DOI:
10.1039/D0RA07960J
(Paper)
RSC Adv., 2020,
10, 44058-44065
Retracted Article: Highly sensitive cadmium sulphide quantum dots as a fluorescent probe for estimation of doripenem in real human plasma: application to pharmacokinetic study†
Received
17th September 2020
, Accepted 18th November 2020
First published on 11th December 2020
Abstract
Thioglycolic acid-capped cadmium sulphide quantum dots (TGA-CdS QDs) have been synthesized and utilized as a fluorescent probe for the estimation of doripenem (DOR). Monitoring of DOR in different biological fluids is required to estimate the efficient dose to avoid bacterial infections and resistance. The investigated method is based on the measurement of fluorescence quenching of TGA-CdS QDs after the addition of DOR. The synthesized TGA-CdS QDs were characterized using transmission electron microscopy (TEM), Fourier transform infrared (FTIR) spectroscopy, X-ray powder diffraction (XRD) and ZETA sizer. The TGA-CdS QDs showed unique photophysical properties with high quantum yield (0.32) using a comparison method with rhodamine B. Different experimental parameters affecting the synthesis process of the TGA-CdS QDs and their behavior with the studied drug DOR were examined and optimized. The values of the fluorescence quenching were linearly correlated to DOR concentration over the range of 10–500 ng mL−1 with a good correlation coefficient of 0.9991. The proposed method showed higher sensitivity over several reported methods, with LOD reaching 2.0 ng mL−1. The method was effectively applied for the estimation of DOR in human plasma and urine with good recovery results ranged from 95.16% to 99.51%. Furthermore, the stability of DOR in the human plasma was studied and a pharmacokinetic study of DOR in real human plasma was conducted.
1. Introduction
Doripenem monohydrate (DOR) is (+)-(4R5S,6S)-6-[(1R)-1-hydroxyethyl]-4-d-thazabicyclo[3.2.0]hept-2-ene-2-carboxylic acid,1 β-lactam antibiotic which belongs to the carbapenem subclass. DOR inhibits the bacterial cell wall synthesis by inactivating the essential penicillin-binding proteins (PBPs), which ultimately cause cell death. Carbapenem antibiotics play an extremely important role in the treatment of severe infections such as pneumonia and urinary infections. The emergence of strains resistant to certain antibiotics is turning into an important issue worldwide; which is consequently related to the increase in mortality rates and threaten the global health. Hence, monitoring of antimicrobial drugs such as DOR in different biological fluids is very important to estimate the efficient dose and to avoid bacterial resistance.
From the literature survey, different analytical methods have been reported for the analysis of DOR such as spectrophotometry,2–5 spectrofluorimetry,6 high-performance liquid chromatography (HPLC),7–9 high-performance thin layer chromatography (HPTLC)10 and electrochemical11 methods. From this survey, only one spectrofluorimetric method was reported for the determination of DOR.6 Moreover, the utility of fluorescent nanostructures such as either gold or silver nanoparticles,12 or quantum dots (QDs) have increased in the last few years due to their extraordinary luminescence emission characters. QDs have broad excitation and narrow emission spectrum13 and have emerged as magnetic fluorescent nano-substances. The QDs were utilized in varying fields such as bio-sensing, photo-catalysis, bio-imaging and biological labeling due to their size-dependent optical properties. Owing to their properties, the QDs are considered as a promising photo-luminescent probe for the determination of pharmaceuticals and biologically active substances.14–17
In the present study, water-soluble thioglycolic acid capped cadmium sulphide quantum dots (TGA-CdS QDs) was synthesized and utilized as an ultra-sensitive fluorescent probe for the estimation of DOR in its pure form, pharmaceutical vial and biological fluids. The spectrum of TGA-CdS QDs has distinct broad absorbance and narrow emission, which facilitates the analysis of multi-target analytes.15–17 The synthesized TGA-CdS QDs showed unique photo-physical properties with high quantum yield (0.32) after the reaction with DOR using a comparison method with rhodamine B.16 Different parameters affecting the synthesis process of the TGA-CdS QDs and their behavior with the studied drug DOR were studied and optimized. Furthermore, the synthesized TGA-CdS QDs was characterized using Transmission electron microscopy (TEM), Fourier transform infrared (FTIR) spectroscopy, ZETA sizer and X-ray powder diffraction (XRD). Further, the TGA-CdS QDs was utilized as fluorescence probe for sensitive and cost-effective spectrofluorimetric determination of DOR in its pure form and pharmaceutical dosage form. Further, the method was applied for determination of DOR in real human plasma and human urine. Moreover, this work was extended to the pharmacokinetic study of DOR, which is very important to avoid bacterial resistance and for effective therapeutic drug monitoring dose.
2. Materials and methods
2.1. Instruments
Transmission electron microscope (TEM) JEOL JEM-100CX II tungsten-filament 100 kV. Digital imaging with GatanErlangshen ES500W camera, Gatan Digital Micrograph software. Fourier transform infrared (FT-IR) spectra was measured using a Nicolet™ iS™10 FTIR spectrometer in the wavenumber range of 400–4000 cm−1 Thermo Scientific, USA). The X-ray powder diffraction was measured using a Philips X-ray PW 1710 with Cu Kα radiation (λ = 1.5405 Å) with 40 kV and 30 mA. The scanning rate was maintained at 0.06° per minute, in the 2θ range of 4–80°. Quantum dots particle size were measured using Zeta sizer Nano ZS (Malvern, UK). pH measurements were performed using pH Meter PCE-PHM 14 (PCE Instruments, UK).
2.2. Chemicals and reagents
Doripenem (DOR, purity 99.9%) was purchased from Sigma Aldrich Chemie GmbH (Steinheim, Germany). Doripax® 500 mg vials were obtained from the local market of Assuit, Egypt. Cadmium chloride (CdCl2), sodium hydroxide (NaOH) and sodium sulfide (Na2S) were obtained from EL-Nasr Company (Egypt). Rhodamine B was obtained from Acros Organics (USA). Thioglycolic acid (TGA) was purchased from Sigma Aldrich Chemie GmbH (Steinheim, Germany).
A stock solution of DOR (1.0 mg mL−1) was prepared by precisely weighing 10.0 mg of DOR, transferring it into 10 mL volumetric flask and dissolving it using distilled water.
TGA-CdS QDs was synthesized following the method of Wang et al.,17 the synthesis procedure and preparation of different reagent solutions are described in details in the ESI.† All the experiments included in this study followed the Assiut University and Al-Azhar University guidelines.
2.3. General analytical procedure
1.5 mL of TGA-CdS QDs and 2.0 mL of Tris–HCl (0.08 M) buffer were added into a 10 mL volumetric flask. One milliliter of the working solution of DOR was added in the flask, and the reaction was allowed to proceed at room temperature for 5 min; subsequently volume of the flask was made up to 10 mL with distilled water. The quenching effect of DOR on the fluorescence intensity of the TGA-CdS QDS was measured at 490 nm after excitation at 350 nm against blank.
2.4. Application of the proposed method
2.4.1. Estimation of DOR in pharmaceutical vial. Precise amount equivalent to 5.0 mg from the Doripax® 500 mg vial was transferred into 50 mL volumetric flask containing 20 mL distilled water, the contents were dissolved and the volume was adjusted to 50 mL with distilled water. One milliliter of the prepared solution was transferred and introduced to the general analytical procedure described in Section 2.3.
2.4.2. Estimation of DOR in human plasma. Three hundred microliters of plasma collected from three volunteers were spiked with 1.0 mL of DOR standard solution, followed by further addition of 1.0 mL of acetonitrile for protein precipitation.6 After that, the solution was adjusted to the mark with distilled water and centrifuged at 5000 rpm for 15 min. One milliliter of the supernatant was transferred and introduced into the general analytical procedure described in Section 2.3.
2.4.3. Estimation of DOR in human urine. Five hundred microliters of human urine collected from three volunteers were spiked with 1.0 mL of DOR stock solutions in centrifugation tubes and mixed well. Then, the centrifugation tubes were volume adjusted to 10 mL with distilled water and subsequently centrifuged at 5000 rpm for 15 min. One milliliter of the supernatant was transferred and introduced into the general analytical procedure described in Section 2.3.
2.4.4. Pharmacokinetic study for DOR. Ten healthy human volunteers were administered Doribax® vial (500 mg DOR), as a single infusion dose for 30 min. Five milliliters of human blood were drawn after certain intervals (0.25, 0.5, 1.0, 1.5, 2.0, 2.5, 3.0, 3.5, 4.0, 4.5, 5.0, 5.5, 6.0) h into a heparinized centrifugation tube and centrifuged at 5000 rpm for 15 min. Further, one milliliter of plasma was placed into a centrifugation tube, and then 1.0 mL of acetonitrile was added for protein precipitation.6 After that, the solution was adjusted to the mark with distilled water and centrifuged at 5000 rpm for 15 min. One milliliter of the supernatant was transferred and introduced into the general procedure of Section 2.3.The applications on plasma and urine samples were conducted in accordance with the Declaration of Helsinki and approved by the Egyptian Network of Research Ethics Committees (ENREC) and informed consent was obtained for any experiments with human subjects.
2.5. Stability of DOR in human plasma
Different factors were applied to study the stability of DOR in human plasma using three different concentration levels, low quality control (LQC 10 ng mL−1), medium quality control (MQC 100 ng mL−1) and high-quality control (HQC 500 ng mL−1) levels such as:
Three Freeze–thaw cycle stability at −24 °C, it was used to study the effect of change in the temperature in the stored samples and it measured the DOR stability in human plasma. This condition depended on freezing the plasma samples containing DOR at −24 °C and then allowing the samples to thaw at room temperature for 3 successive cycles.
Long-term stability, plasma samples containing DOR were placed at −24 °C for 1 month and then the samples were thawed at room temperature.
Short-term stability, DOR plasma samples were placed at −24 °C for 12 h and then the samples were thawed at room temperature.
Post-preparative stability, DOR plasma samples were placed at room temperature 25 °C for 6 and 12 h.
3. Results and discussion
3.1. Fluorescence characteristics of the synthesized TGA-CdSQDs
DOR is a broad-spectrum β-lactam antibiotic belonging to the carbapenem subclass, and it is used for treatment of several bacterial infections. As the emergence of bacterial strains resistant to certain antibiotics is threating the global health, monitoring of antimicrobial drugs such as DOR in different biological fluids is necessary for the dose adjustment and to avoid bacterial resistance. From the literature survey, only one spectrofluorimetric method was found for the determination of DOR.6 As it is well known, spectrofluorimetry is an interesting analytical technique due to its sensitivity, selectivity and its wide availability. In addition, utility of different fluorescent probes have emerged as a dynamic technique for quantitative analysis of various pharmaceutical drugs and in clinical diagnosis.12,14,17 From these probes, the QDs have emerged recently due to their extraordinary luminescence emission characters and have been utilized for the determination of pharmaceuticals and biologically active substances.14–18 Therefore, the present method was designed for the preparation of highly sensitive TGA-CdS QDs that produce maximum fluorescence intensity at 490 nm after excitation at 350 nm as represented in Fig. 1a.
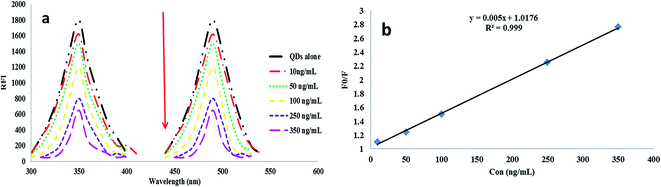 |
| Fig. 1 (a) Fluorescence spectra of TGA-CdS QDs in the absence and presence of different concentrations of DOR (10, 50, 100, 250 and 350 ng mL−1); excitation/emission = 350/490 and (b) Stern–Volmer relationship for fluorescence quenching of the TGA-CdS QDs with different concentrations of DOR (10–500 ng mL−1). | |
After the addition of varying concentrations of DOR (10, 50, 100, 250 and 350 ng mL−1) to the QDs, a quenching in the fluorescence intensity of the TGA-CdS QDs was observed as shown from Fig. 1a.
The mechanism of reaction was explained using the Stern–Volmer equation:15
where,
F0 and
F are the fluorescence efficiencies of the QDs in the absence and presence of the quencher (DOR), respectively,
Ksv is the Stern–Volmer quenching constant and [
Q] is the quencher concentration.
The linearity of the Stern–Volmer plot is a clear indication of the dynamic quenching mechanism. DOR interacts with the excited TGA-CdS QDs resulting in energy/electron transfer, which led to the quenching of the fluorescence of the quantum dots. This process is exactly described by the Stern–Volmer model.
The Stern–Volmer plot was found to be linear (R2 = 0.999) in the concentration range of 10–500 ng mL−1, and the results represented in Fig. 1b are compatible with the formation of dynamic quenching between the TGA-CdS QDs and DOR.
However, the quantum yield of TGA-CdS QDs was calculated via a comparative method16 using rhodamine B (QY = 0.31) solution as reference standard from the following equation:
where
Qst is the fluorescence quantum yield for rhodamine B,
I is the integrated fluorescence intensity,
η is the solvent refractive index (distilled water) and
A is the absorption; which was constantly kept under 0.1 to avert the reabsorption effect on the inward emission spectrum.
19 The quantum yield of the TGA-CdS QDs was calculated to be 0.32 after reaction with DOR.
3.2. Characterizations of the TGA-CdS QDs
The average size of the synthesized TGA-CdS QDs was verified using the transmission electron microscope (TEM); the particles were quite separated from each other in a mono-dispersing form and having diameter of 8.6–9.1 nm range with an average size of 8.85 nm, as shown in Fig. 2a.
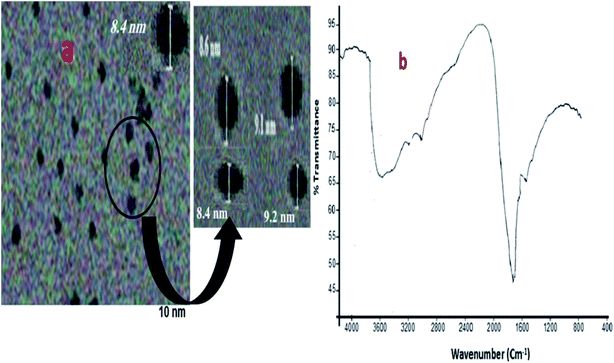 |
| Fig. 2 (a) TEM image and (b) FTIR spectrum of the synthesized TGA-CdS QDs. | |
Furthermore, the particle size was calculated by the Debye–Scherrer20 equation:
where
k is a constant,
λ is the wavelength of X-ray,
β is the full-width at half-maximum and
Θ is the Bragg's angle, it was found that the size was equal to 8.85 nm, which agrees with the TEM result. For more confirmation of the structure of TGA-CdS QDs, FT-IR spectra were obtained. Diagnostic peaks appeared in the spectrum at 3550 and 1660 cm
−1 corresponding to the –OH and C
![[double bond, length as m-dash]](https://www.rsc.org/images/entities/char_e001.gif)
O groups specific for the thioglycolic acid
17as shown in
Fig. 2b.
Furthermore, the diffraction peaks in XRD pattern were centered around 26.95°, 44.5° and 52°, which correspond to the diagnostic peaks of the CdS quantum dots,21 as shown in Fig. 3a.
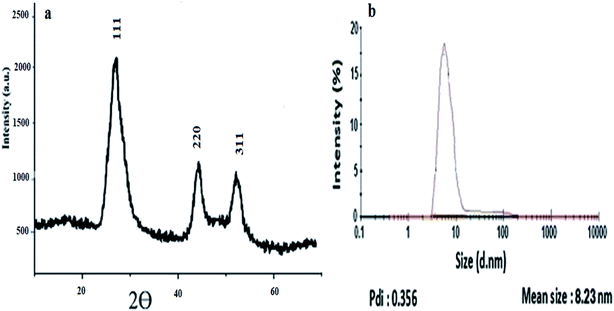 |
| Fig. 3 (a) XRD spectrum and (b) Zeta sizer curve of the synthesized TGA-CdS QDs. | |
For further confirmation, the Zeta sizer data reveals that the TGA-CdS QDs are mono-dispersed with an average hydrodynamic diameter of 8.82 nm and pdi = 0.356; the results were in agreement with the TEM results as represented in Fig. 3b.
3.3. Optimization of the experimental parameters
The effect of temperature on the fluorescence intensity of the TGA-CdS QDs and on their stability was measured in the range of 25–60 °C. It was observed that by increasing the temperature higher than room temperature, a measurable decrease of the QDs fluorescence intensity was occurred as represented in Fig. 4a. Hence, all the fluorescence measurements were performed at room temperature.
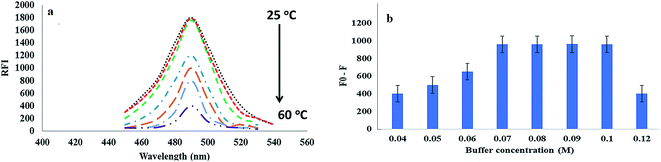 |
| Fig. 4 (a) The effect of different temperatures on the fluorescence intensity of TGA-CdS QDs and (b) the effect of concentration of Tris–HCl buffer on the quenching of TGA-CdS QDs in presence of DOR (250 ng mL−1); where F0 and F are the fluorescence intensity of QDs in the absence and presence of DOR respectively. | |
Further, the stability of TGA-CdS QDs and its reaction with the cited drug (DOR) is pH-dependent. So, different buffer systems such as Tris–HCl, Teorell and Stenhagen, borate and phosphate buffer solutions were tested. It was observed that the fluorescence quenching efficiency (represented as F0/F, where F0 and F are the fluorescence intensities of the TGA-CdS QDs in the absence and presence of DOR at 490 nm, respectively) increased using Tris–HCl buffer compared with the other buffer systems. Moreover, different concentrations of Tris–HCl buffer solutions were investigated in the range 0.05–0.15 M, and it was noticed that the highest efficiencies for the fluorescence quenching were obtained from 0.07 to 0.10 M. Hence, 0.08 M of Tris–HCl buffer was selected as shown in Fig. 4b. After that, different pH values in the range of 6.6 to 7.8 were examined to select the optimum pH value. It was observed that the buffer solution at pH 7.2 showed the most stable fluorescence quenching efficiency and additional increase in the pH values, higher than 7.6 led to a gradual decrease as shown in the corresponding results of Fig. 5a. Furthermore, 2.0 mL of 0.08 M Tris–HCl buffer at pH 7.2 was enough for the stabilization of the synthesized TGA-CdS QDs and its reaction with DOR.
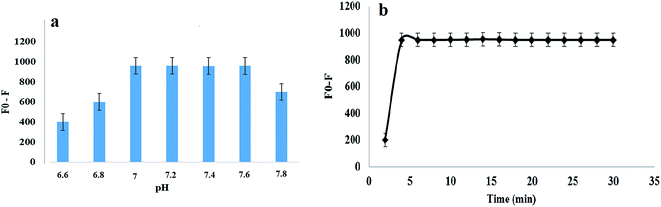 |
| Fig. 5 The effects of: (a) pH value of Tris–HCl buffer and (b) reaction time on the quenching of TGA-CdS QDs in presence of DOR (250 ng mL−1); where F0 and F are the fluorescence intensity of QDs in the absence and presence of DOR respectively. | |
Moreover, the fluorescence quenching efficiency in accordance with the addition of DOR was studied at different time intervals from 1 to 30 min. The maximum fluorescence quenching of the TGA-CdS QDs was obtained within 5 min after the addition of DOR. After that, any further increase in time did not lead to any further increase in the quenching. Subsequently, 5 min was chosen as the optimal reaction time as shown in Fig. 5b.
Nevertheless, various diluting solvents like distilled water, methanol, ethanol, acetonitrile, and acetone were studied. Higher response was observed using distilled water; hence, it was selected as the optimum solvent producing stable quenching results as represented in SM1 (ESI†).
3.4. Method validation
3.4.1. Linearity range and sensitivity parameters. The validation study was performed according to the ICH guidelines22 and US-FDA recommendations.23 A calibration curve was prepared using a standard solution of DOR under the optimum experimental conditions. Good linearity within the range of 10–500 ng mL−1 was obtained with the determination coefficient of 0.9991 using the Stern–Volmer equation as represented by:
where, F0 and F are the fluorescence intensities of the TGA-CdS QDs in the absence and presence of DOR, respectively.The developed spectrofluorimetric method is considered as an ultra-sensitive one with the limit of detection (LOD) value reaching 2.00 ng mL−1 and the limit of quantitation (LOQ) value equal to 6.00 ng mL−1. All statistical parameters of the proposed method are summarized in Table 1.
Table 1 Analytical parameters for the spectrofluorimetric determination of DOR using TGA-CdS QDS
Parameter |
DOR |
LOD: limit of detection. LOQ: limit of quantitation. |
λex (nm) |
350 |
λem (nm) |
490 |
Concentration range (ng mL−1) |
10–500 |
Determination coefficient (r2) |
0.9991 |
Slope |
0.005 |
Intercept |
1.017 |
SD the intercept (Sa) |
0.003 |
LODa (ng mL−1) |
2.00 |
LOQb (ng mL−1) |
6.00 |
3.4.2. Precision and accuracy. Furthermore, different concentrations of DOR (20.0, 30.0, 50.0, 100.0, and 300.0 ng mL−1) were used to examine the accuracy of the proposed method. The accuracy results were ranged from 99.84% to 100.38% with RSD values ranged from 0.30 to 1.09, as shown in the data summarized in Table 2.
Table 2 Accuracy and precision results of the proposed method for determination of DOR using TGA-CdS QDs
Sample number |
Taken (ng mL−1) |
Found (ng mL−1) |
% recoverya ± RSD |
Average of three determinations. RSD: relative standard deviation. |
1 |
20.0 |
20.10 |
100.50 ± 1.03 |
2 |
30.0 |
30.06 |
100.20 ± 0.30 |
3 |
50.0 |
50.02 |
100.04 ± 0.84 |
4 |
100.0 |
99.84 |
99.84 ± 1.09 |
5 |
300.0 |
300.09 |
100.03 ± 0.91 |
Intra-day precision |
20 |
20.01 |
100.05 ± 0.43 |
40 |
40.39 |
100.99 ± 0.61 |
60 |
59.96 |
99.94 ± 0.89 |
Inter-day precision |
20 |
19.99 |
99.97 ± 0.55 |
40 |
39.98 |
99.96 ± 0.70 |
60 |
59.83 |
99.71 ± 0.32 |
To examine the repeatability of the proposed method, intra-and inter-day precision were studied using three different concentration levels of DOR (20.0, 40.0, and 60.0 ng mL−1). The relative standard deviation (RSD) values were ranged from 0.32 to 0.89 as described in Table 2, the results ensure good repeatability of the proposed method.
3.4.3. Robustness. Moreover, the robustness of the presented method was checked by applying minor changes on several experimental parameters and the results are recorded at Table 3. The observed results indicate that there is no significant difference after applying this minor change and ensures reliability and robustness of the method.
Table 3 Robustness study of the proposed method for determination DOR in its pure form
Variation |
% recoverya ± SD |
Mean of six determinations. |
Optimum condition |
100.77 ± 0.82 |
1-Effect of pH (Tris–HCl-buffer) |
Variation |
% recoverya ± SD |
7.1 |
99.87 ± 0.22 |
7.4 |
99.94 ± 0.93 |
2-Effect of buffer concentration (M) |
Variation |
% recoverya ± SD |
0.06 |
99.17 ± 0.52 |
0.09 |
99.88 ± 0.40 |
3-Volume of buffer (mL) |
Variation |
% recoverya ± SD |
1.75 |
99.92 ± 0.43 |
2.25 |
99.90 ± 0.60 |
4-Time of the reaction (min) |
Variation |
% recoverya ± SD |
2 |
99.74 ± 0.70 |
8 |
99.97 ± 0.44 |
Further, a bio-analytical validation study was tested for human plasma samples, where accuracy, precision, Incurred sample reanalysis (ISR) and stability of DOR in the human plasma were examined using the US-FDA guidelines.23 Different concentrations of DOR (10.0, 30.0, 50.0, 100.0, 300.0 and 500.0 ng mL−1) were utilized to study the accuracy and precision of the method in real human plasma. RSD results did not exceed 1.89 and 1.95 for the intra-day (n = 6) and inter-day (n = 30) precision, the results are summarized in SM2 (ESI†).
3.4.4. Selectivity of the proposed method. The sensitivity of the proposed method was sufficient to determine DOR in human plasma; however, some interferences from the complex biological matrix may occur.To evaluate the selectivity of the TGA-CdS QDs towards DOR detection, we investigated the fluorescence behaviors upon addition of several amino acids under the optimized conditions. As shown from SM3 (ESI†), an enhancement in the RFI of TGA-CdS QDs was observed; in addition a shift to longer wavelength was occurred after their reaction with the amino acids. The observed enhancement is probably due to the coupling reaction between the amino group of amino acid and the free carboxyl group of TGA present on the TGA-CdS QDs. The formed products were measured at 550 nm (λex 350), which are far away from the emission wavelength used for measuring DOR (λem 490 nm), thus proving the selectivity for determination of DOR without any interferences from the co-existing biological molecules.
3.4.5. Incurred sample reanalysis (ISR). ISR is very important parameter to evaluate the accuracy and precision of incurred sample in bio-analytical validations using US-FDA guidelines.23 It was calculated as (% of difference between the concentration obtained by incurred samples and initial samples divided by their mean) × 100. The difference values did not exceed 3.96% as recommended by the US-FDA and are shown in Table 4.
Table 4 Incurred sample reanalysis data of DOR using the proposed spectrofluorimetric method
Sample |
Initial concentration (% recoverya ± SD) |
Incurred concentration (% recoverya ± SD) |
% deviation |
Mean of six determinations. |
1 |
98.01 ± 1.60 |
95.41 ± 2.10 |
−2.60 |
2 |
97.55 ± 2.11 |
93.59 ± 2.07 |
−3.96 |
3 |
97.22 ± 2.04 |
94.05 ± 1.76 |
−3.17 |
3.4.6. Stability of DOR in the human plasma. Varying factors affecting the stability of DOR in the human plasma were studied as mentioned in Section 2. Moreover, the percent of recovery was calculated further after these various conditions and the results were ranging from 94.65% to 97.63%, as described in Table 5.
Table 5 Stability of DOR in human plasma under different conditions
Conditions |
% recovery ± SD (n = 5) |
Concentrations |
LQC (20 ng mL−1) |
MQC (100 ng mL−1) |
HQC (500 ng mL−1) |
Three freeze–thaw cycle stability (−24 °C) |
95.99 ± 1.28 |
95.77 ± 1.80 |
96.00 ± 0.84 |
Long-term stability (1 month at −24 °C) |
95.71 ± 1.50 |
96.51 ± 1.08 |
96.11 ± 0.92 |
Short-term stability (12 h at −24 °C) |
97.32 ± 1.39 |
97.00 ± 1.67 |
96.96 ± 1.87 |
Post-preparative stability (6 h at room temperature 25 °C) |
97.55 ± 1.06 |
97.63 ± 1.00 |
97.54 ± 1.32 |
Post-preparative stability (12 h at room temperature 25 °C) |
95.10 ± 1.42 |
94.65 ± 1.80 |
95.90 ± 1.55 |
The highest stability results were obtained from the post-preparative stability (6 h at room temperature 25 °C), and the recovery results were in the range of 97.54 ± 1.32 to 97.63 ± 1.00.
However, the post-preparative stability (12 h at room temperature 25 °C) was the lowest value, and the recovery results were in the range of 94.65 ± 1.80 to 95.90 ± 1.55.
3.5. Application of the proposed method
3.5.1. Determination of DOR in pharmaceutical vial. DOR is used for managing different bacterial infections; this medication is known as a carbapenem-type antibiotic. The developed spectrofluorimetric method was utilized for the estimation of DOR in its pharmaceutical vial Doripax 500®. The % recovery result was found to be 101.96 ± 0.24 compared with the recovery result of the reported method (99.80 ± 1.23).5 The t-value and F-value of the proposed method when compared with the previously reported one5 were found to be 1.72 and 2.11, respectively.
3.5.2. Determination of DOR in biological fluids. Owing to the high sensitivity of the proposed method, it was successfully applied for estimation of the studied drug in human plasma and urine. DOR was determined in the human plasma, where the % recovery results were found to be in the range of 95.16% ± 1.21% to 97.62% ± 1.64% using the investigated method as described in Table 6.
Table 6 Application of the proposed method for determination of DOR in human plasma and human urine
Spiked human plasma |
Spiked human urine |
Added conc. (ng mL−1) |
Found conc. (ng mL−1) |
% recoverya ± SD |
Found conc. (ng mL−1) |
% recoverya ± SD |
Average of six determinations. |
10 |
9.71 |
97.10 ± 0.84 |
9.94 |
99.40 ± 0.53 |
30 |
28.55 |
95.16 ± 1.21 |
29.85 |
99.46 ± 0.68 |
50 |
48.81 |
97.62 ± 1.64 |
49.50 |
99.00 ± 1.02 |
100 |
97.10 |
97.10 ± 0.79 |
99.51 |
99.51 ± 0.47 |
300 |
290.55 |
96.85 ± 1.53 |
297.76 |
99.25 ± 2.05 |
350 |
340.27 |
97.22 ± 1.60 |
348.09 |
99.45 ± 1.46 |
The % recovery results of DOR in spiked human urine samples were ranged from 99.00% ± 1.02% to 99.51% ± 0.47% and without any interference from the complex matrix as presented in Table 6.
3.6. Pharmacokinetic study
The pharmacokinetics of DOR was studied using single infusion dose (500 mg) and the dose was collected after intervals. The study involved 10 volunteers aged from 23 to 37 years. Blood samples were collected after certain intervals (0.25, 0.5, 1.0, 1.5, 2.0, 2.5, 3.0, 3.5, 4.0, 4.5, 5.0, 5.5, 6.0 h). After pre-treatment of the plasma samples, the maximum concentration of DOR (Cmax) was observed to be 32.03 ± 2.32. The obtained results agreed with the previously reported one.6 All the results were observed and are shown in Table 7.
Table 7 Pharmacokinetic study of DOR using the proposed method
Time (h) |
IV (μg mL−1) |
Parameters |
Results |
0.25 |
10.22 ± 3.55 |
Cmax (μg mL−1) |
32.03 ± 2.32 |
0.5 |
32.03 ± 2.32 |
Tmax (h) |
0.30 ± 0.02 |
1.0 |
16.95 ± 2.0 |
t1/2 (h) |
1.12 ± 3.61 |
1.5 |
13.80 ± 2.21 |
AUC (μg h mL−1) |
74.34 ± 6.22 |
2.0 |
12.59 ± 1.55 |
|
|
2.5 |
10.33 ± 1.11 |
|
|
3.0 |
9.43 ± 1.03 |
|
|
3.5 |
7.84 ± 0.90 |
|
|
4.0 |
6.70 ± 0.89 |
|
|
4.5 |
5.88 ± 0.65 |
|
|
5.0 |
5.01 ± 0.51 |
|
|
5.5 |
4.60 ± 0.40 |
|
|
6.0 |
3.04 ± 0.33 |
|
|
The presented approach provides an ultra-sensitive fluorescence nano-probe for the estimation of DOR in real human plasma with LOD value reaching 2.0 ng mL−1 compared to the other reported methods.2–5,7–9
4. Conclusion
Thioglycolic acid capped cadmium sulphide quantum dots (TGA-CdS QDs) was synthesized as a fluorescence probe. The probe was utilized for the estimation of DOR in its pure form, pharmaceutical vial, human plasma and human urine. Different experimental parameters affecting the synthesis of TGA-CdS QDs and their interaction with DOR were examined. The proposed method showed a linear response within the concentration range of 10–500 ng mL−1 with a good correlation coefficient. The detection limit of the developed method reached 2.0 ng mL−1, which was sufficient for the detection of DOR in different biological fluids. Moreover, the presented method was utilized to study different pharmacokinetic parameters of DOR for additional clinical studies.
Conflicts of interest
There are no conflicts to declare.
References
- S. C. Sweetman, Martindale: the complete drug reference, Royal Pharmaceutical Society of Great Britain, Pharmaceutical Press, London, 36th edn, 2009 Search PubMed.
- J. C. Piontek and A. Jelinska, Spectrochim. Acta, Part A, 2010, 77, 554–557, DOI:10.1016/j.saa.2010.06.019.
- K. R. Babu and N. A. Kumari, Der Pharma Chemica, 2013, 5, 312–316 Search PubMed.
- K. R. Babu, N. A. Kumari and R. Vijaya Lakshmi, Int. J. Pharm. Sci. Drug Res., 2013, 5, 184–186 CAS.
- K. R. babu, N. A. Kumari and R. VijayaLakshmi, Int. J. Adv. Pharm., Biol. Chem., 2014, 3, 151–158 CAS.
- B. I. Salman, S. A. Hussein, M. F. B. Ali and M. A. Marzouq, Microchem. J., 2019, 145, 959–965, DOI:10.1016/j.microc.2018.12.018.
- F. S. Barbosa, J. P. E. Cassol, L. C. Batista, E. W. F. Cordeiro, M. C. Santos, A. R. Pohlmann, E. E. S. Schapoval, C. V. Garcia and A. S. Loureiro Mendez, Biomed. Chromatogr., 2017, 31, 1–10, DOI:10.1002/bmc.3940.
- P. Jayasekhar and J. Kurien, Int. J. Pharm. Sci. Rev. Res., 2013, 22, 41–45 Search PubMed.
- P. R. M. Reddy, S. Prathyusha, P. Shanmugasundaram, P. Y. Naidu, G. V. Hanumanaraju and R. Karthikeyan, J. Liq. Chromatogr. Relat. Technol., 2014, 37, 298–310, DOI:10.1080/10826076.2012.745136.
- J. Kurien, P. Jayasekhar and J. John, Int. J. Pharm. Sci. Rev. Res., 2014, 27, 317–321 CAS.
- N. H. Al-Shaalan, Am. J. Pharmacol. Toxicol., 2012, 7, 1–7 CrossRef CAS.
- M. F. B. Ali, M. A. Marzouq, B. I. Salman and S. A. Hussein, Microchem. J., 2019, 146, 187–191, DOI:10.1016/j.microc.2019.01.016.
- T. D. Chaemiso, Journal of Natural Sciences Research, 2017, 7, 6–15 Search PubMed.
- Q. Zhang, C. Zhang, Z. Li, J. Ge, C. Li, C. Dong and S. Shuang, RSC Adv., 2015, 5, 95054–95060, 10.1039/C5RA18176C.
- S. Kulchat, W. Boonta, A. Todee, P. Sianglam and W. Ngeontae, Spectrochim. Acta, Part A, 2018, 196, 7–15, DOI:10.1016/j.saa.2018.01.062.
- Q. Sun, Y. Long, H. Li, S. Pan, J. Yang, S. Liu and X. Hu, J. Fluoresc., 2018, 28, 523–531, DOI:10.1007/s10895-018-2213-8.
- L. Wang, L. Wang, C. Zhu, X. W. We and X. Kan, Anal. Chim. Acta, 2002, 468, 35–41, DOI:10.1016/S0003-2670(02)00632-3.
- J. M. Costa-Fernández, R. Pereiro and A. Sanz-Medel, TrAC, Trends Anal. Chem., 2006, 25, 207 CrossRef.
- C. Würth, M. Grabolle, J. Pauli, M. Spieles and U. R. Genger, Nature Protocols, 2013, 8, 1535–1550, DOI:10.1038/nprot.2013.087.
- C. Tyagi, A. Sharma and R. Kurchania, Journal of Non-Oxide Glasses, 2014, 6, 23–26 Search PubMed.
- W. W. Yu, L. Qu, W. Guo and X. Peng, Chem. Mater., 2003, 15, 2854–2860 CrossRef CAS.
- International Conference on Harmonization Topic Q2 (R1) Validation of Analytical Procedures Text and Methodology 2005 Search PubMed.
- D. Zimmer, Bioanalysis, 2014, 6, 13–19, DOI:10.4155/bio.13.298.
Footnote |
† Electronic supplementary information (ESI) available. See DOI: 10.1039/d0ra07960j |
|
This journal is © The Royal Society of Chemistry 2020 |
Click here to see how this site uses Cookies. View our privacy policy here.