DOI:
10.1039/D0SC04183A
(Edge Article)
Chem. Sci., 2020,
11, 10483-10487
Phosphoryl- and phosphonium-bridged viologens as stable two- and three-electron acceptors for organic electrodes†
Received
30th July 2020
, Accepted 3rd September 2020
First published on 21st September 2020
Abstract
Low molecular weight organic molecules that can accept multiple electrons at high reduction potentials are sought after as electrode materials for high-energy sustainable batteries. To date their synthesis has been difficult, and organic scaffolds for electron donors significantly outnumber electron acceptors. Herein, we report the synthesis and electronic properties of two highly electron-deficient phosphaviologen derivatives from a phosphorus-bridged 4,4'-bipyridine and characterize their electrochemical properties. Phosphaviologen sulfide (PVS) and P-methyl phosphaviologen (PVM) accept two and three electrons at high reduction potentials, respectively. PVM can reversibly accept three electrons between 3–3.6 V vs. Li/Li+ with an equivalent molecular weight of 102 g (mol−1 e−) (262 mA h g−1), making it a promising scaffold for sustainable organic electrode materials having high specific energy densities.
Introduction
Sustainable batteries using non-toxic, abundant metal anodes (Li, Na) and carbon-based cathodes are becoming increasingly viable, pushing the development of electron-accepting organic molecules to the forefront of materials research.1–3 Molecules that can reversibly accept multiple electrons onto a low molecular weight scaffold at high reduction potentials would allow for organic cathodes with high capacity (electrons accepted per gram) and high voltage (high reduction potential vs. the anode). Nevertheless, developing new acceptor scaffolds has been challenging due to the difficulty in synthesizing low molecular weight carbon-based molecules that are so highly electron-withdrawing that they can accept multiple electrons at high reduction potentials. Single-electron acceptors based off benzothiadiazole exhibit high capacity, but rather low voltages of 1–2.5 V vs. Li/Li+.4 Common scaffolds for organic multiple electron acceptors are viologens,5,6 arylene diimides,2,7,8 and aromatic quinones,9–11 which can accept two electrons between 1–3 V vs. Li/Li+. Small molecules that reversibly accept three or more electrons are difficult to find. Our group has developed phosphoryl-bridged viologens (phosphaviologens) that accept two electrons at a reduction potential depending strongly on the valency and functionalization of the phosphorus center.12,13 Phosphaviologen-based materials have been used in high-voltage organic batteries operating between 2.8–3.2 V vs. Li/Li+.14,15 Herein, we show that P-bridged 4,4′-bipyridine can be used to synthesize phosphaviologen sulfide (PVS), a two-electron acceptor, and P-methyl phosphaviologen (PVM), a three electron acceptor. These novel multiple electron acceptors exhibit reversible reductions at between 3–3.6 V vs. Li/Li+. Excluding counterions, the PVS and PVM scaffolds exhibit theoretical capacities of 165 mA h g−1 and 262 mA h g−1, respectively (86 mA h g−1 and 107 mA h g−1 including triflate counterions). PVM in particular was found to be a promising scaffold in Li-ion battery applications as a cathode material.
Results and discussion
PVS and PVM are synthesized using a divergent route starting from 3,3′-dibromo-4,4′-bipyridine, followed by a phosphole ring closing.13 2,7-Diazadibenzophenylphosphole can be converted to PVS in a manner analogous to the synthesis of phosphaviologen oxides (PVO) (Fig. 1A),12 as phosphorus is oxidized using elemental sulfur, and bipyridine is quaternized using methyl triflate. PVS is a dication related to PVO, a two-electron acceptor previously reported by our group, and is expected to accept two electrons in an analogous manner.16,17 The synthesis of PVM is less straightforward, as the reaction of 2,7-diazadibenzophenylphosphole with methyl triflate under normal conditions does not yield the P-methylated product, but rather solely the N-methylated product (see the ESI†). The highly electron-withdrawing nature of viologens renders the phosphorus center unreactive to even very strong electrophiles like methyl triflate. This has also been observed with dipyridinoarsoles,18 and is a common issue with the synthesis of highly electron deficient organic molecules. Only under high temperatures and pressures achieved in a microwave vial, using high boiling solvents can P-methylation occur to form PVM. PVM is a novel tricationic phosphoryl-bridged viologen, and theoretically accepts three electrons: two on the viologen core and one on the bridging phosphorus center. Density Functional Theory (DFT) calculations show PVM as a stronger electron acceptor, having lower lying HOMO and LUMO levels than PVS. The structure of PVM was also confirmed via single crystal X-ray crystallography, with a molecular scaffold showing planar ring systems and weak interactions towards the triflate anion (see the ESI†).
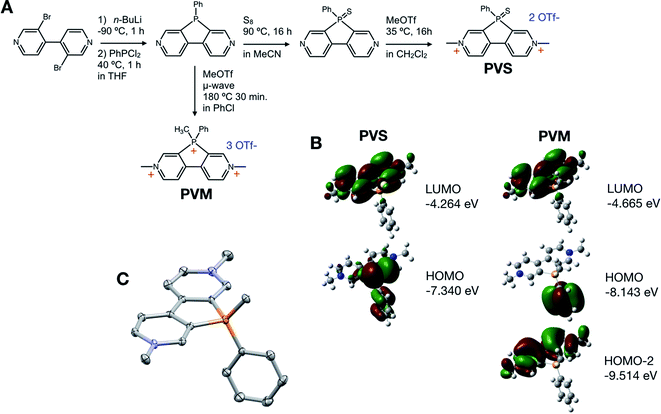 |
| Fig. 1 (A) Synthesis of phosphoryl-bridged viologens accepting two electrons (PVS) and three electrons (PVM). (B) Relevant orbitals and energy levels. Solid-state structure for PVM (C); H atoms and anions omitted for clarity. Energy levels were calculated from optimized structures at a B3LYP/6-31+g(d) level of theory (PCM solvation applied). | |
Cyclic voltammetry (CV) shows that PVS exhibits two one-electron reductions occurring at −0.1 V and −0.5 V vs. Ag/AgCl (Fig. 2A), i.e., −0.6 V and −1.0 V vs. Fc/Fc+, similar to the reduction potentials of PVO.12PVM exhibits two reduction waves at +0.2 and −0.3 V vs. Ag/AgCl (−0.3 V and −0.8 V vs. Fc/Fc+), with the second reduction showing a higher peak current. Three reductions are expected, and the higher peak current indicates there are multiple reductions occurring around −0.3 V vs. Ag/AgCl (−0.8 V vs. Fc/Fc+). Differential pulse voltammetry (DPV) shows the second reduction wave integrates to twice the current of the first reduction wave, proving it is a two-electron redox reaction (Fig. 2B and C). We tentatively assign the first reduction in PVM to the quaternary phosphorus center since it occurs at a higher potential than is expected for viologens. The reduction potentials of viologen derivatives are typically separated by 100–500 mV. In the case of PVM, slower scan rates and DPV could not resolve the second and third reductions, leaving them as a single peak. CV analysis of the electron-transfer rate reveals the two-electron reduction has a single, fast electron-transfer rate (see the ESI†). Viologens are known to undergo dimerization and disproportionation to form the fully reduced species from the radical cation.17,19
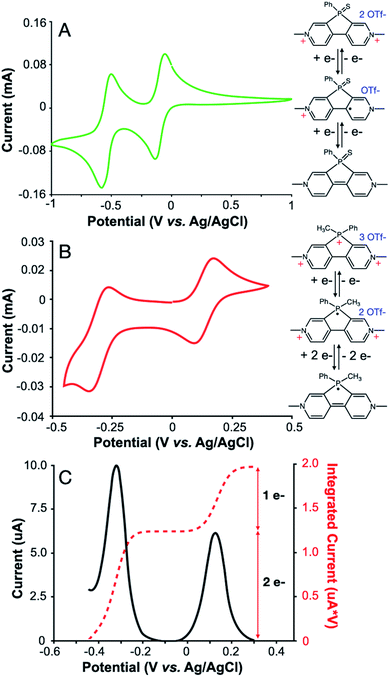 |
| Fig. 2 (A) CV and redox reactions of PVS. (B) CV and redox reactions of PVM. (C) DPV on PVM showing the current (black) and integrated current (red) for each reduction. All experiments were conducted in a 0.1 M TBAPF6/acetonitrile electrolyte under inert atmosphere using a glassy carbon working, Pt wire counter, and Ag/AgCl reference electrodes. | |
Some π-extended viologens, reported in the literature, also only exhibit one reduction wave for both steps (or two very closely spaced one-electron reductions), which is attributed the instability of the reduced radical cation species.20 We propose that the PVM biradical cation rapidly disproportionates to form the triply-reduced radical and radical dication, causing the redox peaks for the second and third reduction to merge. This may be attributed to the strong propensity for biradical species to dimerize and to the higher concentration of PVM at the electrode surface, making dimerization more favorable compared to more dilute optical experiments (see the ESI†). Regardless of the origin of the two viologen reductions merging, PVM accepts three electrons, with the first reduction occurring at +0.2 V vs. Ag/AgCl (−0.3 V vs. Fc/Fc+) on the quaternary phosphorus and the second and third reductions occurring on the viologen core at −0.3 V vs. Ag/AgCl (−0.8 V vs. Fc/Fc+). These reductions occur between 3–3.6 V vs. Li/Li+ and show that PVM is a unique three-electron accepting scaffold. The peak to peak distance for all reductions on PVS and PVM is between 40–75 mV/n as measured by CV, indicating these reductions are highly reversible and generally suitable for battery applications (see the ESI†).
The three redox states of PVS and four redox states of PVM can be observed using chemical reductions. The colorless PVS dication is reduced to the radical cation using Zn powder, forming a dark blue solution. Doubly reduced neutral PVS is formed via reduction with Li metal, forming an orange solution (Fig. 3A). The colorless trication of PVM is converted to the singly reduced radical dication by reduction with Cu(I)Br to form a pale-yellow solution. The second and third reduction of PVM occur in a stepwise manner when using Zn as a reductant. The doubly reduced biradical cation is first formed, yielding a purple solution, followed by the triply reduced neutral radical to give a yellow solution (Fig. 3B). All redox species for PVS and PVM are observed using spectroelectrochemistry, exhibiting the same absorptions (see the ESI†). The high-energy absorbances of the PVM radical dication and neutral radical support our assignment of a localized radical centered on the phospholium ring rather than a delocalized radical on the viologen core. TD-DFT calculations support the assignment of these radical absorptions, showing the PVM biradical cation and PVS radical cation having strong absorptions in the 600 nm range, and the PVM radical dication and neutral radical having strong absorptions in the 300–400 nm range (see the ESI†).
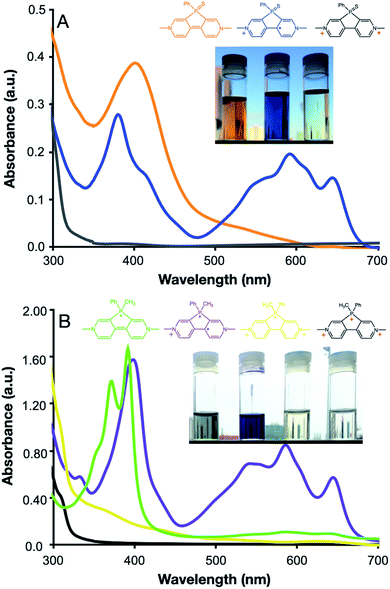 |
| Fig. 3 (A) Optical absorbance of the various redox states of PVS isolated using chemical reductions, and photographs of their solutions (inset). (B) Optical absorbance of the various redox states of PVM isolated using chemical reductions and photos of their solutions (inset). Chemical reductions were performed in a glove box in dilute acetonitrile solutions. | |
PVS exhibits one EPR spectrum for its radical cation that closely matches the splitting pattern for radical cations of phosphaviologen oxides (Fig. 4A).12PVM exhibits three distinct EPR spectra for its three radical redox states (Fig. 4B–D). In all cases, the simulated spectra closely match the experimental EPR spectra (Fig. 4). These calculations show that PVS holds the majority of its spin density on the viologen core, whereas PVM radicals show significant spin density around the phosphorus-centered ring. The radical dication and neutral radical also hold significant spin density on the phenyl and methyl functional groups on the phosphorus center, further indicating the importance of the phosphonium cation in allowing the unique tricationic viologen to stably accept three electrons (see the ESI†).
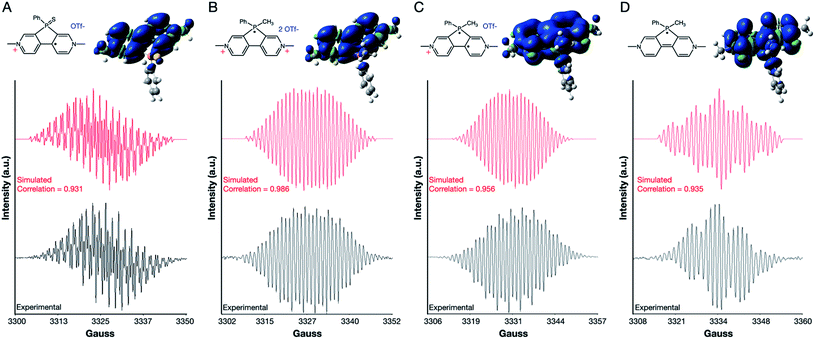 |
| Fig. 4 Spin density maps, EPR spectra, and simulated EPR spectra for the isolated radicals of PVS (A), and PVM (B–D). Spin density maps and hyperfine coupling constants were calculated at a B3LYP/6-31+g(d) level of theory. Simulated EPR spectra were optimized using Winsim. | |
Phosphaviologen oxide (PVO) derivatives function as cathode in high-voltage batteries with a lithium anode, having charging and discharging plateaus between 2.8–3.2 V vs. Li/Li+.16,17 To explore how PVM functions as an organic energy-storage material we fabricated batteries having a Li anode and PVM cathode (which would correspond to a dual-ion battery type in a full cell setup).21 An electrolyte solution saturated in lithium triflate was used to minimize the solubility of PVMvia the common ion effect. The battery was charged and discharged between 2–4.5 V vs. Li/Li+ at various scan rates, showing moderate shoulders between 3–4 V vs. Li/Li+ due to the redox reactions of PVM (Fig. 5). The cell exhibited good cycle stability, with negligible capacity fading after 120 charge/discharge cycles. The observed capacity is only roughly 30% of the theoretical capacity, indicating most of the electrode material is dissolved in the electrolyte solution.15 While this result is somewhat disappointing it nevertheless provides an important proof-of-concept for the utility of PVM as a high voltage cathode material. Alternative synthetic strategies to reduce the solubility of PVM are currently being explored to allow the intrinsic high voltage and high capacity of these novel materials to be achieved.
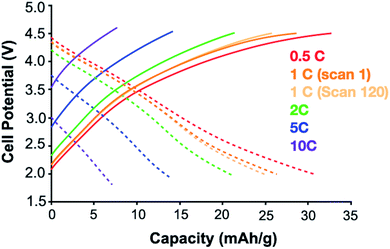 |
| Fig. 5 Charge–discharge profiles at varying C-rates for Li-ion batteries having a PVM cathode. Coin-cell batteries were tested using a lithium reference and a saturated LiPF6/TEGDME electrolyte. Specific capacity is calculated including the mass of a triflate counterion. | |
Conclusions
In conclusion, we have synthesized two new small multiple-electron acceptor molecules having high reduction potentials versus Li, a common battery anode metal. PVS accepts two electrons delocalized across the viologen core, while PVM accepts one electron localized on the phosphorus-centered ring, and two additional electrons delocalized across the viologen core between 3.0–3.6 V vs. Li/Li+. PVM is a particularly promising energy-storage material being a rare example of a small molecule able to accept three electrons at high voltages vs. Li/Li+. Li-ion batteries fabricated using PVM as the cathode exhibit a high voltage, but the high solubility of PVM decreases the overall capacity and lifetime of the battery. Efforts to modify the PVM scaffold to reduce its solubility are ongoing.
Conflicts of interest
There are no conflicts to declare.
Acknowledgements
Financial support by the Natural Sciences and Engineering Research Council of Canada and the Canada Foundation for Innovation is gratefully acknowledged. C. R. B. would like to acknowledge a York Science Fellowship, and T. B. thanks the Canada Research Chairs program for support.
References
- B. C. Melot and J. M. Tarascon, Acc. Chem. Res., 2013, 46, 1226–1238 Search PubMed.
- T. B. Schon, B. T. McAllister, P.-F. Li and D. S. Seferos, Chem. Soc. Rev., 2016, 45, 6345–6406 Search PubMed.
- S. Muench, A. Wild, C. Friebe, B. Häupler, T. Janoschka and U. S. Schubert, Chem. Rev., 2016, 116, 9438–9484 Search PubMed.
- L. Chen, C. R. Bridges, G. Gao, T. Baumgartner and X. He, ACS Appl. Energy Mater., 2019, 2, 7315–7320 Search PubMed.
- G. Li, B. Zhang, J. Wang, H. Zhao, W. Ma, L. Xu, W. Zhang, K. Zhou, Y. Du and G. He, Angew. Chem., Int. Ed., 2019, 58, 8468–8473 Search PubMed.
- A. Jouhara, E. Quarez, F. Dolhem, M. Armand, N. Dupré and P. Poizot, Angew. Chem., Int. Ed., 2019, 58, 15680–15684 Search PubMed.
- H. Wang, S. Yuan, D. Ma, X. Huang, F.-L. Meng and X.-B. Zhang, Adv. Energy Mater., 2014, 4, 1301651–1301657 Search PubMed.
- A. Iordache, V. Delhorbe, M. Bardet, L. Dubois, T. Gutel and L. Picard, ACS Appl. Mater. Interfaces, 2016, 8, 22762–22767 Search PubMed.
- N. Patil, A. Aqil, F. Ouhib, S. Admassie, O. Inganäs, C. Jérôme and C. Detrembleur, Adv. Mater., 2017, 29, 1703373–1703379 Search PubMed.
- H. Wu, S. A. Shevlin, Q. Meng, W. Guo, Y. Meng, K. Lu, Z. Wei and Z. Guo, Adv. Mater., 2014, 26, 3338–3343 Search PubMed.
- T. B. Schon, A. J. Tilley, C. R. Bridges, M. B. Miltenburg and D. S. Seferos, Adv. Funct. Mater., 2016, 26, 6896–6903 Search PubMed.
- M. Stolar, J. Borau-Garcia, M. Toonen and T. Baumgartner, J. Am. Chem. Soc., 2015, 137, 3366–3371 Search PubMed.
- S. Durben and T. Baumgartner, Angew. Chem., Int. Ed., 2011, 50, 7948–7952 Search PubMed.
- C. R. Bridges, M. Stolar and T. Baumgartner, Batteries Supercaps, 2020, 3, 268–274 Search PubMed.
- M. Stolar, C. Reus and T. Baumgartner, Adv. Energy Mater., 2016, 6, 1600944–1600949 Search PubMed.
- L. Striepe and T. Baumgartner, Chem.–Eur. J., 2017, 15, 16924–16940 Search PubMed.
- C. L. Bird and A. T. Kuhn, Chem. Soc. Rev., 1981, 10, 49–82 Search PubMed.
- T. Fujii, S. Tanaka, S. Hayashi, H. Imoto and K. Naka, Chem. Commun., 2020, 56, 6035–6038 Search PubMed.
- J. W. Park, N. H. Choi and J. H. Kim, J. Phys. Chem., 1996, 100, 769–774 Search PubMed.
- M. E. Alberto, B. C. De Simone, S. Cospito, D. Imbardelli, L. Veltri, G. Chidichimo and N. Russo, Chem. Phys. Lett., 2012, 552, 141–145 Search PubMed.
- M. Wang and Y. Tang, Adv. Energy Mater., 2018, 8, 1703320 Search PubMed.
Footnote |
† Electronic supplementary information (ESI) available: Experimental procedures, materials, equipment, additional electrochemical characterization of PVS and PVM, battery characterization, optical absorbance of unreduced PVS and PVM, calculated energy levels for all redox states of PVS and PVM and their Cartesian coordinates, EPR simulation parameters, and excitation energies, 1H-NMR, 13C{1H}-NMR, 19F{1H}-NMR, and 31P{1H}-NMR spectra, and single crystal X-ray crystallography data for PVM. CCDC 2018920. For ESI and crystallographic data in CIF or other electronic format see DOI: 10.1039/d0sc04183a |
|
This journal is © The Royal Society of Chemistry 2020 |