Theoretical insights into nonprecious oxygen-evolution active sites in Ti–Ir-Based perovskite solid solution electrocatalysts†
Received
11th September 2019
, Accepted 14th November 2019
First published on 19th November 2019
Abstract
Deep understanding of the properties of catalytically active sites on complex solid surfaces will benefit the rational design of catalysts with desirable performances. Here, we present DFT calculations that demonstrate the unexpected ability of nonprecious titanium sites on SrTix(Ir)1−xO3 solid solution (STIO) surfaces to electrocatalyze the oxygen evolution reaction (OER), and the crucial roles of iridium content (defined by the atomic ratio Ir/(Ir + Ti) in STIO) and spatial distribution in generating highly active titanium catalytic sites. The surface iridium sites for STIO with iridium content from 12% to 50% show higher activity than those on IrO2 surfaces. The surface titanium sites for STIO with iridium content from 25% to 75% show high catalytic activity similar to iridium catalytic sites, while the surface titanium sites for SrTiO3 (STO) are intrinsically catalytically inert for the OER. These results suggest that for an optimum catalytic activity to be realized, STIO should have an iridium content between 25% and 50% due to the existence of efficient, dual metallic catalytic sites, in agreement with our previous experimental observation. Additionally, the eg-filling of surface titanium sites for STIO is found to be reduced with the increase of the iridium content and strongly correlates with the surface adsorption ability of surface titanium sites towards the key reaction intermediates and thereby the catalytic activity for the OER. Besides the iridium content, the spatial distribution of iridium in STIO is also found to be vital to activate surface titanium sites. The results show that the surface iridium atoms play a dominant role in the activation of surface titanium sites, while the inner iridium atoms have a minor impact on this activation. As presented in this work, a novel core/shell-type material model, where an iridium-free STO core is covered with a single-layered-thick STIO shell, is overall suggested to achieve highly active oxygen evolution electrocatalysts with an ultralow iridium content.
Introduction
The three key electrochemical reactions, including H2O splitting, CO2 reduction and N2 fixation, are considered to play a central role in future renewable energy systems.1–3 In these three reactions, the oxygen evolution reaction (OER) as an essential half reaction is inevitably involved and its effectiveness impacts the whole catalysis system. The OER is a 4e−/4H+-coupled, sluggish process and correspondingly a large overpotential must be applied to overcome the high intrinsic reaction barrier of the OER.4–6 Hence, reduction of the overpotential using appropriate OER electrocatalysts is one of the essential tasks to make the whole catalysis system less energy-intensive. While the state-of-the-art electrocatalysts for the OER in alkaline media are based on nonprecious Fe, Co and/or Ni elements,7–13 the most reliable OER electrocatalysts in acidic solutions comprise precious iridium (Ir) element.14–19 Ir-containing electrocatalysts have been proven to integrate high activity with reasonable stability.20–22 However, the high cost and low earth-abundance of iridium make the relevant electrochemical devices (e.g., proton exchange membrane (PEM) water electrolysers) unfeasible for large-scale commercial deployment.23 Therefore, it is necessary to develop OER electrocatalysts with a greatly reduced (if not eliminated) iridium content and at least similar (if not higher) catalytic activity in comparison with the benchmark Ir-based catalyst.
To this end, researchers have been exploring some new low-iridium catalytic systems beyond IrO2, where nonprecious elements are always involved to construct the target catalysts. When some acid-stable nonprecious oxides have been used as support materials for IrO2-based OER electrocatalysts,24–27 some nonprecious elements have been shown to serve as auxiliary structural components for the construction of Ir-based electrocatalysts with complex crystal structures, such as perovskite15,17,28,29 and pyrochlore.30,31 Despite recent efforts, the iridium content in the majority of OER electrocatalysts is still higher than 50 wt%. Recently, our group reported SrTix(Ir)1−xO3 (STIO) solid solutions with 28 wt% iridium as efficient oxygen evolution electrocatalysts exhibiting remarkable activity superior to the benchmark catalyst IrO2 in acid.14 STIO represents an important low-iridium electrocatalyst, which contains the least iridium mass fraction among the existing Ir-based electrocatalysts. These positive results inspire us to further explore the bimetallic synergic effect and to better understand the properties of catalytically active sites on STIO. This knowledge is expected to be fundamental to allow the rational design of OER electrocatalysts with better performances.
Herein, we conduct DFT calculations to evaluate the properties of bimetallic catalytic sites for the OER with a series of STIO model materials. Our results reveal that both iridium content and spatial distribution play crucial roles in generating highly active titanium catalytic sites for the OER. Our results further suggest a novel core/shell-type material model, where an iridium-free STO core is covered with a single-layered-thick STIO shell, for designing highly active OER electrocatalysts with an ultralow iridium content.
Methods
The density functional theory (DFT) calculations were carried out using the generalized gradient approximation (GGA) with the Perdew–Burke–Ernzerhof (PBE) exchange-correlation functional32 in the framework of the Vienna ab initio simulation package (VASP).33,34 The electron–ion interactions were described with the projector augmented wave (PAW) method and a plane-wave basis set of 400 eV was used.35,36 For all the calculations, the convergence threshold was set as 10−4 eV in energy and 0.05 eV Å−1 in force. A 5 × 5 × 5 Monkhorst–Pack grid37 was used for the calculation of the bulk models. As for slab models, 4 × 4 × 1 and 7 × 7 × 1 Monkhorst–Pack grids were employed for the geometric optimization and projected density of states (PDOS) calculations, respectively. The DFT-D2 method was included for the correction of the van der Waals interactions.38 The dipolar correction was included and the symmetry was switched off for the calculations of slab models. A vacuum space of 15 Å was added on the surface to prevent the interlayer interactions, and the upper two of the eight atomic layers were fully relaxed while the remaining kept frozen.
To establish the STIO bulk models with various iridium contents and doping positions, the structure of the cubic SrTiO3 unit cell was optimized first. The optimized lattice parameter was 3.898 Å, which is close to the experimental value (3.905 Å).39 Then, a 2 × 2 × 2 SrTiO3 supercell was built based on the SrTiO3 unit cell and Ir-doped SrTiO3 supercells were further used as the STIO models with various iridium contents and doping positions (Table S1 and Fig. S1, ESI†). Among the various possible doping configurations in the same Ir
:
Ti molar ratio, the one with the lowest energy was chosen as the corresponding STIO model for next calculations here. The optimized lattice parameters of these models are shown in Table S2, ESI.† The slab model of STIO was obtained by cleaving the corresponding STIO bulk model through the (001) plane (Fig. S2, ESI†), and the surfaces with simultaneously exposed Ir and Ti atoms were studied in this work. For comparison, the slab models of SrTiO3 (STO) and SrIrO3 (SIO) were built by using 2 × 2 × 2 STO and SIO supercells with a cubic structure (Fig. S3, ESI†), respectively.
The catalytic activity of the surface metallic site was evaluated using the methods reported by Nørskov et al.40,41 The Gibbs free energy for each elementary step is described as follows:
| ΔG1 = E(HO*) − E(*) − EH2O + 1/2EH2 + (ΔZPE − TΔS)1 − eU | (1) |
| ΔG2 = E(O*) − E(HO*) +1/2EH2 + (ΔZPE − TΔS)2 − eU | (2) |
| ΔG3 = E(HOO*) − E(O*) − EH2O + 1/2EH2 + (ΔZPE − TΔS)3 − eU | (3) |
| ΔG4 = E(*) − E(HOO*) + EO2 + 1/2EH2 + (ΔZPE − TΔS)4 − eU | (4) |
where
E(*),
E(HO*),
E(O*) and
E(HOO*) represent the total energies of the pure surface and the three intermediate species adsorbed on the surface, respectively.
EH2O,
EH2 and
EO2 are the total energies for single H
2O, H
2 and O
2 molecules, respectively. The free energy of O
2 is fixed at 2.46 eV, which is the experimental value of the free energy change of the reaction H
2O → 1/2O
2 + H
2. The value of zero-point energy is obtained by calculating the vibrational frequencies and the entropy correction for gas phase molecules is considered (Tables S9 and 10 in the ESI
†). The theoretical overpotential is defined as max{Δ
G(1−4)}/e −1.23 V.
The eg-filling of surface titanium atoms was obtained by integrating n(ε) with the correlated energy up to the Fermi level.42,43
in which
n(
ε) is the projected density of states of the e
g orbital for the corresponding surface titanium atom.
Results and discussion
In order to investigate the composition effect on the catalytic activities of surface sites for the OER, we establish a series of STIO models (Fig. 1, left), with different atomic ratios of iridium and titanium (denoted as STIO-x, where x represents the atomic ratio of Ir/(Ir + Ti)). The (001) surfaces of these STIO models are studied as they are the most exposed surfaces according to our previous experimental results.14 All the titanium and iridium sites on the STIO (001) surfaces are investigated as the possible catalytic sites for the OER in our calculations. On the (001) surfaces of STIO, there are usually several types of titanium and iridium sites with different atomic environments (as shown in Fig. S2, ESI†).
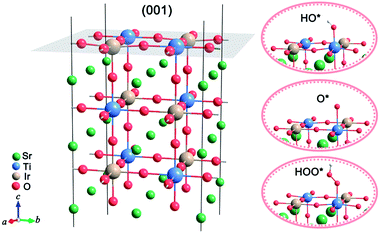 |
| Fig. 1 Structural model with the exposed (001) surface of STIO (left) and schematic of HO*, O* and HOO* intermediates adsorbed at the Ti site on the (001) surface, respectively (right). | |
The OER activities of different titanium and iridium sites on the STIO (001) surface are investigated according to the four-electron transfer pathway [eqn (5)–(8)] proposed by Nørskov et al.40,41
| H2O + * → HO* + H+ + e− | (5) |
| H2O + O* → HOO* + H+ + e− | (7) |
| HOO* → * + O2 + H+ + e− | (8) |
in which * represents a surface Ir or Ti site in the STIO model, and HO*, O* and HOO* are the adsorbed intermediates during the OER (
Fig. 1, right). The adsorption energies of these intermediates are Δ
GOH, Δ
GO, and Δ
GOOH, respectively. The potential-determining step (PDS) involved in the OER is the elementary step that has the highest unfavorable equilibrium potential or needs the highest energy to pass over the overall energy landscape for the OER (
i.e., max{Δ
G(1−4)}/e). The theoretical overpotential (
η) is described as the difference between max{Δ
G(1−4)}/e and the standard equilibrium potential of the OER (1.23 V).
Fig. 2a shows the theoretical overpotentials correlated with iridium contents for all Ti and Ir sites in STIO-x. The surface iridium sites for STIO with iridium content from 12% to 50% show slightly lower overpotentials than those on the benchmark catalyst IrO2 (0.59 V).15,44 The results suggest that STIO with iridium content from 12% to 50% maintains the good activity of SIO, while the latter has higher intrinsic OER activity than the benchmark catalyst IrO2 (Fig. S4†). The surface titanium sites of STIO with iridium content from 25% to 75% exhibit low overpotentials similar to iridium catalytic sites, while the surface titanium sites for SrTiO3 (STO) with a high overpotential of 1.45 V are intrinsically catalytically inert for the OER. We further consider the active site concentration on the STIO surface (Fig. S11a, ESI†), and the result shows that for iridium content from 25% to 50%, the active site concentration is the highest. Hence, STIO should have a high density of efficient, dual metallic catalytic sites when the iridium content is between 25% and 50%, resulting in optimum catalytic activity. These results are consistent with our previous experimental observation.14
 |
| Fig. 2 (a) Negative theoretical overpotentials for different Ti sites and Ir sites on the (001) surfaces of STIO-x. For comparison, the theoretical overpotential for the Ir sites on the IrO2 surface is also involved (see the black line). (b) Volcano-type plot of OER activities for the most active Ti sites on the (001) surfaces of STIO-x. (c) Gibbs free energy diagrams for the OER on surface Ti sites in STIO-12.5% (blue) and STIO-25% (green) with U = 1.23 V. (d) Gibbs free energy diagrams for the OER on surface Ti sites in STIO-75% (green) and STIO-87.5% (blue) with U = 1.23 V. The free energy change for the PDS is emphasized by the black arrows in (c) and (d). | |
Considering that the coverage of the surface under oxidizing conditions may have an effect on the activity of the surface catalytic sites,17 it's necessary to confirm the activity of STIO on the stable surface terminations. Taking STIO, STIO-25% and STIO-50% as examples, we construct surface phase diagrams to determine the stable surface terminations under oxidizing conditions (Fig. S5a–c, ESI†).44,48 The result shows that the surface with a high coverage of HO* is more stable under oxidizing conditions. The recalculated self-consistent activity for the titanium and iridium sites on the 1 monolayer (ML) HO* covered surface is shown in Fig. S5d.† The results show that on the stable surface terminations, the overpotentials for surface Ti and Ir sites are generally close to that for the bare surface.
To further understand the catalytic properties of titanium sites in STIO, the most active titanium sites on each STIO (001) surface as the representative sites are studied. Fig. 2b shows a good volcano-like plot between the theoretical overpotentials of titanium sites and ΔGO–ΔGOH,41 which is a universal activity descriptor for the OER. The right leg of the volcano curve (e.g., STIO-12.5% and STIO-25%) means that the potential-determining step (PDS) is the second step (HO* → O*) during the OER, while the left leg (e.g., STIO-75% and STIO-87.5%) means that the PDS is the third step (O* → HOO*) during the OER. A too low iridium content (x < 25%) and too high iridium content (x > 75%) in STIO-x lead to too weak and too strong adsorption of oxygen-containing intermediates, respectively. Both of these conditions are not favorable for the OER (Fig. 2c and d). Hence, around the peak of the volcano curve (green region in Fig. 2b), the Ti sites have a good balance between the second and the third step involved in the OER, and thereby show optimal catalytic activities for the OER.
The eg orbital filling of transition metal is an important aspect of the electronic structure to reflect the adsorption ability of the oxygen-containing intermediates and thereby the OER activity.45–47 We next investigate the correlation between the eg orbital characters (Fig. 3a) and the surface adsorption properties of the most active surface titanium sites on the STIO (001) surfaces. As shown in Fig. 3b, the eg-filling of the surface titanium sites is reduced with the increase of the iridium content, and linear correlation with the three oxygen-containing intermediates involved in the OER (i.e., O, OH, and OOH). The adsorption abilities of the three intermediates on the surface titanium sites increase as the eg-filling of the surface titanium sites reduces. Moreover, ΔGO changes faster than ΔGHO and ΔGHOO with respect to the eg-filling of surface titanium sites in STIO, making it possible to continuously tailor ΔGO − ΔGOH and thereby OER activity. These results indicate that the activation of the surface titanium sites originates from the optimization of eg-filling of titanium sites in STIO. Unlike titanium sites, the activity of the iridium sites in STIO may not simply depend on the eg-filling (Fig. S6†).
 |
| Fig. 3 (a) The geometry of the eg orbital (dz2−r2 orbital is exhibited as an example) of a Ti site on the surface. (b) Adsorption free energy of HOO*, O* and HO* plotted as a function of eg-filling of the most active Ti sites on the (001) surfaces of STIO-x. The correlated iridium content is shown at the bottom. The green region represents the optimum range of eg-filling for OER activity. The lines are gained from fitting. | |
Besides the effect of the iridium content on the activity of the surface titanium sites in STIO, the effect of the spatial position of iridium is also studied. The corresponding models are constructed by using one iridium atom to substitute a titanium atom in the STO model, where all of the possible 4 planes in the STO model are considered (Fig. 4a and S7, ESI†). Then, the surface titanium sites in all of these models are evaluated for the OER. Fig. 4b shows the activity of each surface titanium site as a function of ΔGO − ΔGHO. The result shows that the activities of surface titanium sites in the model with iridium doped in plane 1 (i.e., the outmost surface) is much higher than those in the models with the iridium doped in the remain three planes, as well as those in STO. These results demonstrate that the surface iridium atoms are essential to improve the OER activity of the surface titanium sites, while the inner iridium atoms have a relatively small effect on the activity of the surface titanium sites. The same method was used to study the effect of the spatial position of titanium (Fig. S8 and 9†). The titanium atom doping near the surface (e.g. plane 1–3) will decrease the activity of the surface iridium sites. When the doping position is deeper than plane 4, the effect of the titanium doping can be neglected.
 |
| Fig. 4 (a) Schematic of an iridium atom substituting a titanium atom on different planes of the STO model. The planes 1–4 represent possible doped planes of the STO model. (b) Negative theoretical overpotential as a function of ΔGO − ΔGHO for the surface titanium sites in the STO model with iridium doped in plane 1 (red), plane 2 (yellow), plane 3 (green), and plane 4 (blue), respectively. The overpotential (black) for a surface titanium site in STO is also shown. | |
The effects of iridium content and spatial distribution on the activity of the surface Ti sites in STIO give us inspiration to create a novel core/shell-type model to further lower the iridium content in the OER catalyst. The models are iridium-free STO cores covered with a single-layered-thick STIO shell (Fig. S10, ESI†). To determine the suitable iridium content in the STIO shell, the iridium contents in the STIO shell are set as 25%, 50%, 75%, and 100%, respectively. There are several different types of iridium and titanium sites in the surfaces, as shown in the Fig. 5 inset. The surface iridium sites in the STIO shell with iridium content from 25% to 75% show higher or similar activity compared with that in IrO2, while surface titanium sites with iridium content from 50% to 75% in the STIO shell exhibit activities close to that for iridium sites in IrO2. And the active site concentrations on surface of STIO-shell with iridium content from 50% to 75% are 100% (Fig. S11b†). Thus, the core/shell-type model needs as low as 50% iridium content in the STIO shell to achieve a high OER performance for all the surface catalytic sites. These results demonstrate that the core/shell-type model provides a case of guiding significance for the synthesis of efficient, low iridium OER electrocatalysts.
 |
| Fig. 5 The theoretical overpotentials for all surface Ti and Ir sites with the increase of iridium content in a novel core/shell-type material model. The inset shows top views of four surfaces and corresponding surface Ti and Ir sites. | |
Conclusions
In summary, we have demonstrated that both the content and spatial distribution of iridium in STIO are essential factors for the activation of the surface titanium sites toward the OER. Surface titanium sites for STIO with iridium content from 25% to 75% have the optimal activity because of their optimal eg-filling and thereby suitable adsorption ability of the key intermediates. Moreover, the surface iridium atoms show the dominant effects on the surface titanium sites, while the inner iridium atoms have negligible effects. Based on these results, we present a novel core/shell-type model with an ultralow iridium content as an efficient OER electrocatalyst, where an iridium-free STO core is covered with a single-layered-thick STIO skin. Our findings provide an important theoretical basis for designing effective OER electrocatalysts with a low amount of precious metals.
Conflicts of interest
There are no conflicts to declare.
Acknowledgements
X. Zou acknowledges the financial support from the National Key R&D Program of China, Grant No. 2017YFA0207800, the National Natural Science Foundation of China (NSFC) Grant No. 21771079 and 21922507, the Jilin Province Science and Technology Development Plan 20170101141JC, the Program for JLU Science and Technology Innovative Research Team (JLUSTIRT) and the Fok Ying Tung Education Foundation, Grant No. 161011. H. Chen acknowledges the financial support from the NSFC (Grant No. 21901083), Postdoctoral Innovative Talent Support Program (Grant No. BX20180120) and China Postdoctoral Science Foundation (Grant No. 2018M641771). We thank the NSFC (21621001) and the 111 Project (B17020) for financial support. We thank the LvLiang Cloud Computing Center of China, and the calculations were performed on TianHe-2.
Notes and references
- Z. W. Seh, J. Kibsgaard, C. F. Dickens, I. Chorkendorff, J. K. Nørskov and T. F. Jaramillo, Science, 2017, 355, eaad4998 CrossRef
.
- J. G. Chen, R. M. Crooks, L. C. Seefeldt, K. L. Bren, R. M. Bullock, M. Y. Darensbourg, P. L. Holland, B. Hoffman, M. J. Janik, A. K. Jones, M. G. Kanatzidis, P. King, K. M. Lancaster, S. V. Lymar, P. Pfromm, W. F. Schneider and R. R. Schrock, Science, 2018, 360, eaar6611 CrossRef
.
- X. Zou and Y. Zhang, Chem. Soc. Rev., 2015, 44, 5148–5180 RSC
.
- N. T. Suen, S. F. Hung, Q. Quan, N. Zhang, Y. J. Xu and H. M. Chen, Chem. Soc. Rev., 2017, 46, 337–365 RSC
.
- N. S. Lewis and D. G. Nocera, Proc. Natl. Acad. Sci. U. S. A., 2006, 103, 15729–15735 CrossRef CAS PubMed
.
- S. Hammes-Schiffer, Acc. Chem. Res., 2009, 42, 1881–1889 CrossRef CAS
.
- Y. Liu, X. Liang, L. Gu, Y. Zhang, G. D. Li, X. Zou and J. S. Chen, Nat. Commun., 2018, 9, 2609 CrossRef PubMed
.
- F. Guo, Y. Wu, H. Chen, Y. Liu, L. Yang, X. Ai and X. Zou, Energy Environ. Sci., 2019, 12, 684–692 RSC
.
- D. Friebel, M. W. Louie, M. Bajdich, K. E. Sanwald, Y. Cai, A. M. Wise, M. J. Cheng, D. Sokaras, T. C. Weng, R. Alonso-Mori, R. C. Davis, J. R. Bargar, J. K. Norskov, A. Nilsson and A. T. Bell, J. Am. Chem. Soc., 2015, 137, 1305–1313 CrossRef CAS
.
- P. F. Liu, S. Yang, B. Zhang and H. G. Yang, ACS Appl. Mater. Interfaces, 2016, 8, 34474–34481 CrossRef CAS
.
- J. S. Chen, J. Ren, M. Shalom, T. Fellinger and M. Antonietti, ACS Appl. Mater. Interfaces, 2016, 8, 5509–5516 CrossRef CAS
.
- Z. Wang, S. Zeng, W. Liu, X. Wang, Q. Li, Z. Zhao and F. Geng, ACS Appl. Mater. Interfaces, 2017, 9, 1488–1495 CrossRef CAS
.
- M. Liu and J. Li, ACS Appl. Mater. Interfaces, 2016, 8, 2158–2165 CrossRef CAS
.
- X. Liang, L. Shi, Y. Liu, H. Chen, R. Si, W. Yan, Q. Zhang, G. D. Li, L. Yang and X. Zou, Angew. Chem., Int. Ed. Engl., 2019, 58, 7631–7635 CrossRef CAS PubMed
.
- L. Yang, G. Yu, X. Ai, W. Yan, H. Duan, W. Chen, X. Li, T. Wang, C. Zhang, X. Huang, J. S. Chen and X. Zou, Nat. Commun., 2018, 9, 5236 CrossRef
.
- Y. Lee, J. Suntivich, K. J. May, E. E. Perry and Y. Shao-Horn, J. Phys. Chem. Lett., 2012, 3, 399 CrossRef CAS PubMed
.
- L. C. Seitz, C. F. Dickens, K. Nishio, Y. Hikita, J. Montoya, A. Doyle, C. Kirk, A. Vojvodic, H. Y. Hwang, J. K. Norskov and T. F. Jaramillo, Science, 2016, 353, 1011–1014 CrossRef CAS PubMed
.
- J. Zhu, Z. Chen, M. Xie, Z. Lyu, M. Chi, M. Mavrikakis, W. Jin and Y. Xia, Angew. Chem., Int. Ed. Engl., 2019, 58, 7244–7248 CrossRef CAS
.
- H. N. Nong, T. Reier, H.-S. Oh, M. Gliech, P. Paciok, T. H. T. Vu, D. Teschner, M. Heggen, V. Petkov, R. Schlögl, T. Jones and P. Strasser, Nat. Catal., 2018, 1, 841–851 CrossRef CAS
.
- T. Reier, M. Oezaslan and P. Strasser, ACS Catal., 2012, 2, 1765–1772 CrossRef CAS
.
- E. Antolini, ACS Catal., 2014, 4, 1426–1440 CrossRef CAS
.
- S. Cherevko, S. Geiger, O. Kasian, N. Kulyk, J.-P. Grote, A. Savan, B. R. Shrestha, S. Merzlikin, B. Breitbach, A. Ludwig and K. J. J. Mayrhofer, Catal. Today, 2016, 262, 170–180 CrossRef CAS
.
- M. Carmo, D. L. Fritz, J. Mergel and D. Stolten, Int. J. Hydrogen Energy, 2013, 38, 4901–4934 CrossRef CAS
.
- E. Oakton, D. Lebedev, M. Povia, D. F. Abbott, E. Fabbri, A. Fedorov, M. Nachtegaal, C. Copéret and T. J. Schmidt, ACS Catal., 2017, 7, 2346–2352 CrossRef CAS
.
- G. Liu, J. Xu, Y. Wang and X. Wang, J. Mater. Chem. A, 2015, 3, 20791–20800 RSC
.
- W. Sun, Z. Zhou, W. Q. Zaman, L. M. Cao and J. Yang, ACS Appl. Mater. Interfaces, 2017, 9, 41855–41862 CrossRef CAS PubMed
.
- J. M. Hu, J. Q. Zhang and C. N. Cao, Int. J. Hydrogen Energy, 2004, 29, 791–797 CrossRef CAS
.
- O. Diaz-Morales, S. Raaijman, R. Kortlever, P. J. Kooyman, T. Wezendonk, J. Gascon, W. T. Fu and M. T. Koper, Nat. Commun., 2016, 7, 12363 CrossRef CAS
.
- Y. Chen, H. Li, J. Wang, Y. Du, S. Xi, Y. Sun, M. Sherburne, J. W. Ager, A. C. Fisher and Z. J. Xu, Nat. Commun., 2019, 10, 572 CrossRef
.
- C. Shang, C. Cao, D. Yu, Y. Yan, Y. Lin, H. Li, T. Zheng, X. Yan, W. Yu, S. Zhou and J. Zeng, Adv. Mater., 2019, 31, e1805104 CrossRef
.
- D. Lebedev, M. Povia, K. Waltar, P. M. Abdala, I. E. Castelli, E. Fabbri, M. V. Blanco, A. Fedorov, C. Coperet, N. Mazari and T. J. Schmidt, Chem. Mater., 2017, 29, 5182–5191 CrossRef CAS
.
- J. P. Perdew, K. Burke and M. Ernzerhof, Phys. Rev. Lett., 1996, 77, 3865 CrossRef CAS
.
- G. Kresse and J. Furthmüller, Comput. Mater. Sci., 1996, 6, 15–50 CrossRef CAS
.
- G. Kresse and J. Furthmüller, Phys. Rev. B: Condens. Matter Mater. Phys., 1996, 54, 11169 CrossRef CAS
.
- P. E. Blöchl, Phys. Rev. B: Condens. Matter Mater. Phys., 1994, 50, 17953 CrossRef
.
- G. Kresse and D. Joubert, Phys. Rev. B: Condens. Matter Mater. Phys., 1999, 59, 1758 CrossRef CAS
.
- H. J. Monkhorst and J. D. Pack, Phys. Rev. B: Condens. Matter Mater. Phys., 1976, 13, 5188 CrossRef
.
- S. Grimme, J. Comput. Chem., 2006, 27, 1787–1799 CrossRef CAS
.
- R. H. Mitchell, A. R. Chakhmouradian and P. M. Woodward, Phys. Chem. Miner., 2000, 27, 583–589 CrossRef CAS
.
- J. K. Nørskov, J. Rossmeisl, A. Logadottir, L. Lindqvist, J. R. Kitchin, T. Bligaard and H. Jonsson, J. Phys. Chem. B, 2004, 108, 17886–17892 CrossRef
.
- I. C. Man, H.-Y. Su, F. Calle-Vallejo, H. A. Hansen, J. I. Martínez, N. G. Inoglu, J. Kitchin, T. F. Jaramillo, J. K. Nørskov and J. Rossmeisl, ChemCatChem, 2011, 3, 1159–1165 CrossRef CAS
.
- J. R. Kitchin, J. K. Nørskov, M. A. Barteau and J. G. Chen, J. Chem. Phys., 2004, 120, 10240–10246 CrossRef CAS
.
- J. H. Montoya, A. D. Doyle, J. K. Nørskov and A. Vojvodic, Phys. Chem. Chem. Phys., 2018, 20, 3813–3818 RSC
.
- J. Rossmeisl, Z. W. Qu, H. Zhu, G. J. Kroes and J. K. Nørskov, J. Electroanal. Chem., 2007, 607, 83–89 CrossRef CAS
.
- J. Suntivich, H. A. Gasteiger, N. Yabuuchi, H. Nakanishi, J. B. Goodenough and Y. Shao-Horn, Nat. Chem., 2011, 3, 546 CrossRef CAS PubMed
.
- J. Suntivich, K. J. May, H. A. Gasteiger, J. B. Goodenough and Y. Shao-Horn, Science, 2011, 334, 1383–1385 CrossRef CAS PubMed
.
- A. Vojvodic and J. K. Nørskov, Science, 2011, 334, 1355–1356 CrossRef CAS PubMed
.
-
M. Pourbaix, Atlas of Electrochemical Equilibria in Aqueous Solutions, National Association of Corrosion Engineers, Houston, Texas, 1974 Search PubMed
.
Footnotes |
† Electronic supplementary information (ESI) available. See DOI: 10.1039/c9ta10059h |
‡ Lei Shi and Hui Chen contributed equally to this work. |
|
This journal is © The Royal Society of Chemistry 2020 |
Click here to see how this site uses Cookies. View our privacy policy here.