DOI:
10.1039/D0SC00844C
(Edge Article)
Chem. Sci., 2020,
11, 4397-4402
A pH ratiometrically responsive surface enhanced resonance Raman scattering probe for tumor acidic margin delineation and image-guided surgery†
Received
12th February 2020
, Accepted 30th March 2020
First published on 7th April 2020
Abstract
Surgery remains the mainstay for most solid tumor treatments. However, surgeons face challenges in intra-operatively identifying invasive tumor margins due to their infiltrative nature. Incomplete excision usually leads to early recurrence, while aggressive resection may injure adjacent functional tissues. Herein, we report a pH responsive ratiometric surface-enhanced Raman scattering (SERRS) probe that determined physiological pHs with a high sensitivity and tissue penetration depth via an innovative mechanism named spatial orientation induced intramolecular energy transfer (SOIET). Due to the positive correlation between tumor acidity and malignancy, an acidic margin-guided surgery strategy was implemented in live animal models by intra-operatively assessing tissue pH/malignancy of the suspicious tissues in tumor cutting edges. This surgery remarkably extended the survival of animal models and minimized their post-surgical complications, showing promise in precisely identifying invasive tumor boundaries and achieving a balance between maximum tumor debulking and minimal functional impairment.
Introduction
Surgery is a mainstream treatment for solid tumors. The goal for infiltrative tumor surgery is the maximal resection of the tumor tissue because the extent of resection is positively proportional to patient survival.1 However, the infiltrative regions developed by the invasion of cancer cells along the micronerves and capillaries are difficult to identify intraoperatively. Therefore, the precise delineation of tumor infiltrative territories remains a pivotal concern in improving surgical prognosis.
Clinically used techniques such as neuro-navigation and intra-operative magnetic resonance imaging (iMRI) rely on paramagnetic gadolinium chelators for delineating the tumor margin. However, this margin actually presents the territory with disrupted vasculatures instead of the actual infiltrative region leading to tumor recurrence. Tumor associated metabolites present the proliferative, invasive and metastatic natures of cancer cells. The intratumoral choline (Cho) to N-acetyl aspartate (NAA) ratio determined by magnetic resonance spectroscopy (MRS) had been used in clinics to evaluate malignancies of primary central nervous system lymphoma and the brain tumor.2 However, the translation of Cho/NAA ratio guided surgery is hindered by the low spatial resolution and extended acquisition time of MRS.3 Nevertheless, intra-operative imaging of tumor associated metabolites shows promise in guiding tumor resection. The metabolic switch from oxidative phosphorylation to aerobic glycolysis (Warburg effect) in cancer cells results in the acidification of the tumor extracellular microenvironment (pH 6.2–6.9).4 Gillies et al. found that the peritumoral regions with the highest invasiveness corresponded to the areas with the lowest extracellular pH (pHe).5 Gertler et al. showed that acidification in tumor boundaries drove transcriptome dynamics to promote the acquisition of invasive phenotypes.6 Therefore, intra-operative determination of acidities in tumor cutting edges holds promise in locating and excising the infiltrative region with high malignancy.
Raman imaging is an optical spectroscopic technology that describes the chemical composition of samples by monitoring the inelastic scattering generated from the interaction between the incident light and virtual energy states of the sample.7 The Raman signal could be massively enhanced by 108 to 1012 fold via a surface-enhanced Raman scattering (SERS) effect.8 Compared with fluorescence imaging, SERS shows higher photo-stability, higher sensitivity and multiplexing potential for the detection of up to ten targeting compounds simultaneously.9,10 Multi-modality or ultra-sensitive SERS probes have been reported to outline tumor infiltrative margins and microscopic malignant foci.11,12 However, these probes visualizing tumor margins via the enhanced permeability and retention (EPR) effect cannot fully delineate tumor invasive regions with high acidity.13 A few pH-responsive SERS probes were reported to define tumors by sensing their acidic environments,14 but their intensity-dependent readout is easily interrupted by uneven probe concentrations induced by tumor heterogenicity, resulting in erroneous detection readouts. There has been growing interest in ratiometric probes that acquire quantitative information for the analyte via built-in self-calibration.15 By this method, the artifical errors caused by heterogeneous probe difussion and distribution could be minimized. Therefore, the pH ratiometrically responsive SERS probe holds promise in locating tumor infiltrative regions by quantitatively determining the pHs/malignancies in tumor cutting edges.
In this work, a novel ratiometric SERRS design strategy was reported and a pH ratiometrically responsive SERRS probe AuS-IR7p was developed, which independently determined pH values with high sensitivity, accuracy and reversibility. With assistance of this probe, an acidic margin-guided tumor resection surgery strategy was proposed by intra-operatively assessing tissue malignancies in live animal models via real-time determination of pH values (Fig. 1A). Furthermore, surgical prognosis was dynamically evaluated by non-invasive contrast enhanced MRI (CE-MRI). The acidic margin-guided surgery strategy using AuS-IR7p shows promise in identifying invasive glioma boundaries and achieving a balance between maximum tumor debulking and minimal functional impairment.
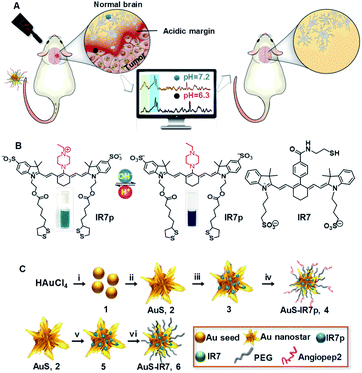 |
| Fig. 1 Design and synthesis of the pH ratiometrically responsive SERRS probe. (A) Illustration presenting the acidic margin-guided brain tumor surgery by intra-operatively determining tissue pH values/malignancies in tumor cutting edges. (B) Chemical structure of the pH-responsive reporter molecule IR7p and the control reporter IR7 without pH sensitivity. (C) Synthesis of pH responsive SERRS probe AuS-IR7p and the control probe AuS-IR7. (i) Sodium citrate; (ii) ascorbic acid, AgNO3; (iii) IR7p; (iv) HS-PEG5K-NHS, cysteine-modified angiopep2 peptide; (v) IR7; (vi) HS-PEG5K-OMe. | |
Results and discussion
Synthesis and characterization of AuS-IR7p
Gold nanostars were chosen as the substrate for the SERS probe due to the followingreasons. First, numerous sharp tips on the probe surface can trigger 2–3 orders of magnitude extra signal enhancement due to the hotspot effect.16 Second, the overlapping between the plasmon absorption of gold nanostars (700–800 nm) and the energy of the incident laser (785 nm) remarkably increases the signal intensity.17 Heptamethine cyanine derivative IR7 was chosen as a Raman molecular reporter because its absorption maxima (760–790 nm) matches with that of the incident laser (785 nm), which triggers the surface enhanced resonance Raman scattering (SERRS) effect18 and leads to extra 1–3 orders of magnitude signal enhancement compared to non-resonance SERS. Additionally, the probe surface was modified with angiopep2 peptides to facilitate its brain delivery by traversing the BBB via low-density lipoprotein-receptor-related protein-1 (LRP1) mediated receptor-mediated endocytosis (RMT).19
The synthesis of the pH ratiometrically responsive SERRS probe AuS-IR7p is presented in Fig. 1B and C. Briefly, the reaction between citrate and chloroauric acid yielded spherical gold seeds 1 with an average diameter of 12 nm. The asymmetric growth of the gold seeds in a mixture of chloroauric acid, ascorbic acid and AgNO3 offered gold nanostars AuS (2) possessing multiple outward gold spikes.20 The surface modification of AuS with IR7p through the Au–S covalent bonds gave nanoparticles 3. Similarly, fabrication of HS-PEG5K-OMe and angiopep2-PEG-SH on 3 with a molar ratio of 10
:
1 offered the final product AuS-IR7p (4). Meanwhile, control SERRS probe AuS-IR7 (6) was prepared by surface modification of AuS with molecule reporter IR7 that has a similar chemical structure to IR7p but without the pH sensitivity. Transmission electron microscopy (TEM) images showed AuS-IR7p dispersed with an average diameter of 50 nm (Fig. 2A). Dynamic light scattering (DLS) determined the average hydrodynamic particle size of AuS-IR7p to be 132.0 nm and its zeta potential to be 8.2 mV (Fig. 2B). In vitro confocal Raman imaging studies demonstrated the acidity triggered SERRS signal enhancement of AuS-IR7p and an extremely low detection limit of 0.8 pM was observed at pH 5.5 (Fig. 2C and D). Notably, AuS-IR7p showed a high tissue penetration depth and its Raman signal was detected even when covered by mouse brain tissue with a thickness of 2.2 mm (Fig. 2E). AuS-IR7p could reversibly monitor the pH variation in the physiological range even after four switching cycles from pH 3 to pH 8 (Fig. S1†). Besides, the average hydrodynamic particle size remained around 130 nm after four pH switching cycles, which verifies the minimized self-aggregation of this SERRS probe (Fig. 2F). AuS-IR7p showed minimized cytotoxicity after culturing with microglial, neuronal and endothelial cell lines (Fig. S2 and S3†).
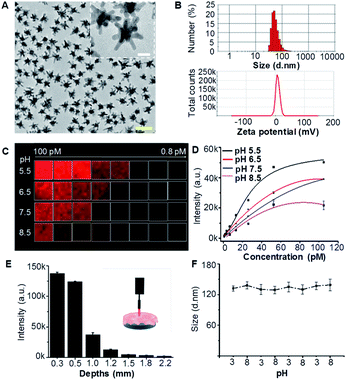 |
| Fig. 2 Characterization of AuS-IR7p. (A) TEM image of AuS-IR7p. Scale bar = 100 nm. Inset: amplified gold nanostars. Scale bar = 20 nm. (B) Hydrodynamic diameter distribution and zeta potentials of AuS-IR7p in PBS solution (pH 7.4). (C) Raman microscopy image of AuS-IR7p as functions of concentrations and pH values by quantifying its characteristic peak at 510 cm−1. (D) Concentration dependent Raman signal intensities of AuS-IR7p in buffered solutions with different pH values. (E) Raman signal intensities of AuS-IR7p as a function of the thickness of covered mouse brain tissue (200 mW laser power density, 1.0 s acquirement time, and 10× objective). Data are presented as mean ± S.D. (n = 3). (F) AuS-IR7p shows reversible pH responsive ability. The initial concentration was 100 pM. Laser power: 80 mW. Acquirement time: 1.0 s. | |
pH ratiometric responses of AuS-IR7p
Raman reporter molecule IR7p demonstrated pH-responsive absorbance in the near-infrared (NIR) wavelength range (Fig. S4†). Obvious bathochromic shift with a new peak centered at 770 nm was monitored when the pH decreased to 2.0 from 8.0. Protonation of nitrogen in the ethylpiperazidine group results in a decreased intramolecular energy gap and thus produces the bathochromic shift ofabsorption. As expected, the absorbance of IR7 remained unchanged from pH 8.0 to pH 3.0 (Fig. S5†). Fig. 3A shows the Raman spectra of AuS-IR7p as a function of pH after excitation at 785 nm. The peak at 311 cm−1 could be assigned to the Au–S stretching and bending. The sharp twin peaks at 527 and 558 cm−1 could be attributed to the ring deformation of the methylene carbon chain. The bands at 784 and 927 cm−1 represent C–H out-of-plane bending mode and C–C symmetric stretching mode of the methylene carbon chain. A peak at 1511 cm−1 could be assigned to the C
O stretching in ester bonds, and a peak at 1199 cm−1 could be assigned to the C–N stretching in the ethylpiperazine ring. Interestingly, while most of the Raman signatures increased gradually upon acidification, the peak at 311 cm−1 (highlighted as Peak 1) remained steady. Notably, the Raman intensity ratio between the twin peak (highlighted as Peak 2) and Peak 1 increased from 1.5 to 5.0 when the pH decreased from 8.0 to 2.0. Plotting the Peak 2/Peak 1 ratio against the pH value offered a pKa value of 4.6 for AuS-IR7p (Fig. 3B). Confocal Raman spectroscopic images of AuS-IR7p showed that while the integrated intensity of Peak 2 increased with acidification, the intensity of Peak 1 remained stable (Fig. S6†). Significantly, the pH values determined by AuS-IR7p (100 pM) coincide well with the value measured using a pH meter, especially in the range of pH 5.0–8.0 (Fig. S7†).
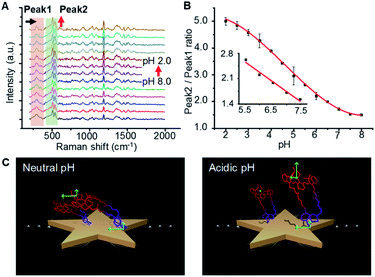 |
| Fig. 3
AuS-IR7p determines pH values via ratiometrically responsive Raman signals. (A) pH dependent Raman spectra of AuS-IR7p. The pH changed with an interval of 0.5 units. (B) Plot of integrated intensity ratios between Raman Peak 2 (450–595 cm−1) and Peak 1 (280–370 cm−1) as a function of pH. The inset shows measurements in the linear response region. (C) Proposed mechanism illustrating the pH ratiometric response of AuS-IR7p. Unprotonated IR7p shows parallel conformation relative to the metallic surface (left panel) but titled or even perpendicular conformation under acidic conditions (right panel) due to the intermolecular electrostatic repulsion triggered by the protonation of reporter molecule IR7p. | |
Three ratiometric SERS strategies had been reported to quantitatively determine the analyte concentration in vivo. The first is simultaneous injection of two probes in which one acts as a control to offset the nonspecific accumulation and the other is applied for analyte sensing.21 The second is the incorporation of two or more types of Raman reporters into a particle, in which one inert reporter serves as an internal reference and the others are used for analyte determination.22 The third is conjugation of a single reporter molecule being functionalized as both an analyte sensor and a self-built-in calibrator. As far as we are aware, AuS-IR7p is the first Raman probe independently determining physiological pH values with a signal reporter molecule. By this method, the measurement errors induced by the diverse pharmacokinetics of the different probes in the first and second strategies could be minimized. The mechanism of ratiometric responsiveness of AuS-IR7p was defined as a spatial orientation induced intramolecular energy transfer (SOIET) (Fig. 3C). The SERRS efficiency is highly dependent on the distance between the reporter molecule and metallic surface (ISERRS ∼ r−12). Protonation of the reporter molecule IR7p increases the intermolecular electrostatic repulsion and triggers their orientations from flat to tilted or even perpendicular attitudes relative to the metallic surface. Vibration bonds in the reporter molecule backbone experienced a much more increased perpendicular polarizability tensor upon the electromagnetic field than Au–S bonds that directly conjugated on the metallic surface.
Acidic margin guided brain tumor resection in live rat models with AuS-IR7p
Acidic margin-guided surgery was conducted in live rat models bearing orthotopic C6 glioblastoma allografts with high invasiveness. In vivo T2W MR images showed the tumor with an average volume of 60–95 mm3 (Fig. 4A). The In vivo pH map generated by chemical exchange saturation transfer (CEST)-MRI demonstrated the pronounced acidosis in the tumor but barely in the normal brain (Fig. 4B). The average pH value of 6.6 was measured in the tumor in comparison to 7.2 in the normal brain. Craniotomy was conducted in the right frontoparietal region (Fig. 4C). Animal models were randomly divided into three groups: (1) intra-operative acidic margin (iAM) guided surgery after intravenous (i.v.) injection of AuS-IR7p, (2) intra-operative structural margin (iSM) guided surgery after i.v. injection of AuS-IR7 and (3) preoperative structural margin (pSM) guided surgery after i.v. injection of Gd3+-DTPA. A handheld Raman spectrometer was used to intra-operatively collect the Raman signal due to its capability to scan the operation bed with high flexibility and velocity. Additionally, with assistance of home-made software that automatically identifies the characteristic Raman peaks and converts the Peak 2/Peak 1 ratio into a pH value, the acidity of a suspicious tissue spot can be obtained in 5 s. In the iAM-guided surgery group, the acidosis distribution pattern at tumor cutting edges was presented as a 3D pixel block map, in which each pixel block presented the pH value of the measured point (1 × 1 × 1 mm). Guided by the pH map, the acidic tissues were excised step by step until no foci with pH below 7.0 could be detected (Fig. 4D). In the iSM-guided group, the tissues detected with characteristic Raman signals of AuS-IR7 were excised continuously until no signal in the operation bed was identified. In the pSM-guided group, the tumor was resected according to the margin delineated by the pre-operative MRI.
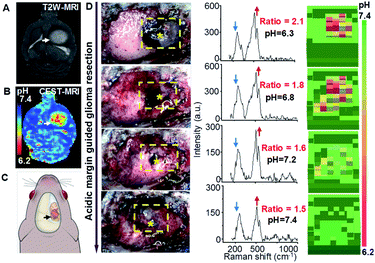 |
| Fig. 4 Acidic margin-guided brain tumor surgery in live rat models. (A) Preoperative T2W-MR image of rat brain bearing C6 orthotopic allografts. The tumor was indicated by a white arrow. (B) In vivo CEST-pH map of a rat brain model. (C) Illustration indicating the surgical location before the craniotomy. (D) Sequential white light images (left panel), intra-operatively collected Raman spectra (middle panel) and a pH topographic map (right panel) of the resection bed. Yellow dashed boxes marked the area with detectable SERRS signals and star symbols presented the points with the highest acidity. | |
Acidic margin guided surgery improving surgical prognosis
Fig. 5A presents in vivo MR images of mouse brains before and on selected days post the above intervention. In the pSM-guided group, rapid tumor relapse was observed as early as 3 days post-surgery and the recurrent neoplastic tissue was observed in all models on day 15. In contrast, obvious tumor recurrences were not detected on day 15 post iAM- or iSM-guided groups (Fig. S8A†). The Kaplan–Meier survival curve showed that all models in the pSM-guided group died before day 16 (Fig. 5B), which was explained by the incomplete tumor resection. For the iSM-guided group, one third of the models died within three days, which resulted from functional disabilities and another one-third died gradually with concomitant tumor recurrence. Remarkably, no animal death was monitored until 21 days post the iAM-guided surgery and one-third of the animals survived more than 90 days without tumor recurrence. The iAM-guided group also showed the highest post-operative recovery (Fig. S8B†). Neurological Assessment (NA) quantitatively determined the neurological functional deficits with respect to reflexes (normal score: 0, maximal deficit score: 21) (Fig. 5C). Although all three groups showed their maximal NA scores on day 3, the NA score of the iAM-guided group remained the lowest.
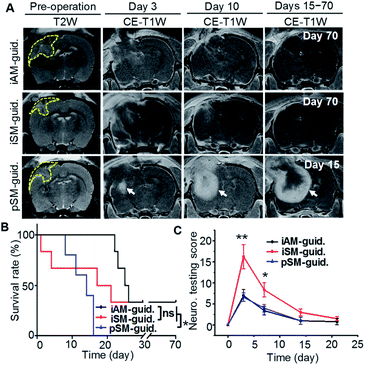 |
| Fig. 5 Intra-operative acidic margin-guided surgery improves surgical prognosis and minimizes functional deficits. (A) In vivo MR images of a rat brain bearing C6 rat orthotopic allografts before and at selected days post iAM-guided, iSM-guided and pSM-guided surgery. Tumors with hyper-intensive signals in the preoperative T2W-MR images were delineated by yellow dotted lines. The recurrent tumor was indicated by a white arrow. Kaplan–Meier survival (B) and neurological testing scores (C) after corresponding surgical intervention. Data were presented as mean ± S.D. (n = 5). **p < 0.01 between the iAM-, iSM-guided strategy and pSM-guided surgery. ns, no significant difference. In panel E, **p < 0.01 and *p < 0.05 between the iAM- and iSM-guided groups on day 3 and 7. iAM-guid.: intra-operative acidic margin guided surgery; iSM-guid.: intra-operative structure margin guided surgery; pSM-guid.: preoperative structural margin guided surgery. CE-T1W: contrast enhanced T1-weighted. | |
Conclusions
In conclusion, by developing a pH ratiometrically responsive SERRS probe, we first propose an acidic margin-guided surgery strategy, which remarkably extends the overall survival of animal models and minimizes postoperative complications. Considering the correlation between the acidity and malignancy in tumor tissues, this strategy will help surgeons to demarcate tumor infiltrated regions that are usually “invisible” in the clinically used surgical navigation systems and achieve a balance between maximum tumor debulking and minimal functional impairment. Additionally, this acidic margin-guided surgery strategy holds promise in the treatment of different types of infiltrated tumors because extracellular acidification is a hallmark of solid tumors regardless of their genotypes or phenotypes.
Ethical statement
All animal studies were performed in compliance with the guidelines set by the Chinese Committee of Management of Laboratory Animals and the overall project protocols were approved by the Ethics Committee of Fudan University.
Conflicts of interest
There are no conflicts to declare.
Acknowledgements
This work was supported by the National Natural Science Foundation of China (No. 81771895 to C. L.; 81572483 and 81611130223 to Y. M.; 81873893 to X. Z.; 81602178 to Q. Y.; 81671753 to Z. W.; 81771890 and 91859106 to R. H.), the Program of Shanghai Science and Technology Committee (No. 19431900400 and 18441900600 to C. L., 13411950201 and 16JC1420100 to Y. M.), the Fudan-SIMM Joint Research Fund (No. 20173001 to C. L. and R. H.), the One Hundred Talent Program of the Chinese Academy of Sciences (to R. H.), the Shanghai Municipal Science and Technology Major Project (No. 2018SHZDZX01 to J. F. and X. Z., 2018SHZDZX03 to Y. M.), the 111 Project (No. B18015 to J. F.) and the Zhangjiang Lab.
Notes and references
- M. G. Matthew, F. R. Pablo, S. N. Amy, L. S. Jason, A. Lilyana, H. B. Gene and A. V. Michael, J. Neurosurg., 2014, 121, 1115–1123 Search PubMed.
- M. Bulik, R. Jancalek, J. Vanicek, A. Skoch and M. Mechl, Clin. Neurol. Neurosurg., 2013, 115, 146–153 CrossRef PubMed.
- J. Zhang, D. X. Zhuang, C. J. Yao, C. P. Lin, T. L. Wang, Z. Y. Qin and J. S. Wu, J. Neurosurg., 2016, 124, 1585–1593 Search PubMed.
- R. J. Gillies, Z. Liu and Z. Bhujwalla, Am. J. Physiol., 1994, 267 Search PubMed.
- V. Estrella, T. Chen, M. Lloyd, J. Wojtkowiak, H. H. Cornnell, A. Ibrahim-Hashim, K. Bailey, Y. Balagurunathan, J. M. Rothberg, B. F. Sloane, J. Johnson, R. A. Gatenby and R. J. Gillies, Cancer Res., 2013, 73, 1524 CrossRef CAS PubMed.
- N. Rohani, L. L. Hao, M. S. Alexis, B. A. Joughin, K. Krismer, M. N. Moufarrej, A. R. Soltis, D. A. Lauffenburger, M. B. Yaffe, C. B. Burge, S. N. Bhatia and F. B. Gertler, Cancer Res., 2019, 79, 1952–1966 CrossRef CAS PubMed.
- K. Kong, C. Kendall, N. Stone and I. Notingher, Adv. Drug Delivery Rev., 2015, 89, 121–134 CrossRef CAS PubMed.
- L. A. Lane, X. Qian and S. Nie, Chem. Rev., 2015, 115, 10489–10529 CrossRef CAS PubMed.
- C. L. Zavaleta, B. R. Smith, I. Walton, W. Doering, G. Davis, B. Shojaei, M. J. Natan and S. S. Gambhir, Proc. Natl. Acad. Sci. U. S. A., 2009, 106, 13511–13516 CrossRef CAS PubMed.
- Y. Qiu, Y. Zhang, M. Li, G. Chen, C. Fan, K. Cui, J.-B. Wan, A. Han, J. Ye and Z. Xiao, ACS Nano, 2018, 12, 7974–7985 CrossRef CAS PubMed.
- M. F. Kircher, A. de la Zerda, J. V. Jokerst, C. L. Zavaleta, P. J. Kempen, E. Mittra, K. Pitter, R. Huang, C. Campos, F. Habte, R. Sinclair, C. W. Brennan, I. K. Mellinghoff, E. C. Holland and S. S. Gambhir, Nat. Med., 2012, 18, 829–834 CrossRef CAS PubMed.
- H. Karabeber, R. M. Huang, P. Iacono, J. M. Samii, K. Pitter, E. C. Holland and M. F. Kircher, ACS Nano, 2014, 8, 9755–9766 CrossRef CAS PubMed.
- S. Harmsen, R. Huang, M. A. Wall, H. Karabeber, J. M. Samii, M. Spaliviero, J. R. White, S. Monette, R. O'Connor, K. L. Pitter, S. A. Sastra, M. Saborowski, E. C. Holland, S. Singer, K. P. Olive, S. W. Lowe, R. G. Blasberg and M. F. Kircher, Sci. Transl. Med., 2015, 7, 271ra277 Search PubMed.
- T. Zhao, G. Huang, Y. Li, S. Yang, S. Ramezani, Z. Lin, Y. Wang, X. Ma, Z. Zeng, M. Luo, E. de Boer, X. J. Xie, J. Thibodeaux, R. A. Brekken, X. Sun, B. D. Sumer and J. Gao, Nat. Biomed. Eng., 2016, 1, 0006 CrossRef PubMed.
- X. L. Huang, J. B. Song, B. C. Yung, X. H. Huang, Y. H. Xiong and X. Y. Chen, Chem. Soc. Rev., 2018, 47, 2873–2920 RSC.
- L. Rodríguez-Lorenzo, R. A. Álvarez-Puebla, I. Pastoriza-Santos, S. Mazzucco, O. Stéphan, M. Kociak, L. M. Liz-Marzán and F. J. García de Abajo, J. Am. Chem. Soc., 2009, 131, 4616–4618 CrossRef PubMed.
- H. Yuan, A. M. Fales, C. G. Khoury, J. Liu and T. Vo-Dinh, J. Raman Spectrosc., 2013, 44, 234–239 CrossRef CAS PubMed.
- S. Schlücker, Angew. Chem., Int. Ed., 2014, 53, 4756–4795 CrossRef PubMed.
- H. Yan, L. Wang, J. Wang, X. Weng, H. Lei, X. Wang, L. Jiang, J. Zhu, W. Lu, X. Wei and C. Li, ACS Nano, 2012, 6, 410–420 CrossRef CAS PubMed.
- L. Han, W. Duan, X. Li, C. Wang, Z. Jin, Y. Zhai, C. Cao, L. Chen, W. Xu, Y. Liu, Y. Y. Bi, J. Feng, Y. Mao, Q. Yue, X. Y. Zhang and C. Li, ACS Appl. Mater. Interfaces, 2019, 11, 15241–15250 CrossRef CAS PubMed.
- Y. W. Wang, N. P. Reder, S. Kang, A. K. Glaser, Q. Yang, M. A. Wall, S. H. Javid, S. M. Dintzis and J. T. C. Liu, Cancer Res., 2017, 77, 4506–4516 CrossRef CAS PubMed.
- S. Ye, X. Li, M. Wang and B. Tang, Anal. Chem., 2017, 89, 5124–5130 CrossRef CAS PubMed.
Footnotes |
† Electronic supplementary information (ESI) available. See DOI: 10.1039/d0sc00844c |
‡ These authors contributed equally to this work. |
|
This journal is © The Royal Society of Chemistry 2020 |
Click here to see how this site uses Cookies. View our privacy policy here.