DOI:
10.1039/D0RA09333E
(Paper)
RSC Adv., 2020,
10, 43400-43411
Achieving polydispersive HDPE by N,N,N-Co precatalysts appended with N-2,4-bis(di(4-methoxyphenyl)methyl)-6-methylphenyl†‡
Received
2nd November 2020
, Accepted 23rd November 2020
First published on 10th December 2020
Abstract
A family of unsymmetrical 2-(2,4-bis(di(4-methoxyphenyl)methyl)-6-MeC6H2N)-6-(1-(arylimino)ethyl)pyridine-cobalt dichloride complexes has been synthesized and characterized by NMR spectroscopy, FT-IR spectroscopy and elemental analysis as well as single crystal X-ray diffraction for Co2 and Co4. Activated with either MAO or MMAO, all the cobalt precatalysts displayed high activities toward ethylene polymerization and produced highly linear polyethylenes with high molecular weights as well as wide polydispersities; for example, the performance using Co1/MAO at 50 °C reached 9.17 × 106 g PE (mol of Co)−1 h−1 with the production polyethylene of molecular weight as high as Mw = 3.14 × 105 g mol−1, Tm = 134.3 °C besides its wide polydispersity of Mw/Mn of 54.6. Besides the terminal vinyl group of the resultant polyethylenes, it is rare for a late-transition metal catalyst to achieve highly linear polyethylenes with not only wide polydispersity but also high molecular weights, being similar to high-density polyethylenes produced using Phillips catalyst.
Introduction
The discovery of bis(imino)pyridine-iron and cobalt dichlorides (A, Scheme 1) as highly active precatalysts for ethylene polymerization heralded a new era in the organometallic application of late transition metals as well as polyolefin catalysts.1 Subsequently, many researchers from both academia and industry have developed various ligand units through extensive modification of 2,6-bis(imino)pyridine derivatives2 and newly designed N-heterocyclic compounds such as 2-benzimidazolyl-6-aryliminopyridines,3 N-[(pyridin-2-yl)methylene]-8-arylaminoquinolines,4 2,8-bis(arylimino)-quinolones,5 and 2-arylimino-1,10-phenanthrolines,6 which have appeared as compatible supports for cobalt and iron precatalysts.2,7 More importantly the incorporation of fused carbocyclic rings to the central pyridine (B, Scheme 1) has been systematically implemented leading to novel ligands containing either singly or doubly fused derivatives with ring sizes of between five and eight for introducing controlled amounts of strain to the parent bis(imino)pyridine framework with a view to modifying the donor properties of the tridentate ligands.8,9 With the particular regard to cobalt precatalysts, polyethylenes with different molecular weights were produced by those catalytic systems;9 for examples, precatalysts supported by the single five-membered fused9a or doubly six-membered fused systems9b produced from oligomers to polyethylene. Narrow dispersive polyethylenes were formed by precatalysts with the single six-,9c or seven-numbered fused systems9d as well as doubly seven-9e or eight-membered fused systems;9f in addition, polyethylenes with mono- to bimodal distributions were achieved through using different cocatalysts.9f
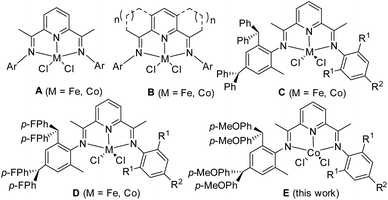 |
| Scheme 1 Structural variations in bis(imino)pyridine-cobalt and -iron precatalysts (A–E). | |
From industrious view, the investigation is worthily conducted not for the precatalysts themselves, but for the polyethylenes formed and their properties demanded. Though there is critical comment on the trace toxic chromium remained in the resultant polyethylenes by Phillips catalysts, the obtained polyethylenes provide better performances of “shear thinning” and “melt strength” because of their unique wide polydispersities (8–65).10 With this fact in mind, the bis(arylimino)pyridylcobalt model1 is reconsidered for its possibility for polydispersive polyethylenes; that is, the cobalt catalytic system is also worthily explored in producing polyethylenes with wide dispersity instead of narrow dispersive polyethylenes being pursued.2
The N-aryl groups diversification of bis(arylimino)pyridines has finely turned their cobalt complex precatalysts for varying catalytic performances and obtaining polyethylenes with different properties;2a,7b especially, the promising precatalysts of iron or cobalt complexes have been developed through using benzhydryl substituents11,12 either benzhydryl6,11,12a or its derivative di(4-fluorophenyl)methyl.12b Within these precatalysts, higher catalytic activities have been commonly achieved when using unsymmetric phenylamine derivatives such as C (Scheme 1) from 2,4-dibenzhydryl-6-methylphenylamine12c,d and D (Scheme 1) from 2,4-bis(di(4-fluorophenyl)-methyl)-6-methyl-phenylamine,12e and the latter exhibited good thermal stability. Subsequently the methoxy group was introduced into the benzhydryl group, producing the unsymmetric 2-(2,4-bis(di(4-methoxyphenyl)- methyl)-6-MeC6H2N)-6-(1-(arylimino)ethyl)pyridine cobalt(II)chlorides (E, Scheme 1), of which the catalytic performance was investigated towards ethylene polymerization. Interestingly, the resultant polyethylenes are significantly different to those obtained by previously cobalt precatalysts; the polydispersive, high molecular weight and linear polyethylenes are firstly achieved by cobalt complex precatalysts. These may result a potential of industrial application. Herein the synthesis and characterization of the title cobalt complexes were described as well as their catalytic performances under various polymerization conditions along with microstructures of resultant polymers.
Results and discussion
Synthesis and characterization of the cobalt(II) complexes Co1–Co5
The compound 2,4-bis(di(4-methoxyphenyl)methyl)-6-methylaniline was synthesized using the similar procedure reported previously,13 which reacts with 2,6-diacetylpyridine to prepare monoketone derivative, 2-acetyl-6-(1-(2,4-bis(di(4-methoxyphenyl)methyl)-6-methylphenylimino)ethyl)pyridine. Then refluxing the monoketone derivative and equivalent aniline with a catalytic amount of p-TsOH in toluene formed a series of 2-(1-(2,4-bis(di(4-methoxyphenyl)methyl)-6-methyl-phenylimino)ethyl)-6-(1-(arylimino)ethyl)pyridine ligands (L1–L5) in acceptable yields {aryl = 2,6-Me2C6H3 (L1), 2,6-Et2C6H3 (L2), 2,6-iPr2C6H3 (L3), 2,4,6-Me3C6H2 (L4) and 2,6-Et2-4-MeC6H2 (L5)} (Scheme 2). All the organic compounds were fully characterized by 1H and 13C NMR spectroscopy, elemental analysis, and the FT-IR spectra. The organic compounds reacted with cobalt dichlorides in dichloromethane and ethanol to form their corresponding cobalt complexes Co1–Co5 (Scheme 2) in good yields, brown solids. All new cobalt complexes have been characterized by elemental analysis as well as 1H NMR, and FT-IR spectroscopy. In the FT-IR spectra, the stretching vibrations for C
Nimine bonds in the complexes appear in the range of 1615–1627 cm−1 which are lower in wave number than those for the free ligands (1643–1650 cm−1), indicating the coordination of the ligand with the cobalt metal atom. Such shifts are consistent with structurally related cobaltous chloride complexes reported elsewhere.12,14b
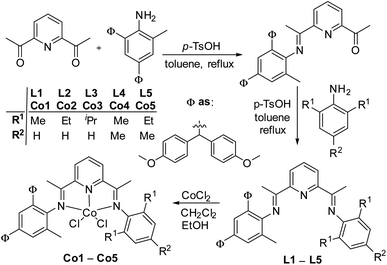 |
| Scheme 2 Synthetic procedure for the ligands L1–L5 and their cobalt complexes Co1–Co5. | |
Moreover, in the 1H NMR spectra of Co1–Co5, recorded in CDCl3 at ambient temperature, broad paramagnetically shifted peaks are a feature of all the complexes (Fig. 1 and S1–S4†). The assignment of the peaks has been made through a comparison with data recorded for related Co(II) (S = 3/2) complexes, relative integration and proximity to the paramagnetic center.1c,2b,14 Taking the complex Co1 for example, two downfield peaks at δ 39.82 (F) and δ 110.59/108.82 (E/E′) can be assigned to the para-pyridyl and meta-pyridyl protons, respectively, which is consistent with data reported elsewhere. On the other hand, the non-equivalent meta-aryl protons can be seen more upfield at δ 7.55 (H for Ar-Hm) and δ 18.23 (C for Ar-Hm) (Fig. 1). As previously mentioned,14b the more upfield peaks can be assigned to the methyl protons B (δ −24.87), and the CH(p-MeOC6H4)2 protons have been ascribed to the signal at δ −20.99.
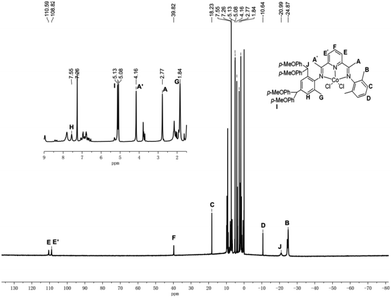 |
| Fig. 1 1H NMR spectrum of Co1; recorded in CDCl3 at ambient temperature. | |
To further confirm the structural identity of the complexes, Co2 and Co4 were used for representative examples as single crystal X-ray diffraction studies. Single crystals of Co2 and Co4 were grown by slow diffusion of hexane into a dichloromethane solution. Their molecular structures are shown in Fig. 2 and 3; selected bond lengths and angles are listed in Table 1. The structures of Co2 and Co4 are analogous to each other.
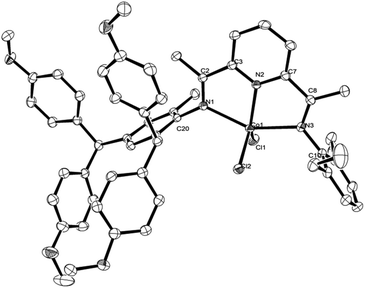 |
| Fig. 2 Molecular structure of complex Co2 with thermal ellipsoids at 30% probability. Hydrogen atoms have been omitted for clarity. | |
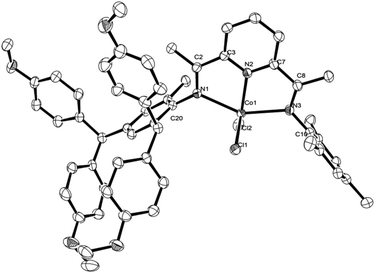 |
| Fig. 3 Molecular structure of complex Co4 with thermal ellipsoids at 30% probability. Hydrogen atoms have been omitted for clarity. | |
Table 1 Selected bond lengths (Å) and angles (°) for Co2 and Co4. Catalytic performance for ethylene polymerization
|
Co2 |
Co4 |
Bond lengths (Å) |
Co(1)–N(1) |
2.266(2) |
2.286(2) |
Co(1)–N(2) |
2.030(2) |
2.041(2) |
Co(1)–N(3) |
2.274(2) |
2.282(2) |
Co(1)–Cl(1) |
2.2529(8) |
2.2372(7) |
Co(1)–Cl(2) |
2.2484(8) |
2.2352(8) |
N(1)–C(2) |
1.281(3) |
1.280(4) |
N(1)–C(20) |
1.441(3) |
1.442(3) |
N(2)–C(3) |
1.341(3) |
1.342(3) |
N(2)–C(7) |
1.343(3) |
1.335(3) |
N(3)–C(8) |
1.277(4) |
1.284(4) |
N(3)–C(10) |
1.441(3) |
1.434(4) |
![[thin space (1/6-em)]](https://www.rsc.org/images/entities/char_2009.gif) |
Bond angles (°) |
N(1)–Co(1)–N(2) |
75.55(8) |
74.98(8) |
N(1)–Co(1)–N(3) |
150.47(8) |
149.94(8) |
N(1)–Co(1)–Cl(1) |
95.35(6) |
100.65(6) |
N(1)–Co(1)–Cl(2) |
100.78(6) |
93.68(6) |
N(2)–Co(1)–N(3) |
74.97(8) |
74.96(8) |
N(2)–Co(1)–Cl(1) |
124.09(7) |
117.13(7) |
N(2)–Co(1)–Cl(2) |
120.99(7) |
126.71(7) |
N(3)–Co(1)–Cl(1) |
102.44(7) |
93.03(6) |
N(3)–Co(1)–Cl(2) |
92.90(6) |
104.17(6) |
C(10)–N(3)–Co(1) |
124.78(17) |
125.96(17) |
C(20)–N(1)–Co(1) |
128.15(16) |
126.27(16) |
As shown in Fig. 2 and 3, in each case a single cobalt center is bound by two chloride ligands and three nitrogen donors, N1, N2 and N3, to afford a distorted square pyramidal geometry; structurally related five-coordinate species have been previously reported.12 The cobalt atom lies 0.046 Å above the basal plane for Co2 and 0.002 Å for Co4. The Co(1)–N(2) pyridine bond [2.030(2) Å, Co2, and 2.041(2) Å, Co4] is significantly shorter than the Co(1)–N(1) imine and Co(1)–N(3) imine bonds [2.266(2) and 2.274(2) Å, Co2, 2.286(2) and 2.282(2) Å, Co4], indicating stronger coordination of N-pyridine with a metal center. The N-aryl groups are nearly right angled with respect to the plane of the N^N^N chelate dihedral angle: 82.9° and 83.5° for Co2 and 85.8° and 79.2° for Co4, revealing the potential catalytic ability in ethylene polymerization.
To develop the potential of precatalysts Co1–Co5 to reconcile the polymerization of ethylene, methylaluminoxane (MAO) and modified methylaluminoxane (MMAO) were chosen as activator, both aluminoxanes have a reputation for being among the most potent in cobalt polymerization catalysis.12,15 The Al/Co molar ratio, reaction temperature, run time and ethylene pressure are all parameters to be investigated. The molecular weights (Mw) and molecular weight distributions (Mw/Mn) of the resultant polyethylenes were determined by gel permeation chromatography (GPC), while their melt temperatures (Tm) were determined by differential scanning calorimetry (DSC). In all cases gas chromatography (GC) was used to detect for any oligomeric fractions. In addition, the structural properties of selected samples of polyethylene were investigated using 1H/13C NMR spectroscopy.
Ethylene polymerization with the Co1/MAO
In the first instance Co1 was employed as the test precatalyst in combination with MAO as a means to ascertain the optimum set of operating conditions, the results are tabulated in Table 2. With the ethylene pressure at 10 atm and the Al/Co ratio at 2500, the temperature was altered from 30 °C to 60 °C (entries 1–4, Table 2), over a thirty-minute run time. A supreme value of the catalytic activity of 3.83 × 106 g PE (mol of Co)−1 h−1 was observed at 50 °C (entry 3, Table 2) affording polymers; no trace of short chain oligomers could be detected, which is outstanding to compare with the activity of the catalyst at other temperatures. As preliminary reported, cobalt catalysts tend to be quite sensitive towards reaction temperature.5,11a,d The polymers showed high molecular weight falling in the scope of 3.45 to 3.31 × 105 g mol−1, which are shown by the GPC curves in Fig. 4. The molecular weights of the polymers gradually decrease on increasing temperature, which can be accredited to a higher rate of chain transfer relative to chain propagation at elevated temperature.11
Table 2 Ethylene polymerization results using Co1/MAOa
Entry |
Precat. |
Al/Co |
T (°C) |
t (min) |
Yield (g) |
Activityb |
Mw |
Mw/Mnc |
Tmd (°C) |
Conditions: 1.5 μmol of Co1; 100 mL toluene, 10 atm ethylene. Values in units of 106 g PE (mol of Co)−1 h−1. Determined by GPC, and Mw: 105 g mol−1. Determined by DSC. 5 atm of ethylene. 1 atm of ethylene. |
1 |
Co1 |
2500 |
30 |
30 |
0.38 |
0.51 |
3.45 |
36.2 |
136.2 |
2 |
Co1 |
2500 |
40 |
30 |
0.94 |
1.25 |
3.40 |
62.0 |
136.1 |
3 |
Co1 |
2500 |
50 |
30 |
2.87 |
3.83 |
3.32 |
35.9 |
135.5 |
4 |
Co1 |
2500 |
60 |
30 |
0.84 |
1.12 |
3.31 |
57.2 |
135.2 |
5 |
Co1 |
3000 |
50 |
30 |
5.82 |
7.76 |
3.22 |
56.0 |
135.1 |
6 |
Co1 |
3250 |
50 |
30 |
6.88 |
9.17 |
3.14 |
54.6 |
134.3 |
7 |
Co1 |
3500 |
50 |
30 |
6.74 |
8.99 |
2.96 |
55.4 |
133.7 |
8 |
Co1 |
3750 |
50 |
30 |
6.43 |
8.57 |
2.89 |
52.3 |
133.8 |
9 |
Co1 |
4000 |
50 |
30 |
6.08 |
8.11 |
2.24 |
22.9 |
135.0 |
10 |
Co1 |
3250 |
50 |
5 |
4.71 |
37.68 |
2.87 |
43.2 |
133.3 |
11 |
Co1 |
3250 |
50 |
15 |
5.52 |
14.72 |
3.01 |
57.7 |
134.2 |
12 |
Co1 |
3250 |
50 |
45 |
7.46 |
6.63 |
3.36 |
56.3 |
134.5 |
13 |
Co1 |
3250 |
50 |
60 |
7.83 |
5.22 |
3.47 |
58.6 |
134.2 |
14e |
Co1 |
3250 |
50 |
30 |
3.19 |
4.25 |
2.75 |
53.5 |
134.6 |
15f |
Co1 |
3250 |
50 |
30 |
0.43 |
0.57 |
1.86 |
36.3 |
135.4 |
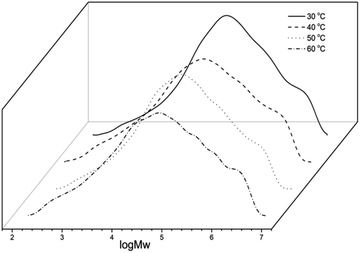 |
| Fig. 4 GPC curves of the polyethylenes (entries 1–4, Table 2). | |
Subsequently, the influence of Al/Co molar ratio was investigated with the reaction temperature fixed at 50 °C, On elevating the Al/Co molar ratio from 2500 to 4000 (entries 3 and 5–9, Table 2), the highest activity (9.17 × 106 g PE (mol of Co)−1 h−1) was revealed at an Al/Co molar ratio of 3250 (entry 6, Table 2). And the molecular weights of the polyethylene were high (range: 3.4 × 105 g mol−1 to 2.24 × 105 g mol−1) and decreased as the Al/Co molar ratio increased (Fig. 5). This latter observation can be credited to promoting chain transfer from cobalt to aluminum by increasing the amount of alkyl aluminum reagent.9e,16 Surprisingly, the dispersities for the resultant polyethylene are particular high, in the range 22.9–62.0, indicating multi-site behaviour for the active species. In previous literature,8f,15 resultant polyethylenes showed wide molecular weight distributions, however, some portion were quite low molecular weights. In addition, the quenched filtrates of current catalytic system were carefully examined by GC without observing any low-molecular polymers solved. Therefore the resultant polyethylenes by the title cobalt precatalysts could be expected to be alert polymers used with the properties of those by Phillips catalyst.10
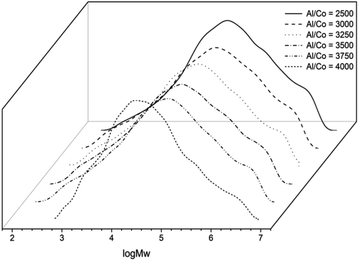 |
| Fig. 5 GPC curves of the polyethylenes (entries 3 and 5–9, Table 2). | |
To explore the lifetime of the active species derived from Co1/MAO, the polymerization runs were conducted over 5, 15, 30, 45, and 60 minutes (entries 6 and 10–13, Table 2) with the Al/Co ratio at 3250 and the temperature at 50 °C. The maximum activity of 37.68 × 106 g PE (mol of Co)−1 h−1 was observed after 5 min (entry 10, Table 2), which indicated the active species was rapidly generated after MAO addition and the catalytic process has barely induction period. Meanwhile, their activities gradually decreased along with prolonging reaction time from 5 to 60 min (Fig. 6), it is line with previous reports.1b,11d Furthermore, ethylene pressure (10 atm, 5 atm, 1 atm) was investigated, the activity dramatically dropped from 9.17 × 106 g PE (mol of Co)−1 h−1 to 0.57 × 106 g PE (mol of Co)−1 h−1 (entries 6, 14 and 15, Table 2) which can be accounted for, in part, to mass transport limitations of the monomer at this low pressure. Likewise, the molecular weights decreased with a reducing in ethylene pressure, in agreement with less facile insertion and lower solubility of the ethylene in the reaction solution at lower ethylene pressure.11d,18
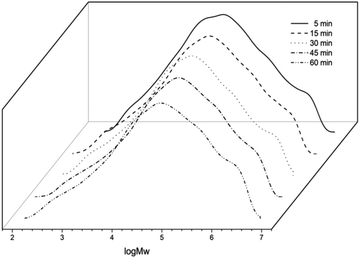 |
| Fig. 6 GPC curves of the polyethylenes (entries 6 and 10–13, Table 2). | |
Ethylene polymerization with the Co1–Co5/MAO
In order to investigate the influence of the ligand structure on the catalytic behavior, Co2–Co5 were additionally screened for ethylene polymerization under the optimum catalytic conditions (Al/Co ratio = 3250, 10 atm C2H4, 50 °C, 30 min) established independently for Co1/MAO. On activation with MAO, Co1–Co5 displayed good activities [5.43–9.32 × 106 g PE (mol of Co)−1 h−1], falling in the order Co4 [2,6-di(Me)] > Co1 [2,4,6-tri(Me)] > Co5 [2,6-di(Et)-4Me] > Co2 [2,6-di(Et)] > Co3 [2,6-di(i-Pr)] (entries 1–5, Table 3). As indicated in the Fig. 7, the activities of Co4 and Co5 with methyl group in the para position, were higher than the para-hydrogen complexes, Co1 and Co2, highlighting a positive effect of having an electron donating methyl group on the catalytic activity. Co3 owned the lowest activity because of the largest steric hindrance. With regard to the molecular weight, the polyethylenes display values ranging from 2.74 × 105 g mol−1 to 4.33 × 105 g mol−1 with Co5 [2,6-di(Et)-4-Me] exhibiting the highest value (entry 5, Table 3), which revealed that the particular steric properties in Co5/MAO are most suitable to promote chain growth leading to polyethylenes with relatively high molecular weights.3a,11a Furthermore, all polyethylenes obtained by Co1–Co5 exhibited wide molecular weight distribution (PDI = 31.9–54.6).
Table 3 Ethylene polymerization results using Co1–Co5/MAO under optimized conditionsa
Entry |
Precat. |
Yield (g) |
Activityb |
Mwc |
Mw/Mnc |
Tmd (°C) |
Conditions: 1.5 μmol of Co; 100 mL toluene, 10 atm ethylene. Values in units of 106 g PE (mol of Co)−1 h−1. Determined by GPC, and Mw: 105 g mol−1. Determined by DSC. |
1 |
Co1 |
6.88 |
9.17 |
3.14 |
54.6 |
134.3 |
2 |
Co2 |
6.51 |
8.68 |
3.92 |
41.0 |
135.4 |
3 |
Co3 |
4.07 |
5.43 |
4.00 |
31.9 |
136.7 |
4 |
Co4 |
6.99 |
9.32 |
2.74 |
46.5 |
134.7 |
5 |
Co5 |
6.75 |
9.00 |
4.33 |
47.5 |
135.5 |
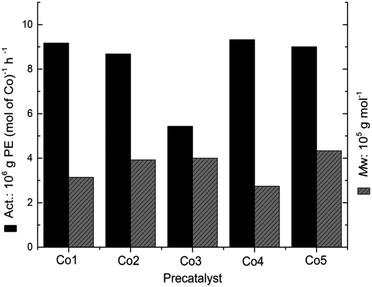 |
| Fig. 7 Comparative activity of Co1–Co5 and the Mw of the corresponding polymers (entries 1–5, Table 3). | |
Ethylene polymerization with the Co1/MMAO
With the ethylene pressure fixed at 10 atm, Co1/MMAO was again optimized by varying the Al/Co ratio, reaction temperature and reaction time, the results are collected in Table 4. In comparison with the Co1/MAO system, the Co1/MMAO system also revealed best activity at 50 °C (entries 1–6, Table 4) affording a polymeric product, no trace of short chain oligomers could be detected. With the increase of temperature, the activity decreases continuously, but up to 80 °C, the activity can still reach 2.79 × 106 g PE (mol of Co)−1 h−1, which proves the good thermal stability of the Co1/MMAO system. Then, the molecular weight of the polymer has a significant trend of decrease with the increase of temperature, which is consistent with previous reports.9,11 The GPC curves for them in Fig. S5.† Similarly, a wide molecular weight distribution of polyethylene (PDI = 20.7–28.4) was obtained, suggesting multi-active sites.11d
Table 4 Ethylene polymerization results using Co1/MMAOa
Entry |
Precat. |
Al/Co |
T (°C) |
t (min) |
Yield (g) |
Activityb |
Mwc |
Mw/Mnc |
Tmd (°C) |
Conditions: 1.5 μmol of Co1; 100 mL toluene, 10 atm ethylene. Values in units of 106 g PE (mol of Co)−1 h−1. Determined by GPC, and Mw: 105 g mol−1. Determined by DSC. 5 atm of ethylene. 1 atm of ethylene. |
1 |
Co1 |
2500 |
30 |
30 |
4.09 |
5.45 |
2.04 |
28.4 |
135.7 |
2 |
Co1 |
2500 |
40 |
30 |
4.32 |
5.76 |
1.54 |
23.9 |
135.9 |
3 |
Co1 |
2500 |
50 |
30 |
4.76 |
6.35 |
1.50 |
20.7 |
135.4 |
4 |
Co1 |
2500 |
60 |
30 |
4.28 |
5.71 |
1.04 |
25.8 |
133.6 |
5 |
Co1 |
2500 |
70 |
30 |
3.88 |
5.17 |
0.96 |
23.2 |
133.4 |
6 |
Co1 |
2500 |
80 |
30 |
2.09 |
2.79 |
0.93 |
26.6 |
130.1 |
7 |
Co1 |
2000 |
50 |
30 |
3.92 |
5.23 |
1.71 |
29.9 |
134.4 |
8 |
Co1 |
2750 |
50 |
30 |
5.25 |
7.00 |
1.43 |
26.0 |
134.3 |
9 |
Co1 |
3000 |
50 |
30 |
4.67 |
6.23 |
1.22 |
25.3 |
134.4 |
10 |
Co1 |
3250 |
50 |
30 |
4.18 |
5.57 |
1.08 |
25.5 |
133.9 |
11 |
Co1 |
3500 |
50 |
30 |
4.08 |
5.44 |
1.00 |
22.2 |
135.3 |
12 |
Co1 |
2750 |
50 |
5 |
1.76 |
14.08 |
1.12 |
24.5 |
134.7 |
13 |
Co1 |
2750 |
50 |
15 |
3.54 |
13.29 |
1.39 |
33.8 |
134.0 |
14 |
Co1 |
2750 |
50 |
45 |
5.49 |
4.88 |
1.58 |
31.6 |
134.4 |
15 |
Co1 |
2750 |
50 |
60 |
5.60 |
3.73 |
1.79 |
32.7 |
135.6 |
16e |
Co1 |
2750 |
50 |
30 |
2.80 |
3.73 |
0.99 |
21.1 |
133.3 |
17f |
Co1 |
2750 |
50 |
30 |
0.68 |
0.19 |
0.18 |
5.97 |
129.9 |
Further investigations were performed by varying the Al/Co ratio from 2000 to 3500 at a fixed temperature of 50 °C (entries 3 and 7–11, Table 4). Inspection of the polymerization data revealed that the highest activity (7.00 × 106 g PE (mol of Co)−1 h−1) was gained with an Al/Co ratio of 2750 (entry 8, Table 4). Below or above this Al/Co ratio there were negative effects on the activities.8,9 The molecular weights of polymers steadily decreased from 1.71 to 1.00 × 105 g mol−1 with the increase in Al/Co ratio (Fig. S6†).
The catalytic activity is inversely related to the polymerization time, and the highest value of 14.08 × 106 g PE (mol of Co)−1 h−1 was found at 5 min run time, indicating a short induction time required to generate the active sites (entry 12, Table 4). With the prolonged reaction time the activity gradually decreases (entries 8 and 13–15 Table 4) and the moderate value of 3.73 × 106 g PE (mol of Co)−1 h−1 was found even for 60 min (Table 4, entry 15), suggesting rather long lifetime of the active sites. The molecular weight of the obtained polyethylene increases constantly with reaction time. The product exhibits unimodal molecular weight distribution and the GPC curve of the reaction time is given in Fig. S7.† Similar dependencies have been observed for the related catalytic systems.12a,19 Furthermore, on reducing the pressure from 10 to 1 atm C2H4 the molecular weight of the polymer formed using Co1/MMAO significantly dropped (entries 8 and 17 Table 4), while at 5 atm C2H4 the molecular weight was as expected between that observed at the two limiting pressures (entries 8, 16 and 17, Table 4). Likewise the catalytic activity showed a similar downward trend as the ethylene pressure was decreased with value at 5 atm about half of that achieved at 10 atm.
Ethylene polymerization with the Co1–Co5/MMAO
Similarly, Co2–Co5/MMAO were screened using the optimum polymerization conditions (Al/Co ratio = 2750, 10 atm C2H4, 50 °C, 30 min) established independently for Co1/MMAO. In this case, Co1–Co5/MMAO displayed a narrower range in catalytic activities (3.59–7.00 × 106 g PE (mol of Co)−1 h−1) that falls in the order: Co1 > Co4 > Co2 > Co5 > Co3 (entries 1–5, Table 5, Fig. S8†). Different to that in MAO (Co4 as the highest), Co1 exhibited the highest activity in MMAO and then drops as the steric properties of the ortho-positions of the N-aryl group progressively increase; this finding can be attributed to the relative ease of ethylene coordination at the active site.9f,11d
Table 5 Ethylene polymerization using Co1–Co5/MMAO under optimized conditionsa
Entry |
Precat. |
Yield (g) |
Activityb |
Mwc |
Mw/Mnc |
Tmd (°C) |
Conditions: 1.5 μmol of Co; 100 mL toluene, 10 atm ethylene. Values in units of 106 g PE (mol of Co)−1 h−1. Determined by GPC, and Mw: 105 g mol−1. Determined by DSC. |
1 |
Co1 |
5.25 |
7.00 |
1.43 |
26.0 |
134.3 |
2 |
Co2 |
4.51 |
6.01 |
1.33 |
18.7 |
135.0 |
3 |
Co3 |
2.69 |
3.59 |
1.87 |
16.3 |
136.1 |
4 |
Co4 |
4.99 |
6.65 |
1.03 |
20.7 |
133.9 |
5 |
Co5 |
4.38 |
5.84 |
1.53 |
16.6 |
135.2 |
Interestingly, Co4 and Co5 with an additional methyl group exhibited a lower activity than that displayed with Co1 and Co2, which suggests a detrimental effect on catalyst performance by an electron donating group in this system, which is contrast to those in MAO.12a,b In terms of the molecular weight of the polymer, this was found to range from 1.03 to 1.87 × 106 g PE (mol of Co)−1 h−1 which is appreciably lower than with Co/MAO, the polymer formed using Co3 [2,6-di(i-Pr)] displayed the highest molecular weight 1.87 × 105 g mol−1, this showed that the steric hindrance within Co3 continuously enhanced chain-growth leading to polyethylenes with relatively high molecular weights.9e,14b
In most of the cases, the melting points of the resultant polyethylenes were higher than 130 °C, which suggests highly linear structures. To further investigate the microstructural properties of the polyethylenes, polyethylene obtained with Co1/MAO at 50 °C (entry 6, Table 2) and Co1/MMAO at 50 °C (entry 8, Table 4) were characterized by high-temperature 1H NMR and 13C NMR measurements at 100 °C in deuterated 1,1,2,2-tetra-chloroethane-d2. As shown in Fig. 8, polyethylene obtained with Co1/MAO at 50 °C display multiplet at δ = 5.91 ppm (a) and δ = 5.03 ppm (b) in the 1H NMR spectrum, together with the peaks around δ = 114.4 (a) and 139.6 ppm (b) in the 13C NMR spectrum, indicating that vinyl groups (–CH
CH2) were formed through the process of β-hydride elimination as a termination reaction. The high intensity of the singlet at δ = 1.36 ppm in the 1H NMR spectrum and the single peak of high intensity around δ = 30 ppm in the 13C NMR spectrum confirm the high linearity polyethylenes obtained. The carbon atoms of the methyl group at the saturated end of the macromolecule were observed at 14.26 ppm (e), along with the carbon atoms located at the close vicinity (c, d, and f) at 33.99, 32.23 and 22.92 ppm, respectively in Fig. 8.20c Meanwhile, as illustrated in the Fig. S9,† 1H NMR and 13C NMR spectra of polyethylene obtained with Co1/MMAO were similar to those obtained with Co1/MAO at 50 °C, indicating the vinyl groups (–CH
CH2) were also formed in the process of polymerization by Co1/MMAO at 50 °C.
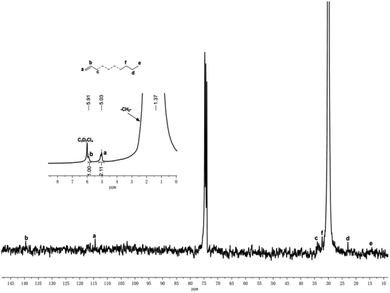 |
| Fig. 8 The 1H/13C NMR spectrum of the polyethylene produced using Co1/MAO (entry 6, Table 2). | |
To compare the catalytic performance of cobalt precatalysts with the methoxy in the benzhydryl group in this work with those with other groups (C and D in Scheme 1), the molecular weights and activities for the pre-catalysts C and D are displayed with current pre-catalyst E (Co1/MAO) together in Fig. 9.12c,e All three pre-catalysts like Co1/MAO [2,6-di(Me)] were screened under optimized conditions. Compared with C and D, the catalytic activity of E is slightly reduced, but the molecular weight of the polymer produced by E is much higher than that of C and D, so it can be seen that the introduction of methoxy group plays a positive role in the molecular weight of the polymer. The polyethylenes by C or D exhibits narrow molecular weight distribution range from 1.8 to 3.5,12 however, polymers by E show broad polydispersities from 22.9 to 62.0, confirming multiple active sites in catalytic system of E besides its continuous growth for the resultant polyethylenes with high molecular weights.
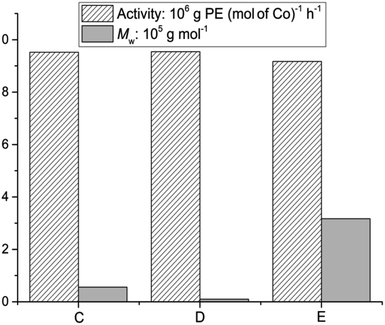 |
| Fig. 9 Comparison of the catalytic performance of Co1/MAO with previously reported comparators C and D (Scheme 1). | |
Experimental
General considerations
All operations including air and/or moisture sensitive compounds were performed under an atmosphere of nitrogen using standard Schlenk techniques. All solvents were dried over sodium and distilled under nitrogen atmosphere prior to use. Methylaluminoxane (MAO, 1.46 M in toluene) and modified methylaluminoxane (MMAO, 1.93 M in heptane) were purchased from Albemarle Corporation. Polymer grade of ethylene was purchased from Beijing Yanshan Petrochemical Company and used as received. Other reagents were purchased from Aldrich, Acros or local suppliers. The compound 2,4-bis(4,4′-dimethoxybenzhydryl)-6-methylaniline was prepared using the similar procedure reported previously.13 The 1H and 13C NMR spectroscopic measurements for the organic compounds were performed on a Bruker DMX 400 MHz and Bruker DMX 300 MHz instrument at room temperature. Chemical shifts are measured in ppm for the 1H and 13C NMR spectra and are relative to TMS as an internal standard. Elemental analyses were conducted on a Flash EA 1112 microanalyzer. FT-IR spectra were recorded using a PerkinElmer System 2000 FT-IR spectrometer. The molecular weights (Mw) and molecular weight distributions (Mw/Mn) of the polyethylenes were determined using a PL-GPC220 instrument at 150 °C with 1,2,4-trichlorobenzene as the solvent. The melt temperatures of the polyethylenes were measured from the second scanning run on a PerkinElmer TA-Q2000 DSC analyzer under a nitrogen atmosphere. In the procedure, a sample about 5 mg was heated to 160 °C at a heating rate of 20 °C min−1 and kept for 5 min at 160 °C to remove the thermal history and then cooled at same rate to −20 °C. For the 1H and 13C NMR spectra of the polyethylenes, a weighed amount of polyethylene (80–100 mg) was combined with 1,1,2,2-tetrachloroethane-d2 (2 mL) with TMS as an internal standard. Inverse gated 13C spectra recorded on a Bruker DMX 300 spectrometer at 75.47 MHz in 5 mm standard glass tubes at 100 °C with the number of scans between 3000 and 4000. Operating conditions used: spectral width 17.9856 kHz; acquisition time 1.8219 s; relaxation delay 2 s.
Synthesis and characterization
Synthesis of 2-acetyl-6-[1-(2,4-bis(di(4-methoxyphenyl)methyl)-6-methylphenylimino)ethyl]pyridine. 1,1′-(Pyridine-2,6-diyl)bis(ethan-1-one) (3.63 g, 22.0 mmol), 2,4-bis(di(4-methoxyphenyl)methyl)-6-methylaniline (11.2 g, 20.0 mmol) and p-TsOH (0.68 g, 4 mmol) in toluene was stirred and heated to 120 °C for 9 h. After removing most of solvent, the residue was purified by alumina column chromatography, eluting with petroleum ether/ethyl acetate (v/v = 20
:
1) to yield as a yellow solid. (6.25 g, 44.3%). 1H NMR (400 MHz, CDCl3, TMS): δ 8.46 (d, J = 8 Hz, 1H, Py-H), 8.10 (d, J = 8 Hz, 1H, Py-H), 7.91 (t, J = 8 Hz, 1H, Py-H), 6.97 (d, J = 8 Hz, 4H, Ph-H), 6.88–6.78 (m, 9H, Ph-H), 6.70 (d, J = 8 Hz, 2H, Ph-H), 6.64 (d, J = 8 Hz, 2H, Ph-H), 6.57 (s, 1H, Ph-H), 5.32 (s, 1H, CH), 5.26 (s, 1H, CH), 3.79 (s, 6H, 2 × OCH3), 3.76 (s, 3H, OCH3), 3.73 (s, 3H, OCH3), 2.72 (s, 3H, CH3), 1.93 (s, 3H, CH3), 1.63 (s, 3H, CH3). 13C NMR (100 MHz, CDCl3, TMS): δ 200.1 (C
O), 168.0 (C
N), 158.1, 157.8, 157.7, 155.6, 152.4, 146.2, 138.9, 137.3, 137.2, 137.1, 137.0, 136.0, 135.2, 135.0, 133.5, 130.5, 130.3, 130.2, 130.1, 129.2, 128.6, 124.7, 124.4, 122.4, 113.8, 113.6, 113.5, 113.3, 55.2, 54.7, 50.7, 25.6, 17.9, 16.4. FT-IR (cm−1): 3001(w), 2952(w), 2902(w), 2832(w), 1698 (νC
O, m), 1638 (νC
N, w), 1606(m), 1580(w), 1508(s), 1460(m), 1444(m), 1357(w), 1298(m), 1240(s), 1174(m), 1110 (w), 1033(m), 958(w), 896(w), 813(m), 777(m), 759(w), 733(w). Anal. calcd for C46H44N2O5 (704.87): C, 78.38; H, 6.29; N, 3.97. Found: C, 78.09; H, 6.27; N, 3.89%.
Synthesis of 2-[CMeN{2,4-{(4-MeOC6H4)2CH}2-6-Me}]-6-(CMeNAr)-C5H3N.
L1: Ar = 2,6-Me2C6H3. A suspension of 2-acetyl-6-[1-(2,4-bis(di(4-methoxyphenyl)-methyl)-6-methylphenylimino) ethyl)pyridine (1.06 g, 1.5 mmol), 2,6-dimethylaniline (0.22 g, 1.8 mmol) and p-TsOH (0.05 g, 0.3 mmol) in toluene was stirred and heated to 120 °C for 9 h. The resulting solution was concentrated on the rotary evaporator to give the crude reaction product, which was purified by alumina column chromatography, eluting with petroleum ether/ethyl acetate (v/v = 50
:
1) to yield as a yellow solid. (0.17 g, 14.4%). 1H NMR (400 MHz, CDCl3, TMS): δ 8.69 (d, J = 4 Hz, 1H, Py-H), 8.35 (d, J = 8 Hz, 1H, Py-H), 7.89 (t, J = 8 Hz, 1H, Py-H), 7.09 (t, J = 8 Hz, 2H, Ph-H), 6.98 (d, J = 8 Hz, 6H, Ph-H), 6.82 (m, 8H, Ph-H), 6.72 (d, J = 8 Hz, 2H, Ph-H), 6.66 (d, J = 8 Hz, 2H, Ph-H), 6.57 (s, 1H, Ph-H), 5.33 (s, 1H, CH), 5.31 (s, 1H, CH), 3.80 (s, 6H, 2 × OCH3), 3.77 (s, 3H, OCH3), 3.72 (s, 3H, OCH3), 2.18 (s, 3H, CH3), 2.09 (s, 3H, CH3), 2.04 (s, 3H, CH3), 1.95 (s, 3H, CH3), 1.65 (s, 3H, CH3). 13C NMR (100 MHz, CDCl3, TMS): δ 168.7 (C
N), 167.0 (C
N), 157.9, 157.8, 155.1, 146.4, 138.8, 137.2, 137.1, 136.8, 136.2, 135.4, 133.5, 130.6, 130.3, 129.2, 128.6, 128.0, 125.5, 124.9, 123.1, 122.2, 122.1, 113.6, 113.4, 55.3, 54.8, 50.7, 18.0, 16.7, 16.5. FT-IR (cm−1): 3003(w), 2950(w), 2899(w), 2833(w), 1645 (νC
N, m), 1608 (νC
N, w), 1578(m), 1508(s), 1463(m), 1449(m), 1365(w), 1299(m), 1245(s), 1209(w), 1174(m), 1120(w), 1080(w), 1036(m), 964(w), 826(w), 771(m), 750(m), 700(w). Anal. calcd for C54H53N3O4 (808.04): C, 80.27; H, 6.61; N, 5.20. Found: C, 80.02; H, 6.62; N, 5.16%.
L2: Ar = 2,6-Et2C6H3. Using a similar procedure as described for L1 but with 2,6-diethylaniline as the amine, L2 was isolated as a pale yellow powder (0.29 g, 22.7%). 1H NMR (400 MHz, CDCl3, TMS): δ 8.43 (d, J = 8 Hz, 1H, Py-H), 8.34 (d, J = 8 Hz, 1H, Py-H), 7.88 (t, J = 8 Hz, 1H, Py-H), 7.11 (t, J = 12 Hz, 2H, Ph-H), 7.03 (t, J = 16 Hz, 1H, Ph-H), 6.97 (d, J = 8 Hz, 4H, Ph-H), 6.88 (d, J = 8 Hz, 2H, Ph-H), 6.83–6.77 (m, 7H, Ph-H), 6.71 (d, J = 8 Hz, 2H, Ph-H), 6.65 (d, J = 8 Hz, 2H, Ph-H), 6.56 (s, 1H, Ph-H), 5.31 (s, 1H, CH), 5.29 (s, 1H, CH), 3.79 (s, 6H, 2 × OCH3), 3.76 (s, 3H, OCH3), 3.71 (s, 3H, OCH3), 2.50–2.28 (m, 4H, CH2), 2.18 (s, 3H, CH3), 1.94 (s, 3H, CH3), 1.65 (s, 3H, CH3), 1.17 (t, J = 16.0 Hz 3H, CH3), 1.12 (t, J = 16.0 Hz 3H, CH3). 13C NMR (100 MHz, CDCl3, TMS): δ 168.7 (C
N), 167.0 (C
N), 157.9, 157.8, 155.3, 155.1, 147.9, 146.4, 138.8, 137.2, 137.1, 136.8, 136.2, 135.5, 133.5, 131.3, 131.2, 130.7, 130.3, 129.2, 128.6, 126.0, 124.9, 123.4, 122.2, 122.1, 113.6, 113.4, 55.3, 54.8, 50.7, 24.7, 24.6, 18.1, 16.8, 16.7, 13.8. FT-IR (cm−1): 2296(w), 2963(w), 2935(w), 2902(w), 2832(w), 1642 (νC
N, m), 1609 (νC
N, w), 1580(m), 1508(s), 1457(m), 1418(m), 1365(w), 1300(m), 1242(s), 1174(m), 1113(w), 1075(w), 1033(m), 966(w), 872(w), 815(m), 771(m), 654(w). Anal. calcd for C56H57N3O4 (822.06): C, 80.45; H, 6.87; N, 5.03. Found: C, 80.51; H, 6.84; N, 5.05%.
L3: Ar = 2,6-i-Pr2C6H3. Using a similar procedure as described for L1 but with 2,6-diethylaniline as the amine, L2 was isolated as a pale yellow powder (0.23 g, 26.1%). 1H NMR (400 MHz, CDCl3, TMS): δ 8.43 (d, J = 8 Hz, 1H, Py-H), 8.34 (d, J = 8 Hz, 1H, Py-H), 7.88 (t, J = 8 Hz, 1H, Py-H), 7.19–7.16 (m, 2H, Ph-H), 7.10 (t, J = 6 Hz, 1H, Ph-H), 6.97 (d, J = 8 Hz, 4H, Ph-H), 6.88 (d, J = 8 Hz, 2H, Ph-H), 6.84–6.77 (m, 7H, Ph-H), 6.71 (d, J = 8 Hz, 2H, Ph-H), 6.65 (d, J = 8 Hz, 2H, Ph-H), 6.56 (s, 1H, Ph-H), 5.31 (s, 1H, CH), 5.29 (s, 1H, CH), 3.79 (s, 6H, 2 × OCH3), 3.77 (s, 3H, OCH3), 3.71 (s, 3H, OCH3), 2.82–2.71 (m, 2H, –CH), 2.20 (s, 3H, CH3), 1.95 (s, 3H, CH3), 1.67 (s, 3H, CH3), 1.19 (d, J = 8 Hz, 6H, 2 × CH3), 1.15 (d, J = 8 Hz, 6H, 2 × CH3). 13C NMR (100 MHz, CDCl3, TMS): δ 168.6 (C
N), 166.9 (C
N), 157.8, 157.7, 155.2, 155.0, 146.5, 146.3, 138.7, 137.1, 137.0, 136.1, 135.8, 135.4, 133.4, 130.6, 130.2, 129.1, 128.5, 124.8, 123.5, 123.0, 122.1, 122.0, 113.5, 113.3, 55.2, 54.7, 50.6, 28.3, 23.2, 22.9, 18.0, 17.1, 16.6. FT-IR (cm−1): 2961(w), 2904(w), 2833(w), 1636 (νC
N, m), 1609 (νC
N, w), 1582(m), 1508(s), 1461(m), 1416(w), 1365(w), 1301(m), 1242(s), 1115(m), 1072(w), 1034(w), 967(m), 935(w), 875(w), 835(m), 816(m), 773(m), 653(w). Anal. calcd for C58H61N3O4 (864.14): C, 80.62; H, 7.12; N, 4.86. Found: C, 80.35; H, 7.11; N, 4.86%.
L4: Ar = 2,4,6-Me3C6H2. Using a similar procedure as described for L1 but with 2,4,6-trimethylaniline as the amine, L4 was prepared as a pale yellow powder (0.29 g, 24.4%). 1H NMR (400 MHz, CDCl3, TMS): δ 8.43 (d, J = 8 Hz, 1H, Py-H), 8.34 (d, J = 8 Hz, 1H, Py-H), 7.88 (t, J = 8 Hz, 1H, Py-H), 6.97 (d, J = 8 Hz, 4H, Ph-H), 6.89 (d, J = 8 Hz, 4H, Ph-H), 6.83–6.78 (m, 7H, Ph-H), 6.71 (d, J = 8 Hz, 2H, Ph-H), 6.65 (d, J = 8 Hz, 2H, Ph-H), 6.56 (s, 1H, Ph-H), 5.31 (s, 1H, CH), 5.29 (s, 1H, CH), 3.79 (s, 6H, 2 × OCH3), 3.76 (s, 3H, OCH3), 3.71 (s, 3H, OCH3), 2.30 (s, 3H, CH3), 2.17 (s, 3H, CH3), 2.04 (s, 3H, CH3), 2.00 (s, 3H, CH3), 1.94 (s, 3H, CH3), 1.65 (s, 3H, CH3). 13C NMR (100 MHz, CDCl3, TMS): δ 168.6 (C
N), 167.4 (C
N), 157.8, 155.2, 146.4, 146.3, 138.7, 137.0, 136.7, 136.1, 135.4, 133.4, 132.2, 130.6, 130.2, 129.1, 128.6, 125.3, 125.2, 124.7, 122.1, 122.0, 113.5, 113.3, 55.2, 54.7, 50.6, 20.7, 17.9, 17.8, 16.6, 16.4. FT-IR (cm−1): 2994(w), 2951(w), 2907(w), 2834(w), 1641 (νC
N, m), 1608 (νC
N, w), 1577(m), 1507(s), 1460(m), 1364(w), 1326(w), 1298(m), 1242(s), 1174(m), 1119(w), 1115(w), 1034(m), 966(w), 816(w), 779(m), 705(m), 662(w). Anal. calcd for C55H55N3O4 (822.06): C, 80.36; H, 6.74; N, 5.11. Found: C, 80.05; H, 6.80; N, 5.06%.
L5: Ar = 2,6-Et2-4-MeC6H2. Using a similar procedure as described for L1 but with 2,6-diethyl-4-methylaniline as the amine, L5 was prepared as a pale yellow powder (0.31 g, 25.6%). 1H NMR (400 MHz, CDCl3, TMS): δ 8.40 (d, J = 8 Hz, 1H, Py-H), 8.32 (d, J = 8 Hz, 1H, Py-H), 7.85 (t, J = 8 Hz, 1H, Py-H), 6.95 (d, J = 8 Hz, 6H, Ph-H), 6.86 (d, J = 8 Hz, 2H, Ph-H), 6.81–6.75 (m, 7H, Ph-H), 6.69 (d, J = 8 Hz, 2H, Ph-H), 6.63 (d, J = 8 Hz, 2H, Ph-H), 6.54 (s, 1H, Ph-H), 5.29 (s, 1H, CH), 5.27 (s, 1H, CH), 3.77 (s, 6H, 2 × OCH3), 3.74 (s, 3H, OCH3), 3.69 (s, 3H, OCH3), 2.42–2.23 (m, 4H, CH2), 2.34 (s, 3H, CH3), 2.16 (s, 3H, CH3), 1.92 (s, 3H, CH3), 1.63 (s, 3H, CH3), 1.14 (t, J = 8.0 Hz 3H, CH3), 1.09 (t, J = 8.0 Hz 3H, CH3). 13C NMR (100 MHz, CDCl3, TMS): δ 168.6 (C
N), 167.1 (C
N), 157.8, 157.7, 155.2, 146.4, 145.3, 138.7, 137.1, 136.7, 136.1, 135.4, 133.4, 132.4, 131.1, 130.6, 130.2, 129.1, 128.5, 126.7, 124.9, 122.0, 113.5, 113.3, 55.2, 54.7, 50.6, 24.6, 24.5, 21.0, 17.8, 16.7, 16.6, 13.9, 13.8. FT-IR (cm−1): 2994(w), 2962(w), 2904(w), 2833(w), 1641 (νC
N, m), 1607 (νC
N, w), 1577(w), 1507(s), 1459(m), 1418(w), 1364(w), 1298(m), 1244(s), 1175(m), 1116(w), 1075(w), 1034(m), 965(w), 856(w), 814(m), 775(m), 745(w). Anal. calcd for C57H59N3O4 (850.12): C, 80.53; H, 7.00; N, 4.94. Found: C, 80.16; H, 7.02; N, 4.93%.
Synthesis of [2-[CMeN{2,4-{(4-MeOC6H4)2CH}2-6-Me}]-6-(CMeNAr)-C5H3N]CoCl2.
Co1: Ar = 2,6-Me2C6H3. To a mixture of L1 (0.202 g, 0.25 mmol) and CoCl2 (0.031 g, 0.24 mmol) was added dichloromethane and ethanol (5/10 mL). The reaction mixture was stirred for 24 h at ambient temperature, and excess diethyl ether added to precipitate the product. The precipitate was collected by filtration, washed with diethyl ether (3 × 10 mL) and then dried under reduced pressure to give Co1 as a brown solid (0.125 g, 56%). 1H NMR (400 MHz, CDCl3, TMS): δ 110.59 (S, 1H, Py-H), 108.82 (S, 1H, Py-H), 39.82 (S, 1H, Py-H), 18.23 (S, 2H, Ph-H), 9.90–8.96 (m, 16H, Ph-H), 7.55 (S, 2H, Ph-H), 5.13 (S, 6H, 2 × OCH3), 5.08 (S, 6H, 2 × OCH3), 4.16 (S, 3H, CH3), 2.27 (S, 3H, CH3), 1.84 (S, 3H, CH3), −10.64 (S, 1H, Ph-H), −20.99 (S, 2H, 2 × CH), −24.59 (S, 3H, CH3), −24.87 (S, 3H, CH3). FT-IR (cm−1): 2997(w), 2952(w), 2907(w), 2834(w), 1609 (νC
N, m), 1585 (νC
N, w), 1507(s), 1464(m), 1442(w), 1370(w), 1299(m), 1175(m), 1109(w), 1031(w), 981(m), 812(w), 771(w), 672(m). Anal. calcd for C54H53Cl2CoN3O4 (937.87): C, 69.16; H, 5.70; N, 4.48. Found: C, 69.17; H, 5.74; N, 4.52%.
Co2: Ar = 2,6-Et2C6H3. The synthesis of Co2 was carried out using a procedure and molar ratios similar to that described for Co1, but with L2 used in place of L1. Following work-up, Co2 was isolated as brown powder (0.11 g, 89%). 1H NMR (400 MHz, CDCl3, TMS): δ 110.30 (S, 1H, Py-H), 109.34 (S, 1H, Py-H), 43.04 (S, 1H, Py-H), 19.33 (S, 2H, Ph-H), 9.90–8.96 (m, 16H, Ph-H), 8.43 (S, 2H, Ph-H), 5.26 (S, 6H, 2 × OCH3), 5.21 (S, 6H, 2 × OCH3), 4.49 (S, 3H, CH3), 2.42 (S, 3H, CH3), 1.76 (S, 3H, CH3), −9.42 (S, 1H, Ph-H), −18.90 (S, 6H, 2×CH3), −20.73 (S, 4H, 2 × CH2), −23.02 (S, 2H, 2 × CH). FT-IR (cm−1): 2962(w), 2903(w), 2833(w), 1609 (νC
N, m), 1584 (νC
N, w), 1508(s), 1462(m), 1444(w), 1371(w), 1300(m), 1246(s), 1177(m), 1109(w), 1031(m), 981(w), 832(w), 811(m), 773(m), 667(w). Anal. calcd for C56H57Cl2CoN3O4 (965.92): C, 69.63; H, 5.95; N, 4.35. Found: C, 69.48; H, 5.89; N, 4.32%.
Co3: Ar = 2,6-i-Pr2C6H3. The synthesis of Co3 was carried out using a procedure and molar ratios similar to that described for Co1, but with L3 used in place of L1. Following work-up, Co3 was isolated as brown powder (0.07 g, 72%). 1H NMR (400 MHz, CDCl3, TMS): δ 110.96 (S, 1H, Py-H), 109.53 (S, 1H, Py-H), 47.46 (S, 1H, Py-H), 19.95 (S, 2H, Ph-H), 10.01–9.44 (m, 8H, Ph-H), 6.99–6.57 (m, 8H, Ph-H), 8.43 (S, 2H, Ph-H), 5.47 (S, 6H, 2 × OCH3), 5.26 (S, 6H, 2 × OCH3), 3.71 (S, 3H, CH3), 3.80 (S, 3H, CH3), 3.16 (S, 3H, CH3), −8.15 (S, 1H, Ph-H), −12.71 (S, 2H, 2 × CH), −13.08 (S, 6H, 2 × CH3), −19.99 (S, 6H, 2 × CH3), −23.16 (S, 2H, 2 × CH). FT-IR (cm−1): 2960(w), 2906(w), 2873(w), 2834(w), 1609 (νC
N, m), 1584 (νC
N, w), 1508(s), 1462(m), 1444(w), 1369(w), 1300(m), 1245(s), 1175(m), 1109(w), 1031(m), 936(w), 832(w), 812(m), 771(m), 726(w). Anal. calcd for C58H61Cl2CoN3O4 (993.98): C, 70.09; H, 6.19; N, 4.23. Found: C, 69.80; H, 6.33; N, 4.33%.
Co4: Ar = 2,4,6-Me3C6H2. The synthesis of Co4 was carried out using a procedure and molar ratios similar to that described for Co1, but with L4 used in place of L1. Following work-up, Co4 was isolated as brown powder (0.18 g, 71%). 1H NMR (400 MHz, CDCl3, TMS): δ 109.38 (S, 1H, Py-H), 109.00 (S, 1H, Py-H), 41.05 (S, 1H, Py-H), 19.76 (S, 3H, CH3), 18.37 (S, 2H, Ph-H), 10.14–7.86 (m, 16H, Ph-H), 7.97 (S, 1H, Ph-H), 7.88 (S, 1H, Ph-H), 5.18 (S, 6H, 2 × OCH3), 5.11 (S, 6H, 2 × OCH3), 4.21 (S, 3H, CH3), 2.70 (S, 3H, CH3), 1.83 (S, 3H, CH3), −21.48 (S, 2H, 2 × CH), −24.01 (S, 3H, CH3), −24.23 (S, 3H, CH3). FT-IR (cm−1): 2990(w), 2905(w), 2835(w), 1610 (νC
N, m), 1585 (νC
N, w), 1509(s), 1464(m), 1444(w), 1372(w), 1300(m), 1248(s), 1178(m), 1109(w), 1033(m), 834(w), 813(w), 777(m), 672(w). Anal. calcd for C55H55Cl2CoN3O4 (951.90): C, 69.40; H, 5.82; N, 4.41. Found: C, 69.45; H, 5.84; N, 4.44%.
Co5: Ar = 2,6-Et2-4-MeC6H2. The synthesis of Co5 was carried out using a procedure and molar ratios similar to that described for Co1, but with L5 used in place of L1. Following work-up, Co5 was isolated as brown powder (0.10 g, 80%). 1H NMR (400 MHz, CDCl3, TMS): δ 109.14 (S, 2H, Py-H), 44.09 (S, 1H, Py-H), 20.26 (S, 3H, CH3), 18.91 (S, 2H, Ph-H), 10.75–9.06 (m, 16H, Ph-H), 8.41 (S, 2H, Ph-H), 5.25 (S, 6H, 2 × OCH3), 5.21 (S, 6H, 2 × OCH3), 4.48 (S, 3H, CH3), 2.32 (S, 3H, CH3), 1.68 (S, 3H, CH3), −19.05 (S, 6H, 2 × CH3), −21.11 (S, 4H, 2 × CH2), −22.08 (S, 2H, 2 × CH). FT-IR (cm−1): 2980(w), 2902(w), 2833(w), 1609 (νC
N, m), 1584 (νC
N, w), 1508 (s), 1461(m), 1411(w), 1374(w), 1300(m), 1248(s), 1177(m), 837(w), 813(m), 777(m), 674(w). Anal. calcd for C57H59Cl2CoN3O4 (979.95): C, 69.86; H, 6.07; N, 4.29. Found: C, 69.87; H, 6.07; N, 4.34%.
X-ray crystallographic studies
The single-crystals diffraction studies for Co2 and Co4 were performed using a Rigaku Sealed Tube CCD (Saturn 724+) diffractometer with graphite-monochromated Cu-Kα radiation (λ = 1.54184 Å) at 173(2) K. Cell parameters were obtained by global refinement of the positions of all collected reflections. Intensities were corrected for Lorentz and polarization effects and empirical absorption. The structures were solved by direct methods and refined by full matrix least squares on F2. All hydrogen atoms were placed in calculated positions. Structure solution and refinement were performed by using the SHELXL-97 package. Crystal data and structure refinements for Co2 and Co4 are shown in Table S4.†21 CCDC 2027357 and 2027358 contain the supplementary crystallographic data for complexes Co2 and Co4, respectively.†
Polymerization studies
Ethylene polymerization at 1 atm C2H4. Precatalyst Co1 (1.4 mg, 1.5 μmol) was added to a Schlenk vessel with a magnet stir bar followed by freshly distilled toluene (30 mL) and then the required amount of aluminum activator (MAO, MMAO) was introduced via syringe. The solution was afterwards stirred at 50 °C under 1 atm of C2H4 introduced by a rubber gas bag. After 30 min, the pressure was vented and the reaction quenched with acidified ethanol (ethanol/HCl = 50/1). The suspension was stirred for 1 h, after that the precipitated polymer was washed with ethanol, dried under vacuum at 50 °C and then weighed.
Ethylene polymerization at 5/10 atm C2H4. The polymerization at high ethylene pressure was carried out in a stainless-steel autoclave (250 mL) equipped with an ethylene pressure controller, a mechanical agitator and a thermoregulatory. At the desired reaction temperature the precatalyst (Co1–Co5: 1.5 μmol) dissolved in toluene (50 mL) was injected into the autoclave, followed by freshly distilled toluene (25 mL). The required amount of aluminum activator like MAO, MMAO, and more toluene (25 mL) were then injected successively to complete the addition. With rapid stirring, the autoclave was immediately pressurized to the setting ethylene pressure (5/10 atm). After a prescribed reaction time, the ethylene pressure was vented and the reaction quenched with acidified ethanol (ethanol/HCl = 50/1). The suspension was stirred for 1 h, and then the precipitated polymer was filtered and washed with anhydrous ethanol and dried under vacuum at 50 °C and weighed.
Conclusions
A series of unsymmetrical 2-(2,4-bis(di(4-methoxyphenyl)methyl)-6-MeC6H2N)-6-(1-(arylimino)ethyl)pyridine cobalt complexes has been synthesized and fully characterized. The molecular structures of the representative cobalt complexes Co2 and Co4 were obtained by the single-crystal X-ray diffraction method, showing distorted square-pyramidal geometry at the metal. Activated with either MAO or MMAO, all precatalysts Co1–Co5 displayed high activities towards ethylene polymerization, especially when using Co1/MAO (Al/Co = 3250 at 50 °C under 10 atm ethylene), up to 9.17 × 106 g PE (mol of Co)−1 h−1) producing strictly linear polyethylene with high molecular weight (3.14 × 105 g mol−1) and wide polydispersity (54.6). In particular, regardless of whether Co/MAO or Co/MMAO catalytic system, ethylene undergoes β-hydride elimination reaction pathway during polymerization, resulting in vinyl-polyethylene. Therefore, the introduction of methoxy to the benzhydryl group results in a significant increase in the molecular weight of the produced polyethylene as well as the wide polydispersities (around 50), the first time obtaining the high-density polyethylene with wide polydispersity similar to ones produced by chromium Phillips catalysis.10 The less toxic cobalt catalysts potentially replace Phillips catalysts in commercial application for the polydispersive polyethylenes.
Conflicts of interest
There are no conflicts to declare.
Acknowledgements
This work was supported by the National Natural Science Foundation of China (No. 21871275).
Notes and references
-
(a) G. J. P. Britovsek, V. C. Gibson, B. S. Kimberley, P. J. Maddox, S. J. McTavish, G. A. Solan, A. J. P. White and D. J. Williams, Chem. Commun., 1998, 7, 849–850 RSC;
(b) B. L. Small, M. Brookhart and A. M. A. Bennett, J. Am. Chem. Soc., 1998, 120, 4049–4050 CrossRef CAS;
(c) G. J. P. Britovsek, M. Bruce, V. C. Gibson, B. S. Kimberley, P. J. Maddox, S. Mastroianni, S. J. McTavish, C. Redshaw, G. A. Solan, S. Strömberg, A. J. P. White and D. J. Williams, J. Am. Chem. Soc., 1999, 121, 8728–8740 CrossRef CAS.
-
(a) Z. Flisak and W.-H. Sun, ACS Catal., 2015, 5, 4713–4724 CrossRef CAS;
(b) Z. Wang, G. A. Solan, W. Zhang and W.-H. Sun, Coord. Chem. Rev., 2018, 363, 92–108 CrossRef CAS;
(c) C. Bariashir, C. Huang, G. A. Solan and W.-H. Sun, Coord. Chem. Rev., 2019, 385, 208–229 CrossRef CAS;
(d) S. Yuan, Y. Yan, G. A. Solan, Y. Ma and W.-H. Sun, Coord. Chem. Rev., 2020, 411, 213254 CrossRef CAS.
-
(a) W.-H. Sun, P. Hao, S. Zhang, Q. Shi, W. Zuo, X. Tang and X. Lu, Organometallics, 2007, 26, 2720–2734 CrossRef CAS;
(b) Y. Chen, P. Hao, W. Zuo, K. Gao and W.-H. Sun, J. Organomet. Chem., 2008, 693, 1829–1840 CrossRef CAS;
(c) R. Gao, Y. Li, F. Wang, W.-H. Sun and M. Bochmann, Eur. J. Inorg. Chem., 2009, 4149–4156 CrossRef CAS;
(d) L. Xiao, R. Gao, M. Zhang, Y. Li, X. Cao and W.-H. Sun, Organometallics, 2009, 28, 2225–2233 CrossRef CAS;
(e) W. Lin, L. Zhang, J. Gao, Q. Zhang, Y. Ma, H. Liu and W.-H. Sun, Molecules, 2020, 25, 4244 CrossRef CAS;
(f) W. Lin, L. Zhang, H. Suo, A. Vignesh, N. Yousuf, X. Hao and W.-H. Sun, New J. Chem., 2020, 44, 8076–8084 RSC.
- K. Wang, K. Wedeking, W. Zuo, D. Zhang and W.-H. Sun, J. Organomet. Chem., 2008, 693, 1073–1080 CrossRef CAS.
- S. Zhang, W.-H. Sun, T. Xiao and X. Hao, Organometallics, 2010, 29, 1168–1173 CrossRef CAS.
-
(a) J. D. A. Pelletier, Y. D. M. Champouret, J. Cadarso, L. Clowes, M. Gañete, K. Singh, V. Thanarajasingham and G. A. Solan, J. Organomet. Chem., 2006, 691, 4114–4123 CrossRef CAS;
(b) S. Jie, S. Zhang, K. Wedeking, W. Zhang, H. Ma, X. Lu, Y. Deng and W.-H. Sun, C. R. Chim., 2006, 9, 1500–1509 CrossRef CAS;
(c) S. Jie, S. Zhang and W.-H. Sun, Eur. J. Inorg. Chem., 2007, 5584–5598 CrossRef CAS;
(d) M. Zhang, R. Gao, X. Hao and W.-H. Sun, J. Organomet. Chem., 2008, 693, 3867–3877 CrossRef CAS;
(e) J. Guo, W. Zhang, T. Liang and W.-H. Sun, Polyhedron, 2020, 193, 114865 CrossRef.
-
(a) W. Zhang, W.-H. Sun and C. Redshaw, Dalton Trans., 2013, 42, 8988–8997 RSC;
(b) B. Gansukh, R. Zhang, J. Guo, W. Zhang and W.-H. Sun, Gen. Chem., 2020, 6, 190031–190039 Search PubMed.
-
(a) S. Jie, W.-H. Sun and T. Xiao, Chin. J. Polym. Sci., 2010, 28, 299–304 CrossRef CAS;
(b) T. Xiao, W. Zhang, J. Lai and W.-H. Sun, C. R. Chim., 2011, 14, 851–855 CrossRef CAS;
(c) Z. Wang, G. A. Solan, Y. Ma, Q. Liu, T. Liang and W.-H. Sun, Research, 2019, 2019, 1–15 Search PubMed;
(d) C. Bariashir, Z. Wang, Y. Ma, A. Vignesh, X. Hao and W.-H. Sun, Organometallics, 2019, 38, 4455–4470 CrossRef CAS;
(e) J. Guo, W. Zhang, I. I. Oleynik, G. A. Solan, I. V. Oleynik, T. Liang and W.-H. Sun, Dalton Trans., 2020, 49, 136–146 RSC;
(f) M. Han, Q. Zhang, I. I. Oleynik, H. Suo, G. A. Solan, I. V. Oleynik, Y. Ma, T. Liang and W.-H. Sun, Dalton Trans., 2020, 49, 4774–4784 RSC.
-
(a) J. Ba, S. Du, E. Yue, X. Hu, Z. Flisak and W.-H. Sun, RSC Adv., 2015, 5, 32720–32729 RSC;
(b) V. K. Appukuttan, Y. Liu, B. C. Son, C.-S. Ha, H. Suh and I. Kim, Organometallics, 2011, 30, 2285–2294 CrossRef CAS;
(c) W.-H. Sun, S. Kong, W. Chai, T. Shiono, C. Redshaw, X. Hu, C. Guo and X. Hao, Appl. Catal. Gen., 2012, 447–448, 67–73 CrossRef CAS;
(d) F. Huang, W. Zhang, E. Yue, T. Liang, X. Hu and W.-H. Sun, Dalton Trans., 2016, 45, 657–666 RSC;
(e) S. Du, W. Zhang, E. Yue, F. Huang, T. Liang and W.-H. Sun, Eur. J. Inorg. Chem., 2016, 1748–1755 CrossRef CAS;
(f) Z. Wang, G. A. Solan, Q. Mahmood, Q. Liu, Y. Ma, X. Hao and W.-H. Sun, Organometallics, 2018, 37, 380–389 CrossRef CAS;
(g) Q. Zhang, N. Wu, J. Xiang, G. A. Solan, H. Suo, Y. Ma, T. Liang and W.-H. Sun, Dalton Trans., 2020, 49, 9425–9437 RSC;
(h) Q. Zhang, R. Zhang, M. Han, W. Yang, T. Liang and W.-H. Sun, Dalton Trans., 2020, 49, 7384–7396 RSC.
- P. M. Max, Adv. Catal., 2010, 53, 123–606 Search PubMed.
-
(a) S. Wang, B. Li, T. Liang, C. Redshaw, Y. Li and W.-H. Sun, Dalton Trans., 2013, 42, 9188–9197 RSC;
(b) F. He, W. Zhao, X.-P. Cao, T. Liang, C. Redshaw and W.-H. Sun, J. Organomet. Chem., 2012, 713, 209–216 CrossRef CAS;
(c) Q. Mahmood, Y. Ma, X. Hao and W.-H. Sun, Appl. Organomet. Chem., 2019, 33, e4857 CrossRef;
(d) R. Zhang, Y. Ma, M. Han, G. A. Solan, Y. Pi, Y. Sun and W.-H. Sun, Appl. Organomet. Chem., 2019, 33, e5175 Search PubMed;
(e) L. Guo, H. Gao, L. Zhang, F. Zhu and Q. Wu, Organometallics, 2010, 29, 2118–2125 CrossRef CAS;
(f) W. Zhao, E. Yue, X. Wang, W. Yang, Y. Chen, X. Hao, X. Cao and W.-H. Sun, J. Polym. Sci., Part A: Polym. Chem., 2017, 55, 988–996 CrossRef CAS;
(g) A. S. Ionkin, W. J. Marshall, D. J. Adelman, B. B. Fones, B. M. Fish and M. F. Schiffhauer, Organometallics, 2008, 27, 1902–1911 CrossRef CAS;
(h) R. Zhang, M. Han, Y. Ma, G. A. Solan, T. Liang and W.-H. Sun, Dalton Trans., 2019, 48, 17488–17498 RSC.
-
(a) J. Yu, W. Huang, L. Wang, C. Redshaw and W.-H. Sun, Dalton Trans., 2011, 40, 10209–10214 RSC;
(b) S. Wang, W. Zhao, X. Hao, B. Li, C. Redshaw, Y. Li and W.-H. Sun, J. Organomet. Chem., 2013, 731, 78–84 CrossRef CAS;
(c) J. Lai, W. Zhao, W. Yang, C. Redshaw, T. Liang, Y. Liu and W.-H. Sun, Polym. Chem., 2012, 3, 787 RSC;
(d) W. Zhang, S. Wang, S. Du, C.-Y. Guo, X. Hao and W.-H. Sun, Macromol. Chem. Phys., 2014, 215, 1797–1809 CrossRef CAS;
(e) S. Du, S. Kong, Q. Shi, J. Mao, C. Guo, J. Yi, T. Liang and W.-H. Sun, Organometallics, 2015, 34, 582–590 CrossRef CAS.
-
(a) S. Meiries, K. Speck, D. B. Cordes, A. M. Z. Slawin and S. P. Nolan, Organometallics, 2012, 32, 330–339 CrossRef;
(b) S.-F. Yuan, Z. Fan, Q. Zhang, Z. Flisak, Y. Ma, Y. Sun and W.-H. Sun, Appl. Organomet. Chem., 2020, 34, e5638 CAS.
-
(a) G. J. P. Britovsek, V. C. Gibson, B. S. Kimberley, S. Mastroianni, C. Redshaw, G. A. Solan, A. J. P. White and D. J. Williams, J. Chem. Soc., Dalton Trans., 2001, 10, 1639–1644 RSC;
(b) Q. Zhang, Y. Ma, H. Suo, G. A. Solan, T. Liang and W.-H. Sun, Appl. Organomet. Chem., 2019, 33, e5134 Search PubMed;
(c) Z. Wang, Y. Ma, J. Guo, Q. Liu, G. A. Solan, T. Liang and W.-H. Sun, Dalton Trans., 2019, 48, 2582–2591 RSC;
(d) H. Suo, Z. Li, I. V. Oleynik, Z. Wang, I. I. Oleynik, Y. Ma, Q. Liu and W.-H. Sun, Appl. Organomet. Chem., 2020, 34, e5937 CrossRef CAS.
-
(a) F. Huang, W. Zhang, Y. Sun, X. Hu, G. A. Solan and W.-H. Sun, New J. Chem., 2016, 40, 8012–8023 RSC;
(b) H. Suo, I. I. Oleynik, C. Bariashir, I. V. Oleynik, Z. Wang, G. A. Solan, Y. Ma, T. Liang and W.-H. Sun, Polymer, 2018, 149, 45–54 CrossRef CAS;
(c) J. Guo, Z. Wang, W. Zhang, I. I. Oleynik, A. Vignesh, I. V. Oleynik, X. Hu, Y. Sun and W.-H. Sun, Molecules, 2019, 24, 1176 CrossRef;
(d) C. Bariashir, Z. Wang, H. Suo, M. Zada, G. A. Solan, Y. Ma, T. Liang and W.-H. Sun, Eur. Polym. J., 2019, 110, 240–251 CrossRef CAS;
(e) Y. Huang, R. Zhang, T. Liang, X. Hu, G. A. Solan and W.-H. Sun, Organometallics, 2019, 38, 1143–1150 CrossRef CAS;
(f) M. Zada, A. Vignesh, H. Suo, Y. Ma, H. Liu and W.-H. Sun, Mol. Catal., 2020, 492, 110981 CrossRef CAS;
(g) M. Han, Q. Zhang, I. I. Oleynik, H. Suo, I. V. Oleynik, G. Solan, Y. Ma, T. Liang and W.-H. Sun, Catalysts, 2020, 10, 1002 CrossRef CAS.
- S. Du, X. Wang, W. Zhang, Z. Flisak, Y. Sun and W.-H. Sun, Polym. Chem., 2016, 7, 4188–4197 RSC.
-
(a) Z. Long, B. Wu, P. Yang, G. Li, Y. Liu and X.-J. Yang, J. Organomet. Chem., 2009, 694, 3793–3799 CrossRef CAS;
(b) C. Huang, S. Du, G. A. Solan, Y. Sun and W.-H. Sun, Dalton Trans., 2017, 46, 6948–6957 RSC.
- Q. Mahmood, E. Yue, J. Guo, W. Zhang, Y. Ma, X. Hao and W.-H. Sun, Polymer, 2018, 159, 124–137 CrossRef CAS.
-
(a) W. Zhao, J. Yu, S. Song, W. Yang, H. Liu, X. Hao, C. Redshaw and W.-H. Sun, Polymer, 2012, 53, 130–137 CrossRef CAS;
(b) X. Cao, F. He, W. Zhao, Z. Cai, X. Hao, T. Shiono, C. Redshaw and W.-H. Sun, Polymer, 2012, 53, 1870–1880 CrossRef CAS.
-
(a) L. Guo, M. Zada, W. Zhang, A. Vignesh, D. Zhu, Y. Ma, T. Liang and W.-H. Sun, Dalton Trans., 2019, 48, 5604–5613 RSC;
(b) M. Zada, L. Guo, Y. Ma, W. Zhang, Z. Flisak, Y. Sun and W.-H. Sun, Molecules, 2019, 24, 2007 CrossRef CAS;
(c) R. Zhang, Y. Huang, G. A. Solan, W. Zhang, X. Hu, X. Hao and W.-H. Sun, Dalton Trans., 2019, 48, 8175–8185 RSC.
-
(a) G. M. Sheldrick, Acta Crystallogr., Sect. C: Struct. Chem., 2015, 71, 3–8 Search PubMed;
(b) G. M. Sheldrick, Acta Crystallogr., Sect. A: Found. Adv., 2015, 71, 3–8 CrossRef.
Footnotes |
† Dedicated to Prof. Dr Sabu Thomas on his 60th birthday. |
‡ Electronic supplementary information (ESI) available: NMR charts of cobalt complexes and representative polymeric samples as well as crystal data and structure refinements for Co2 and Co4. CCDC 2027357 (Co2) and 2027358 (Co4). For ESI and crystallographic data in CIF or other electronic format see DOI: 10.1039/d0ra09333e |
|
This journal is © The Royal Society of Chemistry 2020 |