the reaction of H2O2 oxidizing TMB to form ox-TMB. As shown in Fig. 4c, it is worth mentioning that Au@NH2-MIL-125(Ti) exhibits stronger peroxidase-like activity than single NH2-MIL-125(Ti) and Au nanoparticles. Herein, we speculate that the enhancement is probably due to the synergistic effect between the single components of the nanohybrid. In order to obtain the best catalytic activity, we further investigated the influence of pH values on the catalytic activity of Au@NH2-MIL-125(Ti) because the peroxidase-like activity of nanozymes is sensitive to the pH values.11,34–36 As shown in Fig. 4d, like the natural enzymes and most reported peroxidase-like nanozymes, the highest catalytic activity was found at pH = 4.0. Therefore, the optimal pH value for the reaction system is 4.0.
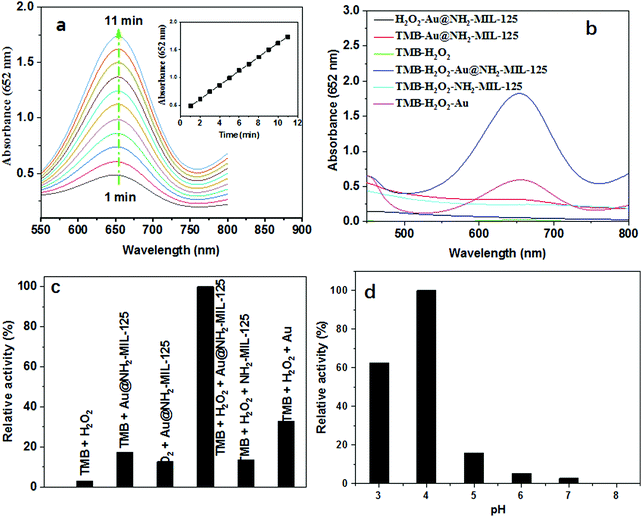 |
| Fig. 4 (a) Time-dependent changes of UV-vis absorption curves of TMB oxidation (0.2 mM) in the presence of H2O2 (5 mM) and the Au@NH2-MIL-125(Ti) nanohybrid (20 μg ml−1) in acetate buffer solution (pH = 4.0). The inset shows the plot of absorbance at 652 nm versus time; (b) UV-vis absorption curves of different reaction systems after 10 min, the TMB, H2O2 and catalyst concentrations are 0.2 mM, 5 mM and 20 μg ml−1, respectively; (c) comparison of the relative catalytic activities of different reaction systems; (d) relative catalytic activities of the Au@NH2-MIL-125(Ti) nanohybrid catalyst versus various pH values. | |
To gain a better understanding of the catalytic mechanism of the peroxidase-like nanozyme, Au@NH2-MIL-125(Ti) was further characterized as a biomimetic enzyme by an apparent steady state kinetic analysis with H2O2 and TMB as substrates, respectively. In order to understand the catalytic mechanism and acquire kinetic parameters, kinetic experiments were performed by varying the concentration of one substrate while keeping the concentration of another constant. The concentrations of the blue product were calculated from the obtained absorbances at 652 nm using a molar attenuation coefficient value of 39
000 M−1 cm−1. It could be seen in Table 1 that the average Km value for H2O2 of the prepared Au@NH2-MIL-125(Ti) nanohybrid is 1.75 mM which is lower than that of horse radish peroxidase (HRP) (3.7 mM). On the other hand, the average Km value for TMB was calculated to be 0.20 mM, which is also lower than the value for HRP (0.43 mM). The calculated average Km values suggest that this nanostructure has higher substrate affinities than the HRP enzyme.12 It is worth noting that, as is observed for natural HRP, the proximate parallel double reciprocal lines shown in Fig. 5b and d verify its ping-pong mechanism.6,36
Table 1 Kinetic parameters of Au@NH2-MIL-125 and horseradish peroxidase (HRP) with TMB and H2O2 as substrates, respectively
Substrate |
Catalysts |
K
m/mM |
Substrate |
K
m/mM |
TMB |
HRP |
0.4346 |
H2O2 |
3.712 |
Au@NH2-MIL-125(Ti) |
0.20
this work
|
1.75
this work
|
Fe3O4 |
0.0986 |
15412 |
MoS2-PPy-Pd |
0.9322 |
6.422 |
Co3O4@CeO2 |
0.04620 |
0.3520 |
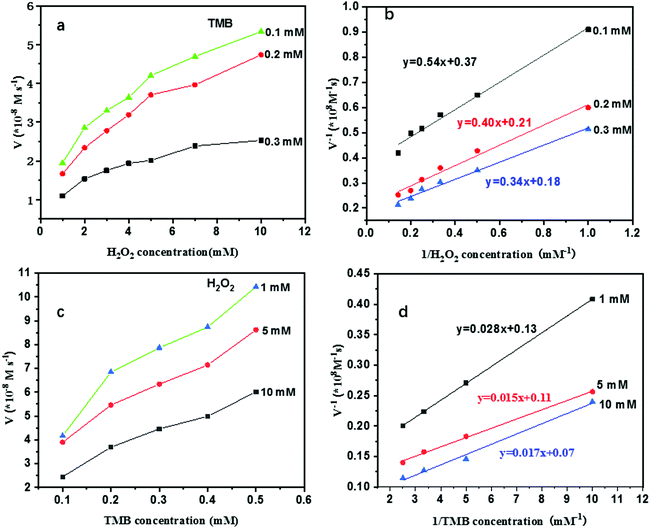 |
| Fig. 5 Steady-state kinetic assays for Au@NH2-MIL-125(Ti). Experimental conditions: catalyst concentration 20 μg ml−1, acetate buffer solution 5 ml and pH = 4. (a) TMB concentration was fixed at 0.1, 0.2, and 0.3 mM, respectively, while the concentrations of H2O2 varied from 1 to 10 mM; (c) H2O2 concentration was fixed at 1, 5, and 10 mM, respectively, while the concentrations of TMB varied from 0.1 to 0.5 mM; (b and d) the corresponding double reciprocal plots of H2O2 and TMB. | |
It is commonly known that the mechanism in peroxidase-like nanozymes stems from their ability to catalyze the decomposition of H2O2 to generate ˙OH and ˙O2−.36–39 Therefore, herein, radical trapping agents were used to elucidate the catalytic mechanism of Au@NH2-MIL-125(Ti). As shown in Fig. 6, typical scavengers, terephthalic acid (TA) and isopropanol (TPA) as ˙OH scavengers, and benzoquinone (BQ) as a ˙O2− scavenger, were added into the H2O2
+
TMB + Au@NH2-MIL-125(Ti) system while keeping other conditions fixed. As expected, the absorbance at 652 nm decreased sharply with the addition of BQ as a radical trapping agent, while only a slight change in adsorption at 652 nm can be observed in the system by adding TA or IPA. Thus, it can be deduced that the strong oxidizing ˙O2− radicals generated during the reaction between Au@NH2-MIL-125(Ti) and H2O2 played major roles in the catalytic reaction. TMB can be oxidized to be the corresponding blue oxidation product by the generated ˙O2− radicals.
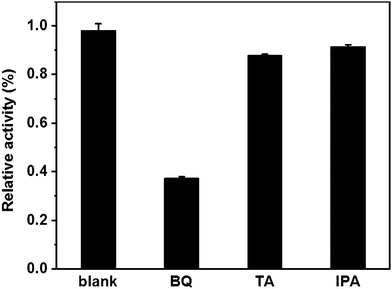 |
| Fig. 6 Relative activity (%) of the H2O2 + TMB + system in the absence (blank) or presence of radical scavengers (TA, IPA and BQ). | |
As we know, H2O2 plays an important role in biological systems, clinical diagnosis, environment, and food manufacturing.1,4,5,40 Therefore, the sensitive detection of H2O2 is very important and has attracted much attention. It has been well established that the color and absorbance change of TMB oxidation catalyzed by peroxidase-like nanomaterials such as Au@NH2-MIL-125(Ti), which has been proved to be a superior peroxidase-like nanozyme, are reported to be H2O2 concentration-dependent. Therefore, Au@NH2-MIL-125(Ti) was first used for the sensitive detection of H2O2 by a colorimetric strategy. As clearly shown in Fig. 7a, the absorbance values at 652 nm of the reaction system increase with the increase of the H2O2 concentration. Typically, the absorbance values are in a fixed proportion to the H2O2 concentrations ranging from 2 to 10 μM. Accordingly, the detection limit is calculated to be as low as 0.24 μM (S/N = 3), which is lower than those by other methods (shown in Table S1, ESI†). All the above results indicate that the developed nanozyme-based sensor has great potential for quantitative detection of H2O2 with ultrahigh sensitivity.
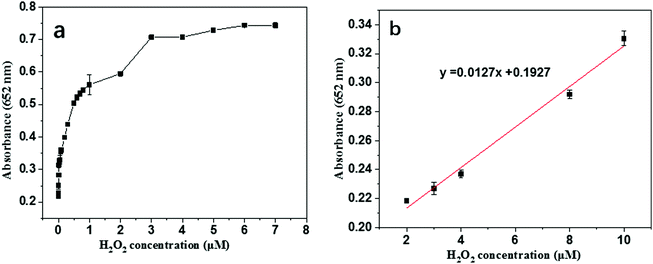 |
| Fig. 7 (a) The dose–response curve with regard to the H2O2 detection; (b) the corresponding calibration curve. | |
Bio-thiols, such as cysteine, play key roles in the process of reversible redox reactions in physiological systems and the presence of abnormal contents will lead to various diseases.41–43 Therefore, it is vital important to develop sensitive sensing methods for cysteine. As is known to all, TMB can be oxidized into a blue product by H2O2 in the presence of a catalyst. However, the oxidation can be totally or completely reduced by adding cysteine due to its antioxidant properties. Furthermore, the enzymatic activity can be inhibited by cysteine due to its strong bonding capacity onto the Au surface to mask the active sites. Both effects lead to the reduction of TMB oxidation and lighten the blue color of the reaction system. Based on the above discussions, a colorimetric strategy for cysteine detection has been developed. Accordingly, the ΔA values at 652 nm of the reaction solution, catalyzed by the Au@NH2-MIL-125, were determined in the presence of cysteine with varying concentrations ranging from 1 μm to 1000 mM. As displayed in Fig. 8a, it is obvious that the ΔA values increase along with the increasing cysteine concentration. Moreover, a good linear trend is achieved in the range of 1–10 μM. Accordingly, the detection limit (LOD) for cysteine sensing is calculated to be 0.14 μM (S/N = 3), which is lower than or at least comparable to previously reported colorimetric methods for cysteine detection (shown in Table S2, ESI†). This linear range and detection limit for cysteine detection are reasonable. Therefore, Au@NH2-MIL-125 could be used as an effective peroxidase-like nanozyme and has potential for application in colorimetric detection of cysteine.
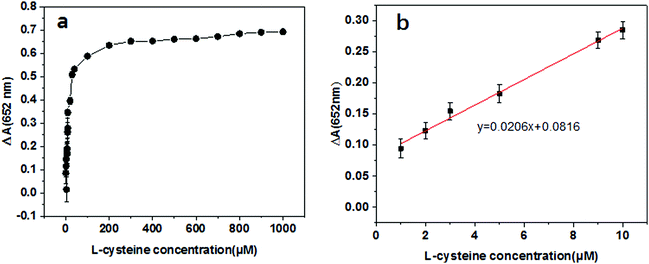 |
| Fig. 8 (a) Curve graph of absorbance versus concentrations of cysteine and (b) the corresponding linear calibration plot for cysteine. Experimental conditions: TMB (0.1 mM), H2O2 (5 mM), catalyst suspension (20 μg ml−1), and reaction time (10 min). | |
Specificity is another crucial index in cysteine detection. Therefore, we further studied the selectivity of detection towards other biomolecules. Fig. 9 shows the inhibition effects of some typical common amino acids and glucose on the TMB oxidation. Obviously, in the presence of cysteine, the ΔA values are much higher than those of other used interferences except for glutathione, indicating that cysteine shows an efficient inhibition effect on the reaction system. On this basis, we constructed a rapid and sensitive colorimetric method for cysteine detection.
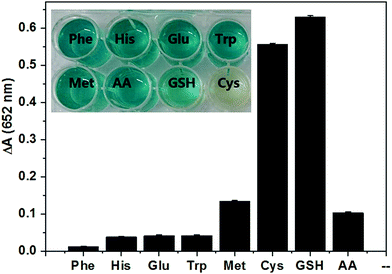 |
| Fig. 9 Inhibition effects of various amino acids and glucose on TMB oxidation. Experimental conditions: TMB (0.1 mM), H2O2 (5 mM), catalyst dispersion (20 μg ml−1), amino acid and glucose concentration (100 μM), and reaction time (10 min). | |
Considering that a variety of diseases are associated with cysteine, it is of importance to explore the reliability of the developed assay under physiological conditions.44–48 It is well known that human blood plasma contains high concentrations of cysteine (165–335 μm),44 homocysteine (9–13 μM)45,46 and glutathione (14–20 μm).47 Herein, the practicality of the provided assay for cysteine detection was evaluated with fetal bovine serum as the sample by applying a standard addition method.48 The standard curve (Fig. S1, ESI†) is obtained by conducting cysteine detection in the as-prepared serum sample according to the developed assay, where cysteine with different concentrations was spiked into the diluted fetal bovine serum and human serum and then quantified according to the procedure discussed above. The recoveries of the cysteine are found to distribute within the range from 93.8% to 103.5% with RSD values ranging from 3.2% to 6.1% as shown in Table 2. The desirable results validate the reliability and practicality of the provided colorimetric assay for cysteine analysis in real samples. Taken together, we established a reliable colorimetric assay that can be readily applied in biological applicability by measuring cysteine in fetal bovine serum and human serum.
Table 2 Determination of cysteine in fetal bovine serum
Samples |
Added/μM |
Founda/μM |
Recovery% |
RSD/% |
Mean of five determinations ± standard deviation.
|
Fetal bovine serum |
4.0 |
3.754 |
93.8 |
3.23 |
7.0 |
7.022 |
100.3 |
5.62 |
9.0 |
9.320 |
103.5 |
6.13 |
Mercury ions (Hg2+) can cause a series of serious health and environmental problems due to their high biological accumulation and toxic properties49–54 Therefore, it is urgent to develop sensitive and selective detection strategies for Hg2+ in water and blood. Colorimetric assays have been proved to be a rapid, visual read-out and high throughput analysis method for Hg2+ detection. As discussed above, the catalytic activity of Au@NH2-MIL-125(Ti) was greatly inhibited in the presence of cysteine at certain concentrations. As described in previous reports, Hg2+ has higher affinity towards –SH than that of Au, thereby the inhibited activity can be completely or partially recovered by the existence of various concentration of Hg2+.54 Thus, herein, a colorimetric method for Hg2+ detection was developed using cysteine as an inhibitor. Although the catalytic activity of Au@NH2-MIL-125(Ti) toward TMB oxidation can be fully inhibited by a higher concentration of cysteine, the recovery of catalytic activity was not easy in the presence of excess cysteine. Therefore, the detection of Hg2+ was carried out with 60 μM cysteine. As expected, along with the increasing concentration of Hg2+, the absorption values increased gradually and present a linear relationship ranging from 1 to 5 μM (Fig. 10a). The detection limit of Hg2+ calculated from the linear equation presented as y = 0.03023x + 0.21155 in Fig. 10b was determined to be as low as 100 nM (S/N = 3), which is much lower than the established maximum tolerant level of Hg2+ in blood (0.01 mg L−1 and 500 nM).54 As shown in Table S3 (ESI†), our results were compared with other previously reported Hg2+ detection methods. The linear trend and LOD value of this assay are comparable to some of previously reported fluorometric and colorimetric based assays.50–58 However, a lower LOD value and improved sensitivity are desirable to meet the permissible value (0.006 mg L−1 and 30 nM) for drinking water.51
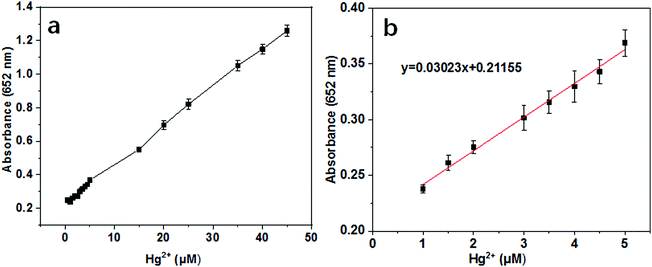 |
| Fig. 10 (a) Dose–response curve for absorbance at 652 nm vs. Hg2+ concentration and (b) the linear calibration plot for detection of Hg2+. Experimental details: TMB (0.1 mM), H2O2 (5 mM), catalyst dispersion (20 μg ml−1), cysteine (60 μM), and reaction time (5 min). | |
As shown in Fig. 11, the selectivity for Hg2+ was determined against various other metal ions in the presence of cysteine. As we know, Hg2+ has a larger stability constant with thiol ligands than other metallic ions, including Ni2+, Co2+, Zn2+, Pb2+, and Zr4+, which is the prerequisite for the selective detection of Hg2+. However, obvious interference of some metal ions like Ag+, Fe3+, Cu2+ and Bi3+ was observed. Compared to the control test, the existence of such metal ions also enhances the absorbance value. This phenomenon can be ascribed to two possible reasons. Some ions like Cu2+ and Bi3+ also have high binding capacities compared to thiol ligands. Meanwhile, some ions like Ag+ and Fe3+ have intrinsic catalytic activity toward TMB oxidation. Therefore, to eliminate the potential interference from these ions, EDTA and chloride (molar ratio = 2
:
1) were used as masking agents. Notably, the interferences of these ions were almost eliminated completely in the presence of these two masking agents. Concurrently, the presence of Hg2+ can be detected sensitively without interference, even with the naked eye by the change of the color of the solution from colorless to blue.
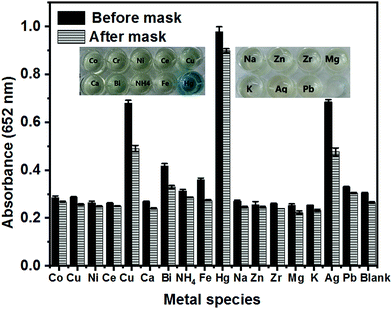 |
| Fig. 11 Absorbance of the TMB solutions before and after masking (0.5 mM EDTA, 1 mM NaCl) in the presence of 100 μM different metal ions. | |
The practical applicability of this colorimetric assay for the detection of Hg2+ in environmental water samples was further investigated. The standard addition method was used to detect the concentrations of Hg2+ in tap water from Dalian Minzu University. Hg2+ at different concentrations was spiked into the water samples and then quantified according to the procedure discussed above. The results are listed in Table 3. It can be observed that the recovery values of Hg2+ in the water samples are between 91.56 and 106.9%. The relative standard deviations (RSD, n = 5) at each concentration level are all less than 3.03%. The results indicate that this assay can be used for the qualitative analysis of Hg2+ in environmental water samples with good recovery and precision.
Table 3 Determination of Hg2+ in the environmental water sample
Samples |
Added/μM |
Founda/μM |
Recovery% |
RSD/% |
Mean of five determinations ± standard deviation.
|
Tap water |
3 |
3.123 |
104.1 |
3.03 |
6 |
5.493 |
91.56 |
2.03 |
9 |
9.629 |
106.9 |
2.53 |
4. Conclusion
In summary, we have fabricated an Au@NH2-MIL-125(Ti) composite by an impregnation method followed by a borohydride reduction method, in which small and uniform Au metallic nanoparticles were successfully deposited on the surface and entrapped into the nanocages of NH2-MIL-125(Ti). The resulting nanohybrid nanozyme demonstrated an obviously better peroxidase-like catalytic activity than single Au NPs and NH2-MIL-125(Ti). Its superior peroxidase-like activity was attributed to the synergistic effect of the small size of Au NPs and NH2-MIL-125(Ti). Furthermore, the kinetic experiments revealed that the nanozyme has lower Km values for both TMB and H2O2 compared to natural HRP, indicating its higher affinity to both substrates. Motivated by these results, a multifunctional platform for the facile colorimetric sensing of hydrogen peroxide, cysteine and Hg2+ was developed. The detection limits for H2O2 and cysteine were as low as 0.24 μM and 0.14 μM, respectively. By introducing EDTA and chloride as masking agents, Hg2+ can be selectively detected with a very low detection limit down to 100 nM without interference of many other metal ions. Furthermore, the practical applicability for detection of both cysteine in fetal bovine serum and Hg2+ in tap water was confirmed. In summary, the proposed multifunctional platform is sensitive, fast and selective, and shows great promise for practical applications in biosensing and biotechnology.
Conflicts of interest
There are no conflicts to declare.
Acknowledgements
This work was financially supported by the National Natural Science Foundation of China (21473190, 21203017), the Liaoning Natural Science Foundation of China (20180551022) and the Fundamental Research Funds for the Central Universities.
References
- H. Liu, L. Zhu, H. Ma, J. Wen, H. Xu and Y. Qiu,
et al., Copper(II)-coated Fe3O4 nanoparticles as an efficient enzyme mimic for colorimetric detection of hydrogen peroxide, Microchim. Acta, 2019, 186, 518 CrossRef.
- Y. Xiong, S. Chen, F. Ye, L. Su, C. Zhang and S. Shen,
et al., Synthesis of a mixed valence state Ce-MOF as an oxidase mimetic for the colorimetric detection of biothiols, Chem. Commun., 2015, 51, 4635–4638 RSC.
- W. Chansuvarn, T. Tuntulani and A. Imyim, Colorimetric detection of mercury(II) based on gold nanoparticles, fluorescent gold nanoclusters and other gold-based nanomaterials, TrAC, Trends Anal. Chem., 2015, 65, 83–96 CrossRef CAS.
- S. Mvango and P. Mashazi, Synthesis, characterization of copper oxide-gold nanoalloys and their peroxidase-like activity towards colorimetric detection of hydrogen peroxide and glucose, Mater. Sci. Eng., C, 2019, 96, 814–823 CrossRef CAS.
- Y. Wang, D. Zhang and J. Wang, Metastable α-AgVO3 microrods as peroxidase mimetics for colorimetric determination of H2O2, Microchim. Acta, 2018, 185, 1 CrossRef.
- C. Ray, S. Dutta, S. Sarkar, R. Sahoo, A. Roy and T. Pal, Intrinsic peroxidase-like activity of mesoporous nickel oxide for selective cysteine sensing, J. Mater. Chem. B, 2014, 2, 6097–6105 RSC.
- Y.-W. Wang, Q. Liu, L. Wang, S. Tang, H.-H. Yang and H. Song, A colorimetric mercury(II) assay based on the Hg(II)-stimulated peroxidase mimicking activity of a nanocomposite prepared from graphitic carbon nitride and gold nanoparticles, Microchim. Acta, 2019, 186, 7 CrossRef.
- R. Andre, F. Natalio, M. Humanes, J. Leppin, K. Heinze, R. Wever, H. C. Schroeder, W. E. G. Mueller and W. Tremel, V2O5 Nanowires with an Intrinsic Peroxidase-Like Activity, Adv. Funct. Mater., 2011, 21(3), 501–509 CrossRef CAS.
- J. Golchin, K. Golchin, N. Alidadian, S. Ghaderi, S. Eslamkhah and M. Eslamkhah,
et al., Nanozyme applications in biology and medicine: an overview, Artif. Cells, Nanomed., Biotechnol., 2017, 45, 1069–1076 CrossRef CAS.
- Y. Zhou, B. Liu, R. Yang and J. Liu, Filling in the Gaps between Nanozymes and Enzymes: Challenges and Opportunities, Bioconjugate Chem., 2017, 28, 2903–2909 CrossRef CAS.
- H. Wei and E. Wang, Nanomaterials with enzyme-like characteristics (nanozymes): next-generation artificial enzymes(II), Chem. Soc. Rev., 2019, 48(4), 1004–1076 RSC.
- L. Gao, J. Zhuang, L. Nie, J. Zhang, Y. Zhang and N. Gu,
et al., Intrinsic peroxidase-like activity of ferromagnetic nanoparticles, Nat. Nanotechnol., 2007, 2, 577–583 CrossRef CAS.
- D. Yang, M. Fa, L. Gao, R. Zhao, Y. Luo and X. Yao, The effect of DNA on the oxidase activity of nanoceria with different morphologies, Nanotechnology, 2018, 29, 385101 CrossRef.
- S. Singh, Cerium oxide based nanozymes: Redox phenomenon at biointerfaces, Biointerphases, 2016, 11, 04B202 CrossRef.
- A. Sun, L. Mu and X. Hu, Graphene Oxide Quantum Dots as Novel Nanozymes for Alcohol Intoxication, ACS Appl. Mater. Interfaces, 2017, 9, 12241–12252 CrossRef CAS.
- M. Shamsipur, A. Safavi and Z. Mohammadpour, Indirect colorimetric detection of glutathione based on its radical restoration ability using carbon nanodots as nanozymes, Sens. Actuators, B, 2014, 199, 463–469 CrossRef CAS.
- K. S. Mckeating, S. Sloandennison, D. Graham and K. Faulds, An investigation into the simultaneous enzymatic and SERRS properties of silver nanoparticles, Analyst, 2013, 138, 6347–6353 RSC.
- Y. Hu, H. Cheng, X. Zhao, J. Wu, F. Muhammad and S. Lin,
et al., Surface-Enhanced Raman Scattering-Active Gold Nanoparticles with Enzyme Mimicking Activities for Measuring Glucose and Lactate in Living Tissues, ACS Nano, 2017, 11, 5558–5566 CrossRef CAS.
- W. Song, M. Chi, M. Gao, B. Zhao, C. Wang and X. Lu, Self-assembly directed synthesis of Au nanorices induced by polyaniline and their enhanced peroxidase-like catalytic properties, J. Mater. Chem. C, 2017, 5, 7465–7471 RSC.
- Y. Zhu, Z. Yang, L. Song, M. Chi, M. Li, C. Wang and X. Lu, Encapsulation of Co3O4 Nanoparticles Inside CeO2 Nanotubes: An Efficient Biocatalyst for the Ultrasensitive Detection of Ascorbic Acid, Part. Part. Syst. Charact., 2018, 35(8), 180049 CrossRef.
- S. Zhang, H. Li, Z. Wang, J. Liu, H. Zhang and B. Wang,
et al., A strongly coupled Au/Fe3O4/GO hybrid material with enhanced nanozyme activity for highly sensitive colorimetric detection, and rapid and efficient removal of Hg2+ in aqueous solutions, Nanoscale, 2015, 7, 8495–8502 RSC.
- M. Chi, Y. Zhu, L. Jing, C. Wang and X. Lu, Fabrication of ternary MoS2-polypyrrole-Pd nanotubes as peroxidase mimics with a synergistic effect and their sensitive colorimetric detection of L-cysteine, Anal. Chim. Acta, 2018, 1035, 146–153 CrossRef CAS.
- Y. L. Liu, W. L. Fu, C. M. Li, C. Z. Huang and Y. F. Li, Gold nanoparticles immobilized on metal-organic frameworks with enhanced catalytic performance for DNA detection, Anal. Chim. Acta, 2015, 861, 55–61 CrossRef CAS.
- B. Tan, H. Zhao, W. Wu, X. Liu, Y. Zhang and X. Quan, Fe3O4-AuNPs anchored 2D metal-organic framework nanosheets with DNA regulated switchable peroxidase-like activity, Nanoscale, 2017, 9, 18699–18710 RSC.
- Y. Hu, J. Liao, D. Wang and G. Li, Fabrication of Gold Nanoparticle-Embedded Metal-Organic Framework for Highly Sensitive Surface-Enhanced Raman Scattering Detection, Anal. Chem., 2014, 86, 3955–3963 CrossRef CAS.
- Y. Han, L. Han, L. Zhang and S. Dong, Ultrasonic synthesis of highly dispersed Au nanoparticles supported on Ti-based metal-organic frameworks for electrocatalytic oxidation of hydrazine, J. Mater. Chem., 2015, 3, 14669–14674 RSC.
- R. Das, P. Pachfule, R. Banerjee and P. Poddar, Metal and metal oxide nanoparticle synthesis from metal organic frameworks (MOFs): finding the border of metal and metal oxides, Nanoscale, 2012, 4, 591–599 RSC.
- A. Dhakshinamoorthy and H. Garcia, Catalysis by metal nanoparticles embedded on metal-organic frameworks, Chem. Soc. Rev., 2012, 41, 5262–5284 RSC.
- P. Puthiaraj and W.-S. Ahn, Highly active palladium nanoparticles immobilized on NH2-MIL-125 as efficient and recyclable catalysts for Suzuki-Miyaura cross coupling reaction, Catal. Commun., 2015, 65, 91–95 CrossRef CAS.
- J. Qiu, L. Yang, M. Li and J. Yao, Metal nanoparticles decorated MIL-125-NH2 and MIL-125 for efficient photocatalysis, Mater. Res. Bull., 2019, 112, 297–306 CrossRef CAS.
- Y. Fu, D. Sun, Y. Chen, R. Huang, Z. Ding and X. Fu,
et al., An Amine-Functionalized Titanium Metal-Organic Framework Photocatalyst with Visible-Light-Induced Activity for CO2 Reduction, Angew. Chem., 2012, 51, 3364–3367 CrossRef CAS.
- Y. Han, L. Han, L. Zhang and S. Dong, Ultrasonic synthesis of highly dispersed Au nanoparticles supported on Ti-based metal-organic frameworks for electrocatalytic oxidation of hydrazine, J. Mater. Chem. A, 2015, 3(28), 14669–14674 RSC.
- J. Qiu, L. Yang, M. Li and J. Yao, Metal nanoparticles decorated MIL-125-NH2 and MIL-125 for efficient photocatalysis, Mater. Res. Bull., 2019, 112, 297–306 CrossRef CAS.
- C. R. Li,
et al., Amplified colorimetric detection of Ag+ based on Ag+-triggered peroxidase-like catalytic activity of ZIF-8/GO nanosheets, Sens. Actuators, B, 2019, 284, 213–219 CrossRef CAS.
- D. D. Zhou, K. Zeng and M. H. Yang, Gold nanoparticle-loaded hollow Prussian blue nanoparticles with peroxidase-like activity for colorimetric determination of L-lactic acid, Microchim. Acta, 2019, 186(2), 7 Search PubMed.
- C. Ge, R. Wu, Y. Chong, G. Fang, X. Jiang and Y. Pan,
et al., Synthesis of Pt Hollow Nanodendrites with Enhanced Peroxidase-Like Activity against Bacterial Infections: Implication for Wound Healing, Adv. Funct. Mater., 2018, 28, 1801484 CrossRef.
- W. Dong, Y. Zhuang, S. Li, X. Zhang, H. Chai and Y. Huang, High peroxidase-like activity of metallic cobalt nanoparticles encapsulated in metal-organic frameworks derived carbon for biosensing, Sens. Actuators, B, 2018, 255, 2050–2057 CrossRef CAS.
- L. Jin, Y. Sun, L. Shi, C. Li and Y. Shen, PdPt bimetallic nanowires with efficient oxidase mimic activity for the colorimetric detection of acid phosphatase in acidic media, J. Mater. Chem. B, 2019, 7, 4561–4567 RSC.
- D. J. Porter and H. J. Bright, The mechanism of oxidation of nitroalkanes by horseradish peroxidase, J. Biol. Chem., 1983, 258, 9913–9924 CAS.
- H. Liu, H. Ma, H. Xu, J. Wen, Z. Huang and Y. Qiu,
et al., Hollow and porous nickel sulfide nanocubes prepared from a metal-organic framework as an efficient enzyme mimic for colorimetric detection of hydrogen peroxide, Anal. Bioanal. Chem., 2019, 411, 129–137 CrossRef CAS.
- Z. Yang, Y. Zhu, G. Nie, M. Li, C. Wang and X. Lu, FeCo nanoparticles-embedded carbon nanofibers as robust peroxidase mimics for sensitive colorimetric detection of L-cysteine, Dalton Trans., 2017, 46, 8942–8949 RSC.
- Z. Hu, X. Jiang, F. Xu, J. Jia, Z. Long and X. Hou, Colorimetric sensing of bithiols using photocatalytic UiO-66(NH2) as H2O2-free peroxidase mimics, Talanta, 2016, 158, 276–282 CrossRef CAS.
- X. Wu, Y. Xu, Y. Chen, H. Zhao, H. Cui and J. Shen,
et al., Peroxidase-like activity of ferric ions and their application to cysteine detection, RSC Adv., 2014, 4, 64438–64442 RSC.
- Y. Li, Z. Zhang, Z. Tao, X. Gao, S. Wang and Y. Liu, A Asp/Cenanotube-based colorimetric nanosensor for H2O2-free and enzyme-free detection of Cys, Talanta, 2019, 196, 556–562 CrossRef CAS PubMed.
- Y. Liu, X. Lv, M. Hou, Y. Shi and W. Guo, Selective Fluorescence Detection of Cysteine over Homocysteine and Glutathione Based on a Cysteine-Triggered Dual Michael Addition/Retro-aza-aldol Cascade Reaction, Anal. Chem., 2015, 87(22), 11475–11483 CrossRef CAS.
- R. S. Vasan, A. Beiser, R. B. D'Agostino, D. Levy, J. Selhub, P. F. Jacques, I. H. Rosenberg and P. W. F. Wilson, Plasma Homocysteine and Risk for Congestive Heart Failure in Adults Without Prior Myocardial Infarction, JAMA, J. Am. Med. Assoc., 2003, 289(10), 1251–1257 CrossRef CAS.
- D. Ścibior, M. Skrzycki, M. Podsiad and H. Czeczot, Clin. Biochem., 2008, 41, 852–858 CrossRef.
- Z. Jiang, Y. Liu, X. Hu and Y. F. Li, Colorimetric determination of thiol compounds in serum based on Fe-MIL-88NH2 metal-organic framework as peroxidase mimetics. Analytical, Methods, 2014, 6, 5647–5651 CAS.
- C. Peng, Y. Zhang, L. Wang, Z. Jin and G. Shao, Colorimetric assay for the simultaneous detection of Hg2+ and Ag+ based on inhibiting the peroxidase-like activity of core-shell Au@Pt nanoparticles. Analytical, Methods, 2017, 9, 4363–4370 CAS.
- C. Wang, G. Tang and H. Tan, Colorimetric determination of mercury(II) via the inhibition by ssDNA of the oxidase-like activity of a mixed valence state cerium-based metal-organic framework, Mikrochim. Acta, 2018, 185, 475 CrossRef.
- Y. Wang, S. Tang, H. Yang and H. Song, A novel colorimetric assay for rapid detection of cysteine and Hg2+ based on gold clusters, Talanta, 2016, 146, 71–74 CrossRef CAS.
- T. Li, S. Dong and E. Wang, Label-Free Colorimetric Detection of Aqueous Mercury Ion (Hg2+) Using Hg2+-Modulated G-Quadruplex-Based DNAzymes, Anal. Chem., 2009, 81, 2144–2149 CrossRef CAS.
- S. Satyabrata, A. Kotal and T. K. Mandal, One-Dimensional Assembly of Peptide-Functionalized Gold Nanoparticles: An Approach Toward Mercury Ion Sensing, J. Phys. Chem. C, 2007, 111, 1248–1255 CrossRef.
- K. Z. Kamali, A. Pandikumar, S. Jayabal, R. Ramaraj, H. N. Lim and B. H. Ong,
et al., Amalgamation based optical and colorimetric sensing of mercury(II) ions with silver@graphene oxide nanocomposite materials, Microchim. Acta, 2016, 183, 369–377 CrossRef.
- Z. B. Shang, Y. Wang and W. J. Jin, Triethanolamine-capped CdSe quantum dots as fluorescent sensors for reciprocal recognition of mercury(II) and iodide in aqueous solution, Talanta, 2009, 78, 364–369 CrossRef CAS.
- H. Kawasaki, K. Yoshimura, K. Hamaguchi and R. Arakawa, Trypsin-stabilized fluorescent gold nanocluster for sensitive and selective Hg2+ detection, Anal. Sci., 2011, 27, 591 CrossRef CAS.
- X. Xu, J. Wang, K. Jiao and X. Yang, Colorimetric detection of mercury ion (Hg2+) based on DNA oligonucleotides and unmodified gold nanoparticles sensing system with a tunable detection range, Biosens. Bioelectron., 2009, 24, 3153–3158 CrossRef CAS.
- K. Z. Kamali, A. Pandikumar, S. Jayabal, R. Ramaraj, H. N. Lim and B. H. Ong, Amalgamation based optical and colorimetric sensing of mercury(II) ions with silver@graphene oxide nanocomposite materials, Microchim. Acta, 2016, 183, 369–377 CrossRef.
Footnote |
† Electronic supplementary information (ESI) available. See DOI: 10.1039/c9tb02183c |
|
This journal is © The Royal Society of Chemistry 2020 |