DOI:
10.1039/D0RA05962E
(Paper)
RSC Adv., 2020,
10, 30162-30168
A simple Fe3+/bisulfite system for rapid degradation of sulfamethoxazole
Received
8th July 2020
, Accepted 10th August 2020
First published on 17th August 2020
Abstract
Sulfate radical (SO4˙−) based oxidation technologies have been widely used in the remediation of antibiotic-containing wastewater. Activated persulfates are efficient reagents for achieving SO4˙−, but the storage and transportation of concentrated persulfates present associated safety issues. In this study, bisulfite (BS) was used as an alternative precursor for replacing persulfates, and a simple advanced oxidation system (Fe3+/BS) for generating SO4˙− and hydroxyl radical (HO˙) was formulated and evaluated for removing sulfamethoxazole (SMX) from contaminated water. The initial pH, dosages of Fe3+ and BS, as well as the water matrix were investigated to improve the SMX degradation. The results indicated that 1 μmol L−1 SMX was completely removed within 5 min at optimum initial pH of 4.0, Fe3+ dosage of 10 μmol L−1, BS dosage of 100 μmol L−1 and temperature of 25 °C. The presence of HCO3− and natural organic matter (NOM) showed obviously negative effects on SMX degradation, while Cu2+ could slightly promote the degradation of SMX if its concentration was in an appropriate range (∼1 μmol L−1). Scavenger quenching experiments confirmed the presence of SO4˙− and HO˙, which resulted in efficient SMX degradation in the Fe3+/BS system. During the radical chain reactions, Fe2+ and Fe3+ could be converted into each other to form self-circulation in this system. The degradation pathway of SMX by Fe3+/BS was proposed including hydroxylation and bond cleavage.
1 Introduction
Advanced oxidation processes (AOPs), a method mainly based on the reactive species generated from the decomposition of precursors such as hydrogen peroxide, peracetic acid and persulfate (S2O82−),1 have been widely used in wastewater remediation due to their applicability to a wide range of contaminants, high efficiency and cost effectiveness.2 Among all commonly used AOPs, sulfate radical (SO4˙−) based oxidation technologies have been paid more attention due to its high redox potential (E0 = 2.6–3.1 V) and strong selectivity.3 Even though SO4˙− can be effectively generated through the heat, UV and alkaline pH activation of persulfate, Fe(II) or Fe(III) based activation is a viable method for practical application due to its high radical yield and additional coagulation function for contaminant coprecipitation.4
Nowadays, some novel systems using bisulfite (BS) as an alternative precursor for replacing persulfate to generate SO4˙− have been developed to overcome the safety risks from transportation and storage processes of persulfate due to its chemical instability.5 Furthermore, several metal ions, including Fe2+, Fe3+, Co2+ and Cr6+, have been proved to be effective for catalyzing BS to generate SO4˙−.6 Among these catalysts, Fe3+ may be extensively used because it is more environmentally friendly than Co2+ or Cr6+ and more chemically stable than Fe2+. Fe3+ activated BS may have a lot of similarity to Fe2+/persulfate system. Once SO4˙− is generated, it can propagate a series of reactions involving the formation of other reactive species, particularly the hydroxyl radical (HO˙). The possible chain reactions in the presence of oxygen during above process are shown in eqn (1)–(15) and (Table 1).7–14 However, few works focused on the application of such system in the oxidation of organic or inorganic contaminants.
Table 1 Possible reactions involved in the Fe3+/BS system
Reactions |
k (M−1 s−1) |
(1) Fe(III) + nHSO3− → FeIII(HSO3−)n (n = 1, 2, 3) |
|
(2) FeIII(HSO3−)n → Fe(II) + (n − 1)HSO3− + H+ + SO3˙− |
|
(3) Fe(III) + SO3˙− + H2O → Fe(II) + HSO4− + H+ |
|
(4) SO3˙− + O2 → SO5˙− |
k = (1.1–2.5) × 109 |
(5) SO5˙− + HSO3−→SO4˙− + SO42− + H+ |
k = 1.2 × 104 |
(6) SO4˙− + HSO3− → SO3˙− + SO42− + H+ |
k = 1.3 × 108–2.5 × 109 |
(7) 2SO5˙− → 2SO4˙− + O2 |
k = 104–108 |
(8) 2SO5˙− → S2O82− + O2 |
k = 107–108 |
(9) Fe(II) + SO4˙− → Fe(III) + SO42− |
k = 4.6 × 109 |
(10) Fe(II) + SO5˙− + H+ → Fe(III) + HSO5− |
k = 2.0 × 108 |
(11) Fe(II) + HSO5− → Fe(III) + SO4˙− + OH− |
k = 3.0 × 104 |
(12) SO4˙− + OH− → SO42− + HO˙ |
k = (1.4–6.5) × 107 |
(13) SO4˙− + H2O → SO42− + HO˙ + H+ |
k = 1.1 × 101 |
(14) HO˙ + HSO3− → SO3˙− + H2O |
k = 4.5 × 109 |
(15) Fe(II) + HO˙ → Fe(III) + OH− |
k = 4.3 × 108 |
Sulfamethoxazole (SMX), a synthetic antimicrobial that is extensively used in both human and veterinary medicine, has been frequently detected in many water bodies and effluents from wastewater treatment plants due to its incomplete removal during conventional wastewater treatment,15 which leads to an increase of antibiotic resistance in humans. Fe3+/BS based advanced oxidation process may be a suitable method for the removal of SMX from wastewater due to the generation of oxysulfur radicals. However, little is known about the mechanism of SMX oxidation by Fe3+/BS and the primary reactive species involved, although this system with or without light irradiation was widely used in the treatment of wastewater containing dyes or heavy metal ions.13,16,17 Furthermore, few works focused on the Fe(II)/Fe(III) self-circulation during the degradation process of contaminants in Fe3+/BS system.
Thus, the aim of this work was to propose the in situ simultaneous activation of BS by Fe3+ and degradation of SMX by radicals generated from Fe3+/BS. The effects of operational factors, such as the initial pH, Fe3+ and BS dosages, as well as the common water quality constituents including natural organic matter (NOM), inorganic anions and cations on the degradation of SMX were discussed. Besides, the dominant reactive species and Fe(II)/Fe(III) cycle in this system were investigated. Finally, the possible degradation pathways of SMX were tentatively proposed based on the detected reaction products.
2 Materials and methods
2.1 Materials
SMX, tert-butyl alcohol (TBA) and fulvic acid were produced by Aladdin Reagent Company, China. Ferric sulfate and sodium bisulfite were obtained from Sinopharm Chemical Reagent Corporation Ltd., China. Methanol and isopropanol (IPA) were the products of Fisher corporation. H2SO4, NaOH, NaCl, NaNO3, NaHCO3, Na2SO4, Na2S2O3, CuSO4, Mg(NO3)2, Ca(NO3)2 were all of analytical grade and purchased from Chengdu Kelong Chemical Reagent Co., Ltd, China. Deionized water was used in all experiments.
2.2 Experimental procedure
The SMX degradation experiments were carried out in a 250 mL glass beaker containing 100 mL SMX solution (1 μmol L−1) at 298 K. In each experiment, BS was added and the mixture was rotated for 10 min at 400 rpm. During this process, NaOH (0.5 M) and H2SO4 (0.5 M) were used to adjust the initial pH of solution. When the Fe3+ with specific amount was put into the SMX solution, the reaction between Fe3+ and BS resulted in the degradation of SMX. Samples (1.0 mL) were extracted at predetermined time intervals and filtered by 0.2 μm filter membrane, which were then rapidly quenched with 0.1 mol L−1 Na2S2O3. In the radical scavenging experiment, TBA and IPA were added into the pure SMX solution before BS addition.
2.3 Analysis
The SMX concentration was measured using Thermo' HPLC apparatus (Ultimate 3000) at 270 nm. The mobile phase was a mixture of methanol and 0.1% acetic acid (v/v = 60/40) at a flow rate of 0.8 mL min−1. The column temperature was set at 30 °C and the injection volume was 50 μL. The products during SMX degradation were analyzed by UPLC-Q-TOF-MS (ACQUITY UPLC, Quattro Premier XE, Waters, America) with a C18 reversed-phase column (100 × 2.1 mm, 1.7 μm). The mobile phase contained 0.1% formic acid water solution (A) and acetonitrile (B) at a flow rate of 0.5 mL min−1. The gradient was 90% A for 0.2 min, decreasing to 5% for 4.8 min, maintaining for 0.5 min, and then increasing back to 90% for 2 min. The solution pH was monitored by a pH meter (PHS-3C+, Leici). Phenanthroline colorimetric method was used for Fe2+/Fe3+ concentration measurement.
3 Results and discussion
3.1 Degradation mechanism of SMX by Fe3+/BS
3.1.1 Identification of reactive species in Fe3+/BS system. Fig. 1 displayed the complete removal of SMX by Fe3+/BS under proper conditions, and its degradation could be described by pseudo first-order kinetic model with the rate constant of 0.85 min−1. This result demonstrated that Fe3+/BS was an efficient system for SMX degradation and the radicals generated from Fe3+/BS might play an important role during this process. It is widely accepted that HO˙ and SO4˙− can be generated via the reaction of Fe(II) with peroxymonosulfate or persulfate and the transformation of reactive oxygen species,18 which might also occur in a Fe(III)/S(IV) system. Thus, the radical scavenging experiments were carried out to explore the dominant active radicals which were responsible for the removal of SMX by Fe3+/BS. TBA was selected as a strong HO˙ scavenger with the second-order rate constant of 3.8–7.6 × 108 M−1 s−1,17 while IPA was used as a nonselective scavenger for both HO˙ and SO4˙−, with the second-order rate constants of 1.9 × 109 M−1 s−1 and 8.2 × 107 M−1 s−1, respectively.19 As shown in Fig. 1, the SMX degradation rate constant in the system without TBA/IPA addition was much higher than those in Fe3+/BS/TBA (0.39 min−1) and Fe3+/BS/IPA systems (0.051 min−1). This result proved the participation of both HO˙ and SO4˙− in the SMX degradation process. Meanwhile, the SMX degradation rate in Fe3+/BS/TBA system was 7.6-fold faster than that in Fe3+/BS/IPA system, demonstrating that SO4˙− was the dominant radical in Fe3+/BS system under current conditions.
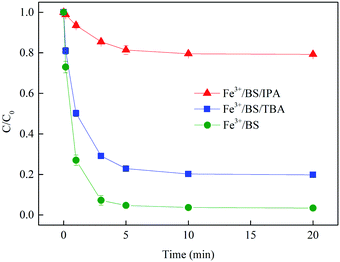 |
| Fig. 1 Effect of radical scavenger on SMX removal. Experimental conditions: [BS]0 = 100 μmol L−1, [Fe3+]0 = 10 μmol L−1, [SMX]0 = 1 μmol L−1, [IPA]0 = [TBA]0 = 1000 μmol L−1, pH0 = 4.0, T = 25 °C. | |
3.1.2 Variation in concentrations of Fe2+ and Fe3+ during SMX degradation. To clarify if Fe2+ and Fe3+ could be converted into each other in this system (as shown in eqn (1)–(3) and (9)–(11)), the concentration variation of Fe2+ and Fe3+ during SMX degradation was investigated. As shown in Fig. 2, the concentration of total Fe was maintained at about 10 μmol L−1 during the whole reaction. A decrease of Fe3+ concentration from 10 to 0.06 μmol L−1 was obviously observed in the initial 3 min, while Fe2+ concentration increased from 0 to 9.7 μmol L−1 correspondingly. However, 65.1% of Fe2+ was inversely transformed to Fe3+ after 20 min reaction, indicating that the oxidation of Fe2+ was occurred based on the eqn (9)–(11) and (15). This result proved the occurrence of Fe(II)/Fe(III) self-circulation in the Fe3+/BS system. For this reason, the radicals could be continuously generated during chain reactions between precursors and regenerated Fe(II)/Fe(III) species.
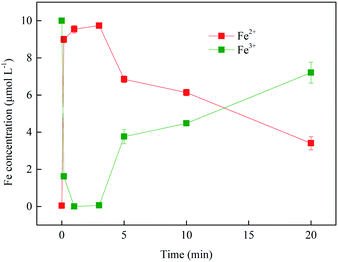 |
| Fig. 2 Variation in concentrations of Fe2+ and Fe3+ during SMX removal. Experimental conditions: [BS]0 = 100 μmol L−1, [Fe3+]0 = 10 μmol L−1, [SMX]0 = 1 μmol L−1, pH0 = 4.0, T = 25 °C. | |
3.2 Factors influencing SMX degradation by Fe3+/BS
3.2.1 Effect of initial pH. Free iron ions can activate BS to generate a series of oxysulfur radicals (e.g., SO3˙−, SO4˙− and SO5˙−), and the pH plays an important role in this process. To determine the optimum initial pH for SMX degradation in Fe3+/BS system, experiments at initial pH values between 3.0 and 8.5 were conducted. As shown in Fig. 4, the fastest and complete SMX removal was obtained at pH 4.0 and most of SMX was efficiently removed in the first 3 min. When the pH decreased to 3.0 or increased to 8.5, the SMX removal after 20 min reaction decreased to 37.0% and 2.9%, respectively. Speciation under the aforementioned conditions might primarily account for the increase in SMX degradation at pH 4.0. As reported by Wang et al.,6 the predominant S(IV) species at pH 4.0 is BS, and thus facilitating the formation of FeIII(HSO3−)n and the consequent reactions shown in eqn (1)–(15). Increasing pH might accelerate both the ionization of BS to form SO32− or S2O62− and the hydrolyzation of Fe(III), which inhibited the speciation of the system. pH 3.0 (or a lower pH value) had a negative effect on both radical generation and SMX removal, because part of S(IV) was volatilized at pH < 3.0. Consequently, 4.0 was deemed as the optimal pH and was used in subsequent experiments.
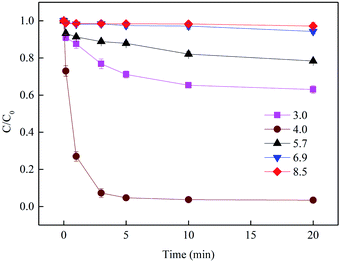 |
| Fig. 4 Effect of initial pH on SMX removal. Experimental conditions: [Fe3+]0 = 10 μmol L−1, [BS]0 = 100 μmol L−1, [SMX]0 = 1 μmol L−1, T = 25 °C. | |
3.2.2 Effect of BS dosage. Since BS was the only precursor of active radicals in a homogeneous Fe3+/BS system under acidic conditions, the dose of BS had a significant effect on the formation of reactive species and SMX degradation. As shown in Fig. 5, the SMX removal efficiency significantly increased with the increasing BS dosage from 10 to 100 μmol L−1, owing to the increasing generation rate and accumulation of FeIII(HSO3−)n and the subsequent radicals with the increasing BS concentration. However, excessively high BS concentration (such as >500 μmol L−1) could retard SMX degradation in the system because of the competitive reactions of excess BS with the active radicals according to eqn (5), (6) and (14). Hence, the balance between the reducing nature of BS and its activity to form radicals is critical. Considering the factors above and economic efficiency, 100 μmol L−1 was used as the optimum BS concentration for this system.
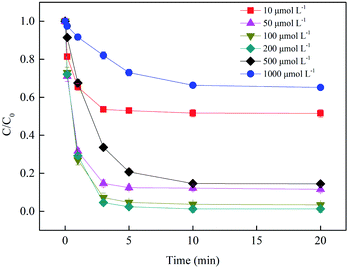 |
| Fig. 5 Effect of BS dosage on SMX removal. Experimental conditions: [SMX]0 = 1 μmol L−1, [Fe3+]0 = 10 μmol L−1, pH0 = 4.0, T = 25 °C. | |
3.2.3 Effect of Fe3+ dosage. Besides the solution pH and BS concentration, speciation of Fe(III) under the aforementioned conditions might primarily account for the increase in chain reaction rate, which was crucially influenced by initial Fe3+ dosage in the system. As shown in Fig. 6, a conspicuous enhancement in the SMX removal was observed when the Fe3+ dosage increased from 1 to 10 μmol L−1. Nevertheless, further increasing Fe3+ concentration only resulted in the acceleration of SMX degradation in the first 1 min, which indicated that 10 μmol L−1 Fe3+ was enough for activating 100 μmol L−1 BS. Therefore, 10 μmol L−1 of Fe3+ was chosen as the optimum condition in this study.
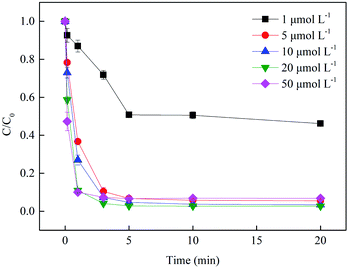 |
| Fig. 6 Effect of Fe3+ dosage on SMX removal. Experimental conditions: [BS]0 = 100 μmol L−1, [SMX]0 = 1 μmol L−1, pH0 = 4.0, T = 25 °C. | |
3.2.4 Effect of NOM. Natural organic matter can be both a source and a sink of radicals, the influence mechanism of which depends on the concentration and characteristics of dissolved organic compound.22 Carboxylate ligands are known to accelerate the advanced oxidation process by forming complex with Fe(III) which reacts faster with
than hydrate in the Fenton process, although they are always assumed as the scavengers for radicals, as shown in eqn (16) and (17).23 In order to investigate the effect of NOM on the Fe(III)/S(IV) system, fulvic acid (FA), a naturally existing macromolecular carboxyl-containing substance, was added in the system. As shown in Fig. 7, the SMX removal in 20 min decreased from 100% to 4.5% with the FA concentration increasing from 0 to 10 mg L−1, which indicated that the degradation of SMX was inhibited significantly in the presence of NOM and the radical scavenging effect of NOM surpassed its promoting effect on radical generation. |
HO˙ + NOM → products k = 2.23 × 108 L (mol C)−1 s−1
| (16) |
|
SO4˙− + NOM → products k > 6 × 106 L (mol C)−1 s−1
| (17) |
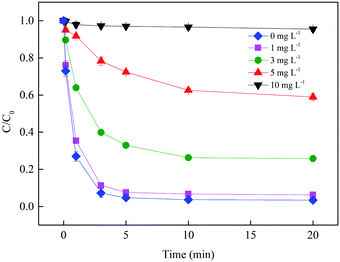 |
| Fig. 7 Effect of NOM on SMX removal. Experimental conditions: [BS]0 = 100 μmol L−1, [Fe3+]0 = 10 μmol L−1, [SMX]0 = 1 μmol L−1, pH0 = 4.0, T = 25 °C. | |
3.2.5 Effect of inorganic anions. SMX-contaminated waters usually contain not only SMX but also substantial concentrations of other inorganic ions (e.g., SO42−, NO3−, HCO3− and Cl−), which may affect the overall efficiency of Fe3+/BS system. In this study, these four typical background anions were selected for examination. As presented in Fig. 8, the presence of SO42− and NO3− both had little effect on SMX degradation, because they could hardly participate in the radical chain reactions. The HCO3− ion exerted an obvious inhibition effect on SMX degradation. After 20 min reaction, the removal of SMX in the presence of 0.5 mmol L−1 HCO3− fell to 7.5%. Possible explanations for this result include: (1) HCO3− could react with HO˙ and SO4˙− with the second-order rate constants of 3.9 × 108 M−1 s−1 and 9.1 × 106 M−1 s−1, respectively (as shown in eqn (18) and (19)), which was inefficient for SMX oxidation;23 and (2) the addition of HCO3− resulted in the increase of solution pH to alkaline range, which was unfavourable for the radical generation due to the formation of Fe(III) precipitate. For Cl−, as reported by Lutze et al.,24 SO4˙− can react with Cl− and the oxidation product Cl˙ is favoured at weakly acidic pHs. No obvious difference on SMX removal was observed between the Fe3+/BS system and Fe3+/BS/Cl− system, because the oxidation of SMX by Cl˙ and its derivate HO˙ (eqn (20)) might occur synchronously. |
HO˙ + HCO3− → CO3˙− + H2O
| (18) |
|
SO4˙− + HCO3− → CO3˙− + SO42− + H+
| (19) |
|
Cl˙ + H2O → HO˙ + Cl− + H+
| (20) |
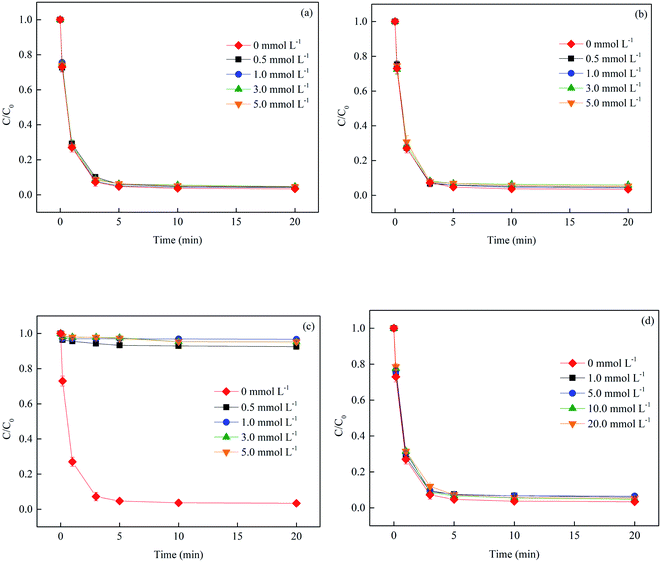 |
| Fig. 8 Effect of SO42− (a), NO3− (b), HCO3− (c) and Cl− (d) on SMX removal. Experimental conditions: [BS]0 = 100 μmol L−1, [Fe3+]0 = 10 μmol L−1, [SMX]0 = 1 μmol L−1, pH0 = 4.0, T = 25 °C. | |
3.2.6 Effect of metal cations. The cations in the solution might have different impacts on SMX removal by binding with it to change its chemical properties or reacting with precursor of radicals directly.25 In this study, the influence of three common cations (i.e., Cu2+, Ca2+ and Mg2+) on SMX degradation was evaluated. As shown in Fig. 9, the degradation of SMX was slightly promoted with the increase of Cu2+ concentration from 0 to 1 μmol L−1. 73.1% SMX was degraded in 1 min reaction without Cu2+ addition, while 76.9% SMX was removed when the Cu2+ concentration was 1 μmol L−1. A reasonable explanation for this result was that SO4˙− could be formed extra from chemically catalyzed decomposition of S2O82− (generated from eqn (8)) by Cu2+, as described in eqn (21), which had been reported by many researches.26 However, Cu2+ was also a scavenger for SO4˙− (eqn (22)), and thus excessive Cu2+ in the system was disadvantageous for SMX degradation. The presence of Ca2+ and Mg2+ could hardly affect SMX removal even at high concentrations, indicating that Ca2+ and Mg2+ were stable so as not to participate in the radical reactions and the sensitivity of SMX after complexation with Ca2+/Mg2+ was unchanged toward active radicals. |
Cu2+ + S2O82− → SO4˙− + SO42− + Cu3+
| (21) |
|
Cu2+ + SO4˙− → SO42− + Cu3+
| (22) |
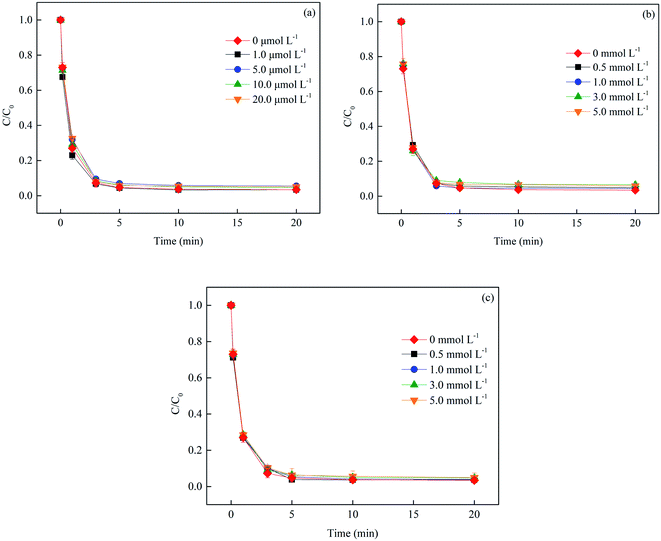 |
| Fig. 9 Effect of Cu2+ (a), Ca2+ (b), and Mg2+ (c) on SMX removal. Experimental conditions: [BS]0 = 100 μmol L−1, [Fe3+]0 = 10 μmol L−1, [SMX]0 = 1 μmol L−1, pH0 = 4.0, T = 25 °C. | |
4 Conclusion
Fe3+/BS, a simple homogeneous advanced oxidation system that efficiently generates multiple radicals was developed. The influence of operational parameters on SMX oxidation by Fe3+/BS, the dominating reactive species contributing to SMX degradation and the reaction pathways were investigated. Under the conditions of pH of 4.0, Fe3+ dosage of 10 μmol L−1, BS dosage of 100 μmol L−1, initial SMX concentration of 1 μmol L−1 and temperature of 25 °C, the removal of SMX was about 100% in 5 min reaction. The presence of SO42−, NO3−, Cl−, Ca2+ and Mg2+ showed little influence on SMX removal, while obviously negative effects of HCO3− and NOM were observed. Cu2+ could slightly promote the destruction of SMX if its concentration was in an appropriate range (∼1 μmol L−1). It has to be mentioned that both HO˙ and SO4˙− were the contributing reactive species for SMX degradation. Fe2+ and Fe3+ could be converted into each other to form a self-circulation in this system. According to the identified products, two degradation pathways of SMX including hydroxylation and bond cleavage were proposed.
Conflicts of interest
There are no conflicts to declare.
Acknowledgements
This research was financially supported by Sichuan Science and Technology Programs (2017SZ0175 and 2018SZDZX0026). Yiqing Liu also gratefully appreciates financial support from the Fundamental Research Funds for the Central Universities (2682018CX32).
References
- G. P. Anipsitakis and D. D. Dionysiou, Environ. Sci. Technol., 2003, 37, 4790–4797 CrossRef CAS PubMed.
- R. Yin, W. Guo, H. Wang, J. Du, Q. Wu, J. Chang and N. Ren, Chem. Eng. J., 2019, 357, 589–599 CrossRef CAS.
- J. Sharma, I. M. Mishra, D. D. Dionysios and V. Kumar, Chem. Eng. J., 2015, 276, 193–204 CrossRef CAS.
- A. Rastogi, S. R. Al-Abed and D. D. Dionysiou, Appl. Catal., B, 2009, 85, 171–179 CrossRef CAS.
- D. Zhou, L. Chen, J. Li and F. Wu, Chem. Eng. J., 2018, 346, 726–738 CrossRef CAS.
- H. Wang, S. Wang, Y. Liu, Y. Fu, P. Wu and G. Zhou, Chemosphere, 2019, 237, 124518 CrossRef CAS PubMed.
- C. Brandt, I. Fabian and R. V. Eldik, Inorg. Chem., 1994, 33, 687–701 CrossRef CAS.
- D. T. F. Kuo, D. W. Kirk and C. Q. Jia, J. Sulfur Chem., 2006, 27, 461–530 CrossRef CAS.
- P. Warneck and J. Ziajka, Ber. Bunsen-Ges. Phys. Chem., 1995, 99, 59–65 CrossRef CAS.
- R. E. Huie and P. Neta, Atmos. Environ., 1987, 21, 1743–1747 CrossRef CAS.
- M. Fischer and P. Warneck, J. Phys. Chem., 1996, 100, 15111–15117 CrossRef CAS.
- H. Herrmann, A. Reese and R. Zellner, J. Mol. Struct., 1995, 348, 183–186 CrossRef CAS.
- J. Xu, W. Ding, F. Wu, G. Mailhot, D. Zhou and K. Hanna, Appl. Catal., B, 2016, 186, 56–61 CrossRef CAS.
- F. J. Rivas, F. J. Beltran, J. Frades and P. Buxeda, Water Res., 2001, 35, 387–396 CrossRef CAS PubMed.
- M. N. Abella'n, B. Bayarri, J. Gime'nez and J. Costa, Appl. Catal., B, 2007, 74, 233–241 CrossRef.
- D. Zhou, Y. Yuan, S. Yang, H. Gao and L. Chen, J. Sulfur Chem., 2015, 36, 373–384 CrossRef CAS.
- Y. Yu, S. Li, X. Peng, S. Yang, Y. Zhu, L. Chen, F. Wu and G. Mailhot, Environ. Chem. Lett., 2016, 14, 527–532 CrossRef CAS.
- X. Xu, D. Liu, W. Chen, S. Zong and Y. Liu, Environ. Chem. Lett., 2018, 16, 1435–1440 CrossRef CAS.
- M. Gu, Q. Sui, U. Farooq, X. Zhang, Z. Qiu and S. Lyu, Chem. Eng. J., 2018, 354, 541–552 CrossRef CAS.
- J. Du, W. Guo, H. Wang, R. Yin, H. Zheng, X. Feng, D. Che and N. Ren, Water Res., 2018, 138, 323–332 CrossRef CAS PubMed.
- C. Qi, G. Yu, J. Huang, B. Wang, Y. Wang and S. Deng, Chem. Eng. J., 2018, 353, 490–498 CrossRef CAS.
- D. Vione, F. Merlo, V. Maurino and C. Minero, Environ. Chem. Lett., 2004, 2, 129–133 CrossRef CAS.
- B. M. Voelker and B. Sulzberger, Environ. Sci. Technol., 1996, 30, 1106–1114 CrossRef CAS.
- H. V. Lutze, N. Kerlin and T. C. Schmidt, Water Res., 2015, 72, 349–360 CrossRef CAS PubMed.
- Y. Liu, X. He, Y. Fu and D. D. Dionysiou, Chem. Eng. J., 2016, 284, 1317–1327 CrossRef CAS.
- X. Xu, Q. Ye, T. Tang and D. Wang, J. Hazard. Mater., 2008, 158, 410–416 CrossRef CAS PubMed.
|
This journal is © The Royal Society of Chemistry 2020 |