DOI:
10.1039/C9SC05765J
(Edge Article)
Chem. Sci., 2020,
11, 1964-1974
The cross-talk modulation of excited state electron transfer to reduce the false negative background for high fidelity imaging in vivo†
Received
14th November 2019
, Accepted 20th December 2019
First published on 18th January 2020
Abstract
In practice, high fidelity fluorescence imaging in vivo faces many issues, for example: (1) the fluorescence background of the probe is bleached by the wide intensity scale of fluorescence microscopy, displaying an inherent false negative background (FNB); and (2) the dosage of the probe has to be increased to achieve sufficient intensity for in vivo imaging, causing a vicious cycle that exacerbates the FNB. Herein, we constructed a fluorophore (F)–electron donor (D)–electron regulator (R) system, and thereby developed a dual modulation strategy for the de novo design of high fidelity probes. Using cross-talk modulation, the probe allows: (1) enhanced ESET (excited state electron transfer) from F to D, which minimizes the inherent FNB based on synergistic PET (photo induced electron transfer); and (2) the inhibition of PET and weakening of ESET from F to D to maximize the reporting intensity to further reduce the FNB, which is additionally enhanced by an overdose of the probe. To test the implementation, we constructed a 7-hydroxy-2-oxo-2H-chromene-3-carbaldehyde (HPC) series of probes, with HPC (F) as the fluorophore, 2-hydrazinylpyridine, which was screened as an electronically adjustable donor (D), and electronic regulators (R). In particular, HPC-7 and HPC-8 provided cell/zebrafish imaging with negligible background even using the rather low fluorescence scale of microscopy (a region for revealing hidden background). Interestingly, with the specificity of HPC for reporting zinc, we achieved probe HPC-5, which possesses both an ultralow inherent FNB and optimal reporting intensity for tissue and in vivo imaging, enabling the in vivo imaging of zinc in mice for the first time. Under this high-fidelity mode, the fluorescence monitoring of zinc ions during the development of liver cancer in mice was successfully performed. We envision that the dual modulation strategy with the F–D–R system could provide a useful concept for the de novo design of practical probes.
Introduction
Molecular probes can exhibit a significant optical response towards analytes. Owing to their reactive specificity, timely reporting and biological affinity, molecular-probe-based fluorescence imaging techniques have been rapidly developed for biological monitoring, molecular medicine and even surgical navigation.1–10 However, in the actual imaging, there are several problems that are usually neglected: (1) inherent false negative background (FNB): although the background seems to be dark, the real background fluorescence will be exposed when the intensity scale of microscopy is compressed (Scheme 1A). Obviously, the real background is covered by the wide intensity scale of microscopy. This problem causes interference in imaging analysis, especially in cells or aquatic organisms. (2) Enhanced FNB owing to a vicious cycle: owing to the unsatisfactory reporting intensity, an increased dose of the probe is required, which unfortunately leads to a vicious cycle as higher dose give a stronger FNB. Inevitably, the imaging may carry the aggravated FNB, meaning that the accuracy and sensitivity will be reduced, especially for in vivo imaging. Therefore, although large numbers of excellent probes have been developed,11–14 developing a high fidelity probe to minimize FNB is of urgent need in practical analysis.
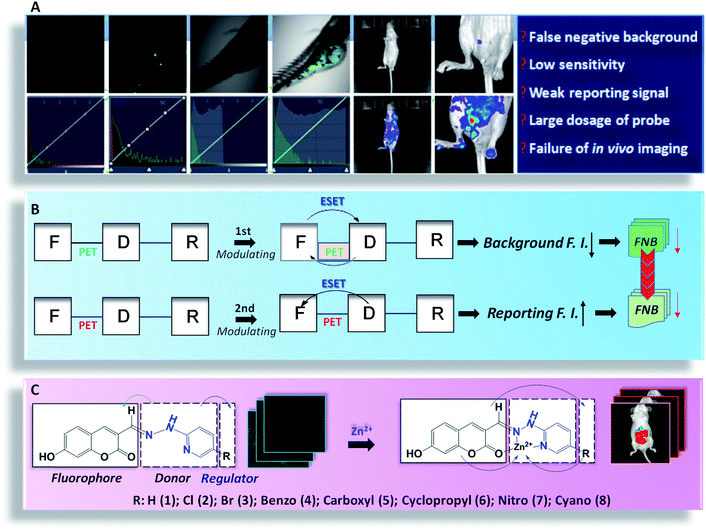 |
| Scheme 1 (A) Some challenging barriers for practical imaging. (B) The working principle of the fluorescent fluorophore (F)–electron donor (D)–electron regulator (R) system. (C) The de novo design of the HPC series of probes. | |
Over time, in the pursuit of accurate and sensitive imaging, researchers have developed many strategies,9,15–17 including electron transfer, structure rotation, hydrophilicity tuning, fluorescence assembly, and so forth. As far as the technique is concerned, whatever the mechanism features, the electron behavior should be the essential factor for controlling the optical properties. It is our belief that modulating the electron transfer with a single mechanism is limited and the excited state electron transfer (ESET) may be far beyond the control of a single factor. Thus, multiple factor cross-talk modulation may provide more preferable optical properties: (1) for light-penetrable samples, such as cells and aquatic organisms, the inherent false negative background fluorescence should be minimized; and (2) for samples with a poor transmittance, such as medical mice, the intensity of the reporting signal should be maximized, to reduce the dosage and thereby minimize the FNB. Therefore, developing the practical probe is a challenge, not only to improve the signal-to-noise ratio, but also to modulate the optical properties towards high fidelity imaging.
High fidelity imaging is critical for biomedical research, especially in tumor development. Zinc ions act as the essential contributor for tissue development.18 In malignant tumors, zinc has been suspected to be associated with the developmental stages.19–21 Although many excellent zinc probes have been developed,22–35 there is still no research on in vivo imaging of Zn ions, which limits deeper insights into tumor development.
Accordingly, we constructed a system of a fluorophore (F)–electron donor (D)–electron regulator (R), and established a dual modulation strategy for the de novo design of high-fidelity imaging probes based on the cross-talk effect of ESET and photo induced electron transfer (PET). F–D–R worked as follows: (1) ensuring PET for fluorescence quenching and adjusting R for enhanced ESET from F to D, to minimize the FNB; and (2) inhibiting PET for fluorescence restoration and decreasing the ESET from F to D, to enhance the fluorescence, thereby further minimizing the FNB. For the implementation, we synthesized 7-hydroxy-2-oxo-2H-chromene-3-carbaldehyde (HPC)-n series probes, consisting of the fluorophore 7-hydroxy-2-oxo-2H-tryptone-3-acetaldehyde (F), an adjustable electron-rich donor 2-hydrazinopyridine (D), as well as the electronic regulators (R). Benefitting from this strategy, the FNB fluorescence became negligible even when the intensity scale of microscopy was drastically compressed, which significantly improved the authenticity and accuracy, as well as the sensitivity. For the application, with the specific response of HPC-n to zinc, the accumulation of zinc during tumor development was visualized. We anticipate this strategy can be applied to de novo design to optimize fluorescent probes for practical use.
Results and discussion
De novo design of probes for cells and zebrafish imaging
We selected HPC to be the fluorophore as it is easily influenced owing to its rich electrons and small planar structure. Furthermore, if we can quench it effectively then we can achieve an ultra-strong response. At the beginning, two classical groups R2 (N1,N1-bis(pyridin-2-ylmethyl)ethane-1,2-diamine) (bpda) and R3 (1-(6-(aminomethyl)pyridin-2-yl)-N,N-bis(pyridin-2-methyl)methanamine) (Fig. 1) were linked to HPC as the ideal design. As expected, the resultant probes BPY and TPY exhibited an excellent response to zinc ions. Unfortunately, the fluorescence background of these are strong, and increases sharply with the rising concentration (Fig. 1). These unsatisfactory results drove us to investigate the mechanism (Fig. 4). The density functional theory (DFT) calculation shows that the highest occupied molecular orbital (HOMO) energies of the two electron donors R2 and R3 are both much higher than that of the fluorophore HPC, indicating their conformation to the PET principle. Thus, PET alone is not sufficient to completely quench the fluorescence. Moreover, cell imaging also revealed that even at a very low dosage (0.01 μM), we still observed a significant FNB (Fig. 3). Under the default ruler of the fluorescence intensity (BPY: left column), the cells did not show a background. However, darkness in the imaging picture does not mean no fluorescence, while the FNB may be concealed by the default ruler of intensity. In fact, when the ruler is compressed to the low region (BPY: right column), even at only half of the default ruler, the FNB became significant. Consequently, although BPY and TPY have already conformed to the PET mechanism, their quenching effects are limited. These results lead us to suppose that, in addition to the pre-designed PET, if another modulating mechanism was introduced, further reduction of the fluorescence may be possible. Therefore, we set out to construct a cross-talk electronic modulation of PET and ESET. Discarding the classical R2 and R3, we screened a moiety 2-hydrazinylpyridine (hpy) as it possessed both a high electron density and a planar conjugated structure for electron regulation. Firstly, we synthesized HPC-1 in order to perform a preliminarily test for such a concept. As Fig. 1 indicates, when the concentration is 1.0 μM, the fluorescence intensity is 50, which is actually lower than that of BPY and TPY at 0.01 μM, demonstrating its powerful effect in reducing FNB. When HPC-1 is reduced to 0.01 μM, the background intensity is rather low. These results indicate that introduction of the rich electron donor hpy into the fluorophore HPC is effective. Investigation of the electron configuration shows that, in HPC-1, the electrons of hpy are remarkably transferred to the orbital of HPC (Fig. 4B). In contrast, in BPY and TPY, the electronic contribution to HPC is not significant owing to their non-planar structures. Thus, it is promising to use hpy for effective multi-modulation of the electronic behaviour. However, although HPC-1 exhibited an excellent quenching effect, in the imaging analysis, the FNB emerged when the intensity scale was compressed (Fig. 3). Thus, we set out to artificially interfere with the ESET for further control of the fluorescence intensity owing to the uneven electron distribution of the electron. Actually, in BPY and TPY, we found that the enhanced fluorescence intensity correlated with the increased ESET from F to D (Fig. 4C). Unfortunately, owing to the non-conjugated structures of these two, it is difficult to modulate the ESET in BPY and TPY. While in hpy, we can construct the system of F–D–R to cross-talk interfere with the electron transfer. With this in mind, we designed a series of HPC-n probes. As shown in Fig. 4D, the HOMO energies of these three groups are higher than those of HPC, indicating that they have met the basic requirements of PET. As expected, their fluorescence intensity is significantly different (Fig. 2), HPC-7 and HPC-8 exhibit quite a weak intensity, while HPC-6 exhibits a very strong intensity, although it fully conforms to PET. Obviously, there is another electronic factor to give optical control in addition to PET. Then, we calculated the electronic distribution at the excited state to investigate the ESET. As indicated in Fig. 4C, HPC-7 exhibits an ESET of 0.1443e from HPC to hpy, while HPC-8 and HPC-6 exhibit the opposite ESET of 0.2372 and 0.4265, respectively. These results indicated, in the excited state, that the electron transfer from F to D regulated by R reduced the electron density on HPC, thereby weakening the fluorescence intensity. Moreover, the excited state molecular orbitals were studied. Fig. 4B showed that in HPC-7, a remarkable electron transfer from HPC to hpy was observed. As a result, the low electron density reduced the probability of the electronic transition, thereby leading to the weak fluorescence. Thus, cross-talk modulation of ESET and PET can be used to find an optimal probe. Then, we screened a series of groups as regulators and calculated their ESET (Fig. 4C). As can be seen, with the decreasing ESET from HPC to hpy, the fluorescence intensity decreased correspondingly. With this relationship, we can achieve an ultra-low background probe for cells and zebrafish imaging. As shown in Fig. 3, HPC-6 still brings about a strong false negative background under the wide intensity scale, even when diluted to 0.01 μM. In sharp contrast, 1 μM of HPC-7 and HPC-8 exhibit a quite low fluorescence background even when the images were shown under the rather low intensity scale. Therefore, HPC-7 and HPC-8 can be used to minimize the FNB for high fidelity imaging. As an additional benefit, the compressed scale of intensity may contribute to more sensitive imaging than the default mode, as the intensity difference in the microscopic view becomes smaller under the compressed scale of intensity.
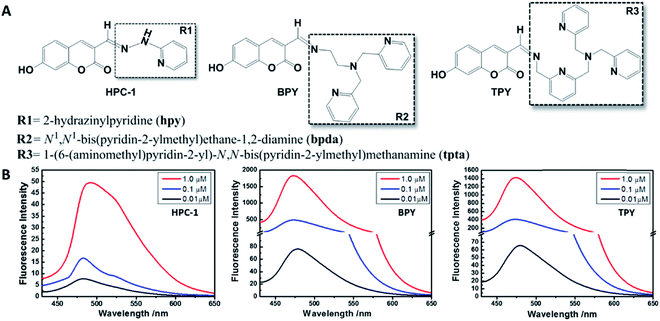 |
| Fig. 1 (A) The structures of HPC-1, BPY and TPY. (B) Intensities from fluorescence background experiments involving probes HPC-1, BPY and TPY (0.01, 0.1, and 1 μM). All experiments were performed in HEPES buffer solution (50 mM HEPES, 100 mM KNO3, pH = 7.2). | |
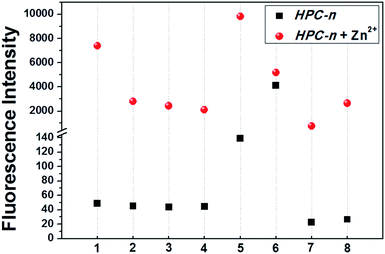 |
| Fig. 2 The intensities of the fluorescence background (black squares) and reporting experiment (red circles) signals for HPC-n (n: 1–8) (1 μM) in response to Zn ions (20 μM). All experiments were performed in HEPES buffer solution (50 mM HEPES, 100 mM KNO3, pH = 7.2). | |
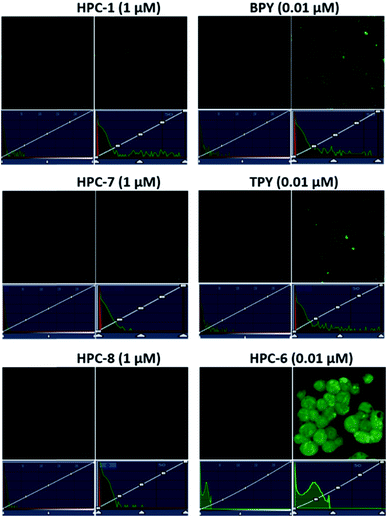 |
| Fig. 3 Revealing the false negativity in the background of cell imaging hidden under the normal intensity scale of a fluorescence microscope. Hek293t cells pre-treated with TPEN (50 μM) were incubated with HPC-n (n: 1, 6, 7, 8), BPY and TPY for 20 min at 37 °C. | |
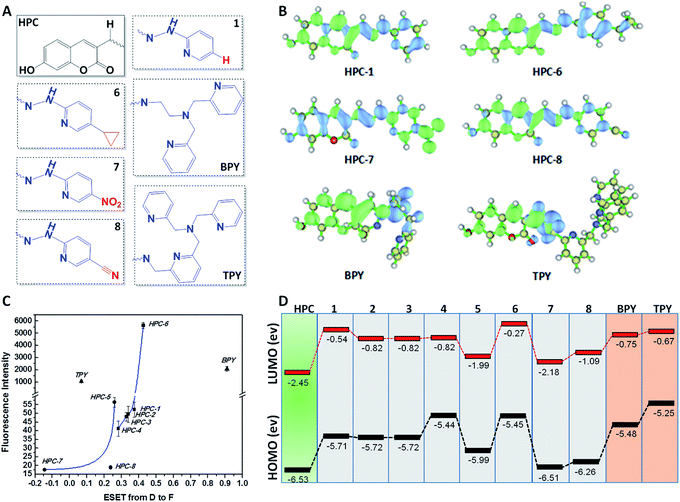 |
| Fig. 4 (A) The structures of HPC-n (n: 1, 6, 7, 8), HPC, BPY and TPY. (B) Theoretical calculations of excited state electron transfer (green and blue represent the high and low electron densities, respectively). (C) ESET in the HPC-n, HPC, BPY and TPY structures. (D) The HOMO and LUMO orbital energies of HPC-n, HPC, BPY and TPY. | |
Ultralow FNB and high fidelity imaging in cells and zebrafish
Before analysis, we investigated the practicability of HPC-7 and HPC-8, because although the two have a very low background, their responses to Zn ions may be different, which will alter their practical behaviors. At three levels (0.01, 0.1 and 1 μM), we tested their fluorescent responses toward Zn. As Fig. 7D shows, although HPC-7 has a lower background, its responses are 9–52 fold, while HPC-8 has a higher background, but better responses at 27–130 folds. We then performed imaging experiments using both of the two probes. All of the fluorescence intensity scales were set as the compressed region, as in Fig. 3. First, we used HPC-7 to image the SHSY-5Y cells, as this probe had a lower fluorescence background while the SHSY-5Y cell contained more Zn.36 As shown in Fig. 5A-1, the spontaneous zinc ions were visualized clearly using this probe. In parallel, the zinc chelator TPEN ((2-pyridylmethyl)ethylenediamine) was used to pre-treat cells before incubation with HPC-7. As shown in Fig. 5A-2, there was no background interference even under the very narrow intensity scale of the microscope, which was obviously attributed to the ultra-low background of HPC-7. Moreover, comparison of these two groups demonstrated that the fluorescence intensity in group 1 was triggered by the spontaneous zinc in the cells. To further confirm this result, we supplemented Zn ions to cells parallel to group 2. As shown in Fig. 5A-3, the fluorescence intensity increased significantly. With the increasing addition of Zn, an enhanced fluorescence intensity was observed (Fig. 5A-4), which confirmed that all of the fluorescence intensity in group 1 was triggered by the Zn ions without any interference from the fluorescence background. Furthermore, under the compressed intensity scale of microscopy, the heterogeneous distribution of Zn ions can be observed (Fig. 5A-3), which is an additional benefit of the high fidelity imaging. Compared with the SHSY-5Y cells, the Hek293t cells contain less Zn ions.37 Thus, we used HPC-8 to image them as this probe possessed a higher sensitivity and stronger reporting intensity. It was found that although this probe responded, its intensity was not high owing to the low level of zinc in the Hek293t cells (Fig. 5B-1). Then, we used HPC-7 as a control (Fig. 5B-2) and found that under the same intensity scale, the cells do not show observable imaging, indicating that HPC-8 is more competent for imaging a reduced amount of analyte. Thus, we used HPC-8 to image the zinc released by the apoptotic Hek293t cells. As shown in Fig. 5B-3, the H2O2-induced apoptotic cells showed an obviously enhanced fluorescence. To verify this result, TPEN was used. As shown in Fig. 5B-4, despite being apoptotic, caused by H2O2, the TPEN-treated cells showed a significantly weakened fluorescence intensity. Applications in different cells indicated the necessity to optimize the probe for high fidelity imaging analysis, which can be provided by the cross-talk modulation of electron transfer.
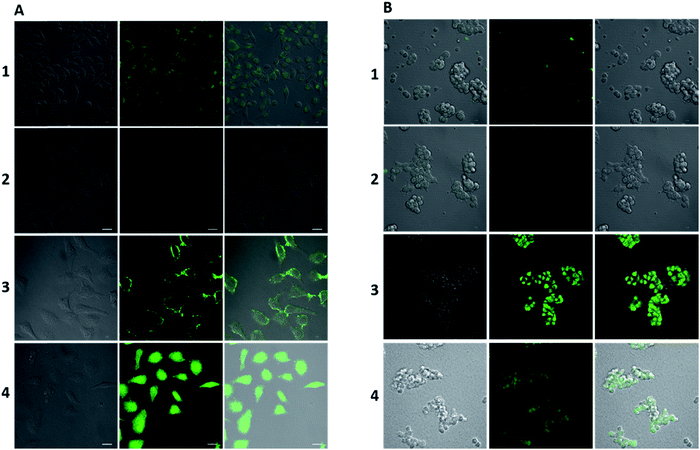 |
| Fig. 5 Ultra-sensitive imaging of zinc in cells. (A) (1) SHSY-5Y cells treated with HPC-7 (1 μM) for 20 min. (2) SHSY-5Y cells pretreated with TPEN (50 μM) before incubation with HPC-7 (1 μM) for 20 min. (3) SHSY-5Y cells pretreated with Zn ions (10 μM) and a group of cells parallel to (2) for 20 min. (4) SHSY-5Y cells pretreated with Zn ions (50 μM) and a group of cells parallel to (3) for 20 min. (B) (1) Hek293t cells were treated with HPC-8 (1 μM) for 20 min. (2) Hek293t cells were treated with HPC-7 (1 μM) for 20 min. (3) Hek293t cells were pretreated with H2O2 (200 μM) for 5 h, then treated with HPC-8 (1 μM). (4) Hek293t cells were pretreated with H2O2 (200 μM) for 5 h, then treated with TPEN (50 μM) for 0.5 h, and then treated with HPC-8 (1 μM) for 0.5 h. Images are displayed under the compressed intensity scale of microscopy as in Fig. 3. Emission window: (left) bright field; (middle) green channel (430–650 nm); and (right) merged. Scale bar: 20 μm. | |
Next, we performed imaging analysis in zebrafish. Before the analysis, we investigated the fluorescence background of probes in zebrafish. We used 1 μM of HPC-8 to incubate the TPEN-pretreated zebrafish. As shown in Fig. 6A, HPC-8 in zebrafish exhibits a rather low fluorescence under the normal scale of intensity. Even if we compressed the scale to 20%, we did not find any fluorescence interference (Fig. 6B). Actually, from the fluorescence distribution, we can see that the strongest distribution is no more than 5% of the fluorescence scale, demonstrating the satisfactorily low background. Therefore, HPC-8 was proven to be very suitable for high fidelity imaging of zebrafish. Owing to this property, we performed the following imaging analysis. Firstly, two groups of zebrafish were cultured with HPC-8, respectively, before and after TPEN treatment. Compared with the Fig. 6C-1 group, Fig. 6C-2 shows a strong fluorescence that reflects the spontaneous zinc ions in zebrafish. Next, we compressed the intensity scale to the same extent as that of Fig. 6B. As a result, Fig. 6C-3 showed no FNB and Fig. 6C-4 provided bright fluorescence images. Thus, HPC-8 can provide highly accurate and sensitive imaging in zebrafish with negligible FNB. The above described studies demonstrate that an ultra-low background probe can be achieved by enhancing the ESET based on synergistic PET. Next, we applied this cross-talk strategy to image higher animals, such as mice. Thus, we investigated control of the reporting intensity using this strategy.
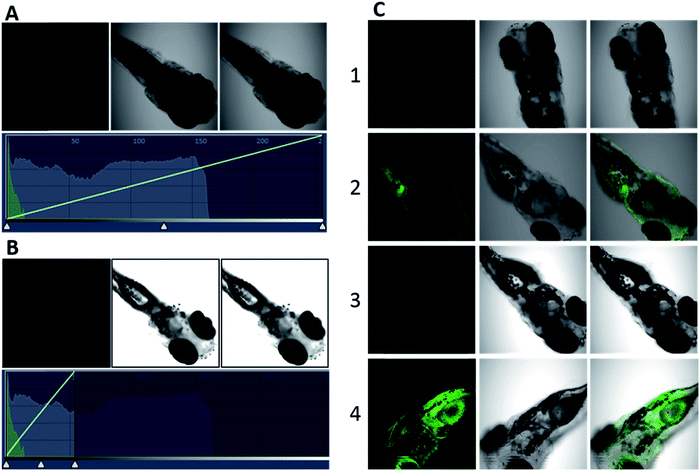 |
| Fig. 6 Ultra-sensitive imaging of zinc in zebrafish. Zebrafish pretreated with TPEN (50 μM) for 60 min, then treated with HPC-8 (1 μM) for 30 min: (A) under a normal scale of intensity; (B) under a compressed scale of intensity as indicated by the white triangle on the bottom. Zebrafish pretreated with TPEN (50 μM) for 60 min then treated with HPC-8 (1 μM) for 30 min (C1)/(C3); zebrafish pretreated with HPC-8 (1 μM) for 30 min (C2)/(C4): (C1) and (C2) under a normal scale of intensity; and (C3) and (C4) under a compressed scale of intensity, as in (B). Emission window: (left) green channel (430–650 nm); (middle) bright field; and (right) merged. | |
Cross-talk modulation of ESET and PET for in vivo imaging
According to the regulator, we divided the probes into three groups. First, we can see that both Zn–BPY and Zn–TPY show a weak ESET from the donor to HPC (Fig. 7C). Correspondingly, the fluorescence intensities of these are not strong. Moreover, the fluorescent enhancement was only 2.1–3.5 fold (Fig. 7D) with a strong FNB. Thus, BPY and TPY were far from meeting the requirements for imaging. The second group includes HPC-1, HPC-2, HPC-3, HPC-4 and HPC-6, and shows significant ESET from hpy to HPC in their Zn-coordinated products. As seen from Fig. 7C, with increasing ESET, the reporting signals increased. Clearly, HPC obtained more electrons at the excited state, which increased the probability of a transition, thereby enhancing the signals. The third group included HPC-7, HPC-8 and HPC-5, which led to increased ESET from the donor hpy to the fluorophore HPC (Fig. 7C) with their electron-withdrawing moiety. Accordingly, these compounds exhibited a gradually increased fluorescence intensity. The above described investigations demonstrate that the reporting signal can be obviously regulated by ESET although these compounds all comply with the PET-inhibited turn on of fluorescence. Thus, we can achieve a probe with a strong reporting intensity by decreasing the ESET from F to D and also forbidding the PET. However, in practice, it is still necessary to consider the question of whether to choose a probe with a higher reporting intensity or a greater florescence enhancement. From Fig. 7B and D, it can be seen that HPC-5 responds to Zn using the strongest fluorescence intensity, but its background fluorescence is not the lowest, and also its fluorescence enhancement is not the largest (70 fold). While, HPC-1 displayed a lower background and quite a good enhancement of 140 folds, its reporting intensity is not the largest. The same is true for HPC-8. Thus, for the in vivo imaging, we performed a thorough investigation of the advantages of the fluorescence background (HPC-8) and reporting intensity (HPC-5), as well as the responding fold increase (HPC-1). Prior to the in vivo imaging, we investigated these probes in the liver tissues of mice. To ensure the consistency, a saline solution of the probe (HPC-8; HPC-5; HPC-1) was injected into the heart of mice over 10 min at the rate of 2 drops per second, then the liver was dissected for imaging. As Fig. 8 shows, HPC-5 and HPC-1 exhibit a stronger fluorescence than HPC-8, suggesting that the two are more beneficial to tissue penetration. This result can be confirmed by the fluorescence intensity distribution on the right. Fig. 8d demonstrates that HPC-5 and HPC-1 provide the reporting signals that mainly occupy the high intensity area of the fluorescence scale, while HPC-8 mostly provided the reporting signals in the low-intensity area. Thus, for tissue penetration, a probe that responds with a sufficiently strong intensity should be what we need, and this provides important guidance for the following in vivo imaging.
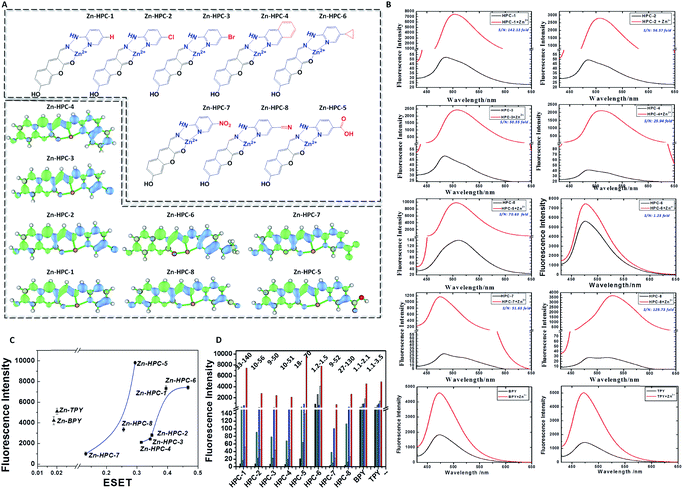 |
| Fig. 7 (A) Structures and ESET (green and blue represent high and low electron densities, respectively) of Zn–HPC-n (n: 1–8) in the excited state. (B) Fluorescence intensity changes of HPC-n (n: 1, 6, 7, 8; 1 μM), BPY (1 μM) and TPY (1 μM) in response to Zn ions (20 μM). (C) ESET in Zn–HPC-n (n: 1–8). (D) Fluorescence response of HPC-n (0.01; 0.1; and 1 μM), BPY (0.01; 0.1; and 1 μM) and TPY (0.01; 0.1; and 1 μM) to Zn ions (20 N). Experiments were performed in HEPES buffer solution (50 mM HEPES, 100 mM KNO3, pH = 7.2). | |
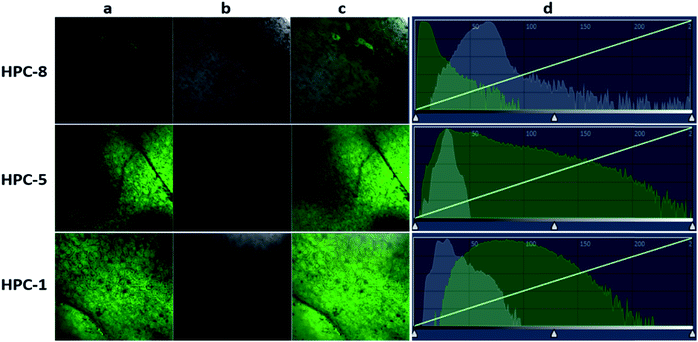 |
| Fig. 8 Cardiac perfusion imaging of probes in the livers of mice. 50 mL (20 μM) samples of HPC-8, HPC-5 and HPC-1 solutions were respectively injected into the superior vena cava of mice over 10 min at a rate of 2 drops per second, and the livers were dissected for imaging analysis: (a) emission channel (430–650 nm); (b) bright field; (c) merged. | |
Maximizing the reporting intensity for further reduction of the FNB
Unlike cell or tissue imaging, in vivo imaging is more challenging owing to the thick fur and self-fluorescence. Even for near-infrared probes, a high dosage is needed to provide a reporting signal strong enough for penetrating the fur. To overcome this difficulty, we can use this modulation strategy to achieve a probe with a strong intensity, so as to reduce the FNB to the lowest level. Therefore, we investigated the probes with both a high reporting intensity and a high sensitivity for their practicability in in vivo imaging. These probes include HPC-1, HPC-2, HPC-3, HPC-4, HPC-5, HPC-7 and HPC-8, which can offer more than a 50 fold increase in the fluorescent response. As shown in Fig. 9A-a, HPC-1, HPC-5 and HPC-8 exhibit an insignificant FNB. Then, in order to evaluate their reporting intensity, we injected Zn–HPC-1, Zn–HPC-5 and Zn–HPC-8 into mice. From Fig. 9A-b, it can be observed that Zn–HPC-8 provided a weak intensity. We list the fluorescence intensity in Fig. 9B, from which we can see that HPC-5-b provided the strongest fluorescence. This result indicates that the fluorescent response of HPC-5 is most likely to be detected during the in vivo imaging. Moreover, on the premise of sufficient intensity, we can reduce the dosage of HPC-5 to further reduce the FNB. Although HPC-1 and HPC-8 have a lower background, neither of them can provide a stronger reporting intensity than HPC-5. It is no doubt that the reporting signal can be displayed by reducing the fluorescence scale of the microscope, but the self-fluorescent background of fur will be passively enhanced. For example, owing to their weak reporting fluorescence intensities, if probes HPC-2, HPC-3, HPC-4 and HPC-7 were to exhibit a detectable reporting signal, without increasing their dosage, the fluorescence scale would have to be decreased. As a result, the fluorescent backgrounds of the probes themselves would be forced to rise, as shown in Fig. 9C. Consequently, for in vivo imaging, probe HPC-5, which has a high reporting intensity should be competent.
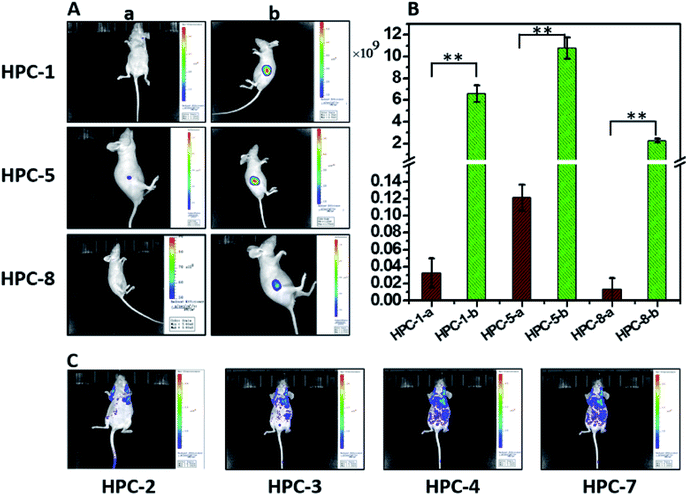 |
| Fig. 9 (A) In vivo fluorescence imaging of (a) HPC-n and (b) Zn–HPC-n (n: 1, 5, 8) in mice. (B) Fluorescence intensity collected from (A-a) and (A-b) with statistical analysis performed using Student's t-test (n = 10), **P < 0.01. (C) In vivo fluorescence imaging of HPC-2, HPC-3, HPC-4 and HPC-7 in mice. HPC-n and Zn–HPC-n (100 μL, 20 μM). | |
In vivo monitoring of zinc during tumor development
On the basis of the above described investigations, we set out to perform imaging analysis of zinc in tumors to give the first in vivo fluorescence imaging of zinc. The role of zinc in tumors has always been subtle. It is reported that the proliferative effect of the growth factor in tumors is accompanied by a zinc increase, and in the case of zinc deprivation, cell proliferation is reduced.38 Cancerous cells may accumulate more zinc than juxtaposed non-cancerous cells, implying a potential tissue specificity for zinc distribution. However, fluorescence visualization of this characteristic during tumor development of hepatocellular carcinoma is still challenging. Therefore, we hoped to achieve this with the aid of the probe featuring an ultra-low FNB and a strong reporting intensity. First, we investigated the accumulation of zinc at different levels during the development of hepatocellular carcinoma in mice. Mice were subcutaneously inoculated with HepG2 cells, and their growth and tumorigenesis were observed daily. As shown in Fig. 10A, the fluorescence intensity in the blank group a was found to be the lowest. The maximum fluorescence intensity was gradually enhanced from group b to h, which indicated the increased peak value of the Zn accumulated in the tumors. The lowest value for the fluorescence intensity did not exhibit a significant increase, which can be attributed to the metabolic and regulatory effects. This experiment demonstrates that the probe HPC-5 is sensitive to the rising zinc in vivo and can thus be used to monitor zinc-mediated tumor development. Based on this, we then tracked the time-dependent development of tumor-bearing mice. We divided the mice into two groups, respectively, for the control and test. 100 μL of ZnCl2 solution (100 μM) was injected into mice to achieve stable statistical data. In vivo imaging was performed during 1–4 weeks of tumor development. From Fig. 10B and F, we observed a significantly strong fluorescence intensity distribution in groups m to p, compared with the control groups i to l. Moreover, during the development of the tumor, the fluorescence intensity showed a time-dependent upward trend, indicating the accumulation of zinc in the tissues of the tumor. For confirmation, we analyzed the anatomical tumors. As shown in Fig. 10G, the weight of the tumors from groups a to h exhibited an upward trend, which was due to the fact that the accumulated zinc led to vigorous cell mitosis and promoted the up-regulation of growth factors. Obviously, the fluorescence intensity of anatomical tumors was positively correlated with the Zn-accumulated development of the hepatocellular carcinoma. Therefore, under the mode of high fidelity, the in vivo imaging of Zn accumulation during tumor development was successfully completed.
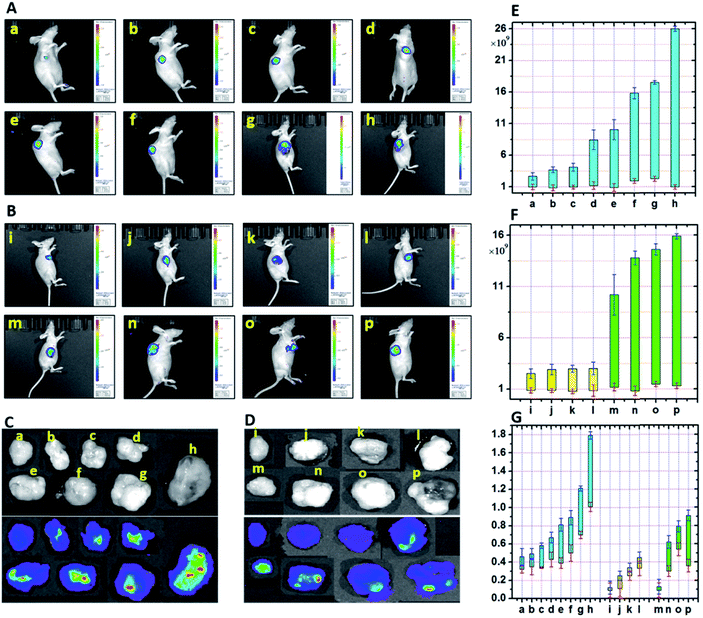 |
| Fig. 10
In vivo fluorescence imaging of zinc in mice tumors. (A) 100 μL of zinc chloride solution (a to h: 0; 10; 30; 60; 100; 150; 200; and 300 μM) was injected into each group for 4 weeks. (B) 100 μL of zinc chloride solution (100 μM) was injected into each group for 4 weeks when tumors were visible to the naked eye (m: 1 week; n: 2 weeks; o: 3 weeks; p: 4 weeks); the control group (i: 1 week; j: 2 weeks; k: 3 weeks; l: 4 weeks) was injected with physiological saline. (C) Fluorescence imaging of the anatomical tumors from group (A). (D) Fluorescence imaging of the anatomical tumors from group (B). (E) and (F) Fluorescence intensity distributions collected from groups (A) and (B). (G) Weights of the anatomical tumors. HPC-5 probe (100 μL; 20 μM) was injected before in vivo imaging was performed 20 min later. Data are expressed as the mean ± S.D. (n = 10). | |
Conclusions
We have constructed an F–D–R system for the cross-talk modulation of ESET and PET, and thereby de novo designed a series of fluorescent probes for high fidelity imaging. These allow: (1) the adjustment of R to enhance ESET from F to D on the basis of synergistic PET to minimize the inherent FNB; and (2) the inhibition of PET and the decrease of ESET from F to D to enhance the reporting fluorescence, thereby further minimizing the FNB. This strategy allows researchers to design practical probes with minimal backgrounds and maximal reporting signals for high fidelity imaging without false negative responses. Benefiting from this strategy, a negligible FNB was achieved in the imaging analysis of cells/zebrafish/tissues, even under the rather low intensity scale of microscopy that can uncover the FNB. For the first time, the in vivo imaging analysis of zinc accumulation in malignant tumors was performed. This strategy and the implementation of this de novo design will provide the methodology to find practical molecular probes for in vivo imaging.
Conflicts of interest
There are no conflicts to declare.
Acknowledgements
All experiments were performed in compliance with the Chinese National Standard Laboratory Animal Guidelines for ethical review of animal welfare (GB/T 35892-2018) and all experiments followed institutional guidelines. All protocols were approved by the Institutional Animal Care and Use Committee in Binzhou Medical University, Yantai, China. Approval number: BZ2014-102R. This work was supported by the National Natural Science Foundation of China (No. 21535004, 91753111, 21390411, 21475074, and 21403123), the China Postdoctoral Science Foundation (2017M622254; 2016M600531), the Open Funds of the Shandong Province Key Laboratory of Detection Technology for Tumor Markers (KLDTTM2015-6; KLDTTM2015-9), and the Natural Science Foundation of Shandong Province (ZR201709240033; ZR2019MB016).
Notes and references
- J. Ning, T. Liu, P. Dong, W. Wang, G. Ge, B. Wang, Z. Yu, L. Shi, X. Tian, X. Huo, L. Feng, C. Wang, C. Sun, J. Cui, T. D. James and X. Ma, J. Am. Chem. Soc., 2019, 141, 1126–1134 CrossRef CAS PubMed.
- H. Wang, J. Chang, M. Shi, W. Pan, N. Li and B. Tang, Angew. Chem., 2019, 131, 1069–1073 CrossRef.
- W. Gao, Y. Sun, M. Cai, Y. Zhao, W. Cao, Z. Liu, G. Cui and B. Tang, Nat. Commun., 2018, 9, 231 CrossRef PubMed.
- Y. L. Pak, S. J. Park, D. Wu, B. Cheon, H. M. Kim, J. Bouffard and J. Yoon, Angew. Chem., Int. Ed., 2018, 57, 1567–1571 CrossRef CAS PubMed.
- J. Zhang, X. Chai, X.-P. He, H.-J. Kim, J. Yoon and H. Tian, Chem. Soc. Rev., 2019, 48, 683–722 RSC.
- D. Cheng, J. Peng, Y. Lv, D. Su, D. Liu, M. Chen, L. Yuan and X. Zhang, J. Am. Chem. Soc., 2019, 141, 6352–6361 CrossRef CAS PubMed.
- N. Sun, D. Malide, J. Liu, I. I. Rovira, C. A. Combs and T. Finkel, Nat. Protoc., 2017, 12, 1576–1587 CrossRef CAS PubMed.
- Z. Ye, H. Yu, W. Yang, Y. Zheng, N. Li, H. Bian, Z. Wang, Q. Liu, Y. Song, M. Zhang and Y. Xiao, J. Am. Chem. Soc., 2019, 141, 6527–6536 CrossRef CAS PubMed.
- J. Shin, P. Verwilst, H. Choi, S. Kang, J. Han, N. H. Kim, J. G. Choi, M. S. Oh, J. S. Hwang, D. Kim, I. Mook-Jung and J. S. Kim, Angew. Chem., 2019, 131, 5704–5708 CrossRef.
- X. Wu, W. Shi, X. Li and H. Ma, Acc. Chem. Res., 2019, 52, 1892–1904 CrossRef CAS PubMed.
- P. Mouroulis and R. O. Green, Opt. Eng., 2018, 57, 1–19 Search PubMed.
- J. Zhang, Q. Wang, Z. Guo, S. Zhang, C. Yan, H. Tian and W.-H. Zhu, Adv. Funct. Mater., 2019, 29, 1808153 CrossRef.
- J. Ning, W. Wang, G. Ge, P. Chu, F. Long, Y. Yang, Y. Peng, L. Feng, X. Ma and T. D. James, Angew. Chem., Int. Ed., 2019, 58, 9959–9963 CrossRef CAS PubMed.
- W. Fu, C. Yan, Z. Guo, J. Zhang, H. Zhang, H. Tian and W.-H. Zhu, J. Am. Chem. Soc., 2019, 141, 3171–3177 CrossRef CAS PubMed.
- Y. Yang, S. K. Seidlits, M. M. Adams, V. M. Lynch, C. E. Schmidt, E. V. Anslyn and J. B. Shear, J. Am. Chem. Soc., 2010, 132, 13114–13116 CrossRef CAS PubMed.
- H. Kobayashi, M. Ogawa, R. Alford, P. L. Choyke and Y. Urano, Chem. Rev., 2010, 110, 2620–2640 CrossRef CAS PubMed.
- H. S. Choi, S. L. Gibbs, J. H. Lee, S. H. Kim, Y. Ashitate, F. Liu, H. Hyun, G. Park, Y. Xie, S. Bae, M. Henary and J. V. Frangioni, Nat. Biotechnol., 2013, 31, 148–153 CrossRef CAS PubMed.
- L. Lauinger, J. Li, A. Shostak, I. A. Cemel, N. Ha, Y. Zhang, P. E. Merkl, S. Obermeyer, N. Stankovic-Valentin, T. Schafmeier, W. J. Wever, A. A. Bowers, K. P. Carter, A. E. Palmer, H. Tschochner, F. Melchior, R. J. Deshaies, M. Brunner and A. Diernfellner, Nat. Chem. Biol., 2017, 13, 709–714 CrossRef CAS PubMed.
- E. B. Wright and T. L. Dormandy, Nature, 1972, 237, 166 CrossRef CAS PubMed.
- N. W. H. Addink, Nature, 1950, 166, 693 CrossRef CAS PubMed.
- P. A. Futreal, L. Coin, M. Marshall, T. Down, T. Hubbard, R. Wooster, N. Rahman and M. R. Stratton, Nat. Rev. Cancer, 2004, 4, 177–183 CrossRef CAS PubMed.
- K. Komatsu, K. Kikuchi, H. Kojima, Y. Urano and T. Nagano, J. Am. Chem. Soc., 2005, 127, 10197–10204 CrossRef CAS PubMed.
- M. Royzen, A. Durandin, V. G. Young, N. E. Geacintov and J. W. Canary, J. Am. Chem. Soc., 2006, 128, 3854–3855 CrossRef CAS PubMed.
- K. Kiyose, H. Kojima, Y. Urano and T. Nagano, J. Am. Chem. Soc., 2006, 128, 6548–6549 CrossRef CAS PubMed.
- F. Qian, C. Zhang, Y. Zhang, W. He, X. Gao, P. Hu and Z. Guo, J. Am. Chem. Soc., 2009, 131, 1460–1468 CrossRef CAS PubMed.
- G. Masanta, C. S. Lim, H. J. Kim, J. H. Han, H. M. Kim and B. R. Cho, J. Am. Chem. Soc., 2011, 133, 5698–5700 CrossRef CAS PubMed.
- Z. Guo, G.-H. Kim, J. Yoon and I. Shin, Nat. Protoc., 2014, 9, 1245–1254 CrossRef CAS PubMed.
- D. Li, S. Chen, E. A. Bellomo, A. I. Tarasov, C. Kaut, G. A. Rutter and W.-h. Li, Proc. Natl. Acad. Sci. U. S. A., 2011, 108, 21063–21068 CrossRef CAS PubMed.
- Z. Liu, C. Zhang, Y. Chen, W. He and Z. Guo, Chem. Commun., 2012, 48, 8365–8367 RSC.
- C. Zhang, Z. Liu, Y. Li, W. He, X. Gao and Z. Guo, Chem. Commun., 2013, 49, 11430–11432 RSC.
- P. Du and S. J. Lippard, Inorg. Chem., 2010, 49, 10753–10755 CrossRef CAS PubMed.
- J. E. Kwon, S. Lee, Y. You, K.-H. Baek, K. Ohkubo, J. Cho, S. Fukuzumi, I. Shin, S. Y. Park and W. Nam, Inorg. Chem., 2012, 51, 8760–8774 CrossRef CAS PubMed.
- B. Tang, H. Huang, K. Xu, L. Tong, G. Yang, X. Liu and L. An, Chem. Commun., 2006, 3609–3611, 10.1039/b606809j.
- Z. Xu, J. Yoon and D. R. Spring, Chem. Soc. Rev., 2010, 39, 1996–2006 RSC.
- Y. Chen, Y. Bai, Z. Han, W. He and Z. Guo, Chem. Soc. Rev., 2015, 44, 4517–4546 RSC.
- W. Li, B. Fang, M. Jin and Y. Tian, Anal. Chem., 2017, 89, 2553–2560 CrossRef CAS PubMed.
- A. M. Hessels, P. Chabosseau, M. H. Bakker, W. Engelen, G. A. Rutter, K. M. Taylor and M. Merkx, ACS Chem. Biol., 2015, 10, 2126–2134 CrossRef CAS PubMed.
- Y. Cui, S. Vogt, N. Olson, A. G. Glass and T. E. Rohan, Cancer Epidemiol., Biomarkers Prev., 2007, 16, 1682–1685 CrossRef CAS PubMed.
Footnote |
† Electronic supplementary information (ESI) available. See DOI: 10.1039/c9sc05765j |
|
This journal is © The Royal Society of Chemistry 2020 |
Click here to see how this site uses Cookies. View our privacy policy here.