DOI:
10.1039/D0RA04852F
(Paper)
RSC Adv., 2020,
10, 27377-27386
Facile photocatalytic reduction of carcinogenic Cr(VI) on Fe-doped copper sulfide nanostructures†
Received
1st June 2020
, Accepted 3rd July 2020
First published on 21st July 2020
Abstract
In this study, Fe-doped copper sulfide nanoparticles (NPs) were investigated for the solar-assisted reduction of CrVI ions in raw water. The Fe-doped NPs were synthesized by decomposing copper(II) N,N-diphenylmethylpiperazinecarbamodithioate via a facile single-step, one-pot solvothermal method in the presence of iron salt. The CrVI photoreduction data were fit to a pseudo-first-order kinetic model and a Langmuir model. The CuS/Cu2S NP reduction ability for CrVI increases with an increase in dopant percentage. The best catalyst (9% Fe-doped) was able to reduce CrVI (10−4 M K2Cr2O7) to CrIII in raw water using an initial amount of 10 mg in 6 min with a reduction efficiency of up to 100%. The photocatalytic activity was examined while varying five different parameters: sunlight, diffused light, change in pH, and changes in the concentration of the catalyst and the temperature. This new approach presents an active, simple, and cost-effective means for wastewater treatment.
1. Introduction
Currently, an innovative technology built on the photocatalysis of an aqueous suspension of nanoparticles has received substantial consideration. The photocatalysis has been used for the quick and effective removal of environmental contaminants.1 The solar light with an energy more than the semiconductor's band gap sequentially generates electron–hole pairs in the conduction and the valence bands (VB). These charge carriers (electron–hole pairs) are responsible for oxidizing or reducing pollutants in solution, provided that they have appropriate redox potentials. An innovating application of solar light-driven photocatalytic process involves the elimination of toxic metals, like chromium, lead and mercury, from industrial surplus water. The metals are reduced on the catalyst's surface and can be successfully removed from the resulting slurries by chemical or mechanical means2 or they can be converted to less harmful materials.3–6
Chromium, an important inorganic element, exists commonly as the CrVI and CrIII forms in both naturally and anthropogenically modified waters. The former is injurious to human health and is classified as both mutagenic and carcinogenic. It has been declared a primary pollutant by the EPA (US Environmental Protection Agency) owing to its high solubility, high toxicity and easy accumulation.7–9 The World Health Organization (WHO) has recommended 0.1 ppm as the permitted limit of CrVI in inland water and 0.05 ppm in drinking water. CrVI exists in water as different oxyanions as a function of pH, i.e., chromate (CrO42−), hydrogen chromate (HCrO41−) and dichromate (Cr2O72−). Hence, the conversion of lethal CrVI into ecofriendly CrIII can be an important remediation approach to evade the venomous effects of CrVI on human health. CrIII is less poisonous and considered as an indispensable micronutrient for human health.10
Several methods have been described for CrVI reduction, involving ion exchange, adsorption, membrane filtration, chemical precipitation, solvent extraction and electrocoagulation. Most of these techniques, however, are accompanied by problems, such as sludge disposal, formation of secondary pollutants, high capital cost, and high power depletion. These are the reasons why these techniques are not widely used. In contrast, photocatalysis is gaining attention as it is an economically inexpensive, viable and eco-friendly technique. Various reductants are being used for hexavalent chromium reduction, such as H2S, HCOOH, Fe(II)-bearing minerals and Fe(0).11 HCOOH is the most favorable for the reduction of CrVI among these reductants owing to its production of relatively environment friendly by-products, mild reaction conditions, and ready availability.
Copper sulfide (CuxS) is an eminent p-type semiconductor that has diverse uses in the fields of electronics, chemical sensing, photocatalysis, and batteries. In the last decade, it has attained great consideration in the area of catalysis because of its intriguing properties, high catalytic efficiency, nontoxicity, low price and tunable band gap (1.2–2.5 eV) in the visible region depending upon the stoichiometric composition from x = 1 to 2.12 These features make CuS possibly an ideal light-harvesting and charge-transport material in photocatalysis. However, the solar-driven electron transfer process is restricted by recombination that quenches the photocatalytic efficiency. The most efficient way to improve the photocatalytic activity is to dope the material with suitable transition metals. In our work, FeII is used as the dopant because it has a sufficiently positive reduction potential to draw electrons from CuS and thus prevent recombination of the charges. Moreover, the quantum-confinement effect in the semiconductor shifts the conduction band (CB) to a more negative value, thereby facilitating the shift of electrons from the catalyst's surface to the adsorbed reactant molecules.13
2. Experimental
2.1 Materials
In the following experiments, CuCl2·2H2O, K2Cr2O7, Pb(NO3)2 and FeCl2·2H2O were purchased from Merck, NaOH from Fluka, and N,N diphenylmethylpiperazine, DMF, CS2, LR grade formic acid and ethylenediamine were purchased from Sigma-Aldrich. The analytical quality chemicals and solvents were used as received.
2.2 Preparation of single source precursor
The dithiocarbamate ligand and the copper complex were prepared according to our previously reported procedures.14 An aqueous solution of CuCl2·2H2O was prepared by dissolving 0.160 g (0.938 mmol) of the salt in deionized water (30 mL). To this solution, an aqueous solution (30 mL) containing 0.5 g (1.83 mmol) of sodium 4-benzhydrylpiperazine-1-carbodithioate (Scheme 1) was added. After 3 h of vigorous stirring, the brown precipitates formed were filtered, washed several times with cold water, and dried. Yield: 0.373 g, 73.3%. Mp: 262–264 °C. FTIR (cm−1): 1489, νC–N; 968, νC–S; 360, νCu–S.
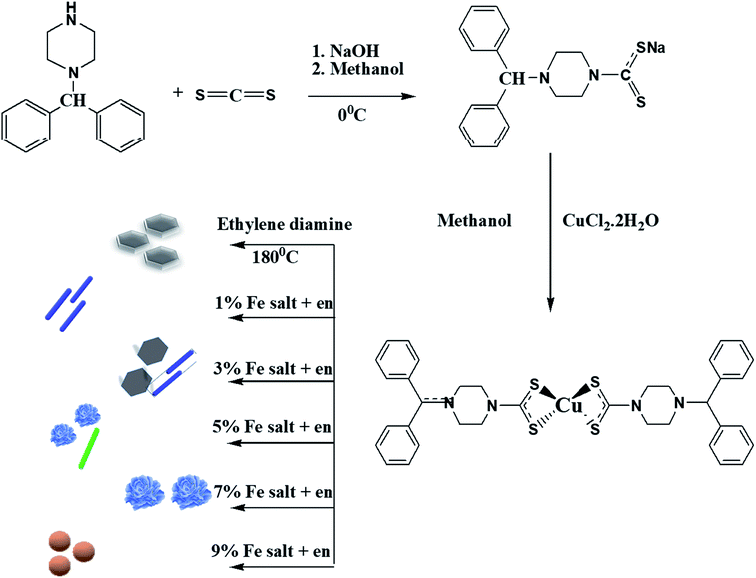 |
| Scheme 1 Synthesis of single source precursor, CuS/Cu2S and Fe-doped CuS/Cu2S. | |
2.3 Synthesis of CuS/Cu2S and Fe-doped CuS/Cu2S nanostructures
The CuS/Cu2S and Cu1−xFexS (x = 1, 3, 5, 7 and 9%) nanostructures were readily synthesized by a thermal decomposition procedure using a single source precursor (Scheme 1). About 1 g of the dried complex, together with x grams of FeCl2·2H2O (x = 0, 0.01, 0.03, 0.05, 0.07, 0.09 g), were added into a two-necked flask fitted with a condenser on one neck and a bent tube on the second that is connected to a test tube containing aqueous lead nitrate solution. About 15 mL of ethylenediamine was added and heated up to 180 °C for about 3 h. As the reaction progressed, the released H2S gas that was formed led to the production of PbS in the test tube containing the aq. Pb(NO3)2 solution. Eventually, black Fe-doped CuS/Cu2S nanoparticles were obtained. These nanoparticles were filtered off, washed thoroughly with methanol several times and dried at room temperature. The proposed mechanism for the synthesis of nanoparticles is shown in Scheme S1 in the ESI.†
2.4 Characterization
Scanning electron microscopy was carried out using a Schottky Field Emission JEOL JSM-7600F instrument operational at an accelerating voltage of 0.1 to 30 kV. An Energy Dispersive X-ray Analyzer (EDX) was used to confirm the purity of the material. Powder X-ray diffraction analysis was performed on a PANanalytical X'Pert3 PRO Powder instrument fitted with Cu Kα radiation (λ = 1.54178 Å). UV-vis analysis of the Fe-doped CuS/Cu2S nanoparticles was done on a Shimadzu double-beam spectrophotometer model 1800. A Nicolet 6700 Infrared Spectrophotometer (Thermo Scientific, USA) was used to measure the FTIR spectra of the complexes and the ligand. X-ray Photoelectron Spectroscopy (XPS) was done to confirm the oxidation states using a Thermo Scientific K-alpha Photoelectron Spectrometer via monochromatic Al Kα radiation. Peak positions were regulated using the C 1s peak at 284.8 eV via Casa XPSTM software. The intensity of the solar light was measured by a solar radiation meter.
2.5 Solar assisted photocatalysis
The photocatalytic performance of Cu1−xFexS was investigated under direct solar light with an average intensity of 17 mW cm−2. The CrVI reduction experiment, both in diffused and direct solar light, was conducted in a Pyrex glass of 100 mL capacity. The reduction reactions were examined by observing the changes in the UV-vis absorbance with the main absorption peak appearing at 352 nm. An aqueous solution of K2Cr2O7 was taken as a standard source of CrVI. About 1 mL of formic acid (23 g L−1) was added to 40 mL of an aqueous K2Cr2O7 solution (100 mg L−1) in a beaker followed by vigorous stirring at room temperature (25 °C) with the pH of the solution maintained at 2.5. At this point, the UV-visible spectrum of the solution mixture was recorded and was denoted as the 0 min data point. 0.01 g (10 mg/40 mL) of the synthesized Cu1−xFexS (x = 0, 1, 3, 5, 7, 9%) nanocatalysts were added to the solution under sunlight with constant stirring. After every 2 min interval, about 3 mL of the solution was collected into an UV-vis cuvette and spectroscopically examined.
3. Results and discussion
3.1 XRD analysis
Fig. 1 shows the crystallographic structures of the pristine CuS/Cu2S and Fe-doped CuS/Cu2S nanostructures, synthesized at 180 °C. The diffraction patterns with 2θ values positioned at 46.2° and 55.2° correspond to the (hkl) planes of the (110) and (201) of the hexagonal chalcocite (Cu2S) system {JCPDS no. 00-026-1116, space group P63/mmc (194)} while the peaks at 27.3° and 32.4° matched well with the (hkl) plane of (100) and (006) of covellite (CuS) {JCPDS card no. 06-0464, space group P63/mmc (194)}. No other phases were observed in the XRD spectra except for the hexagonal phase of CuS/Cu2S. The formation of Cu2S together with CuS is due to the reducing nature of ethylenediamine.15 Notable changes in the (100) and (110) diffraction peaks were observed. A reduction in the intensity of the (110) peak accompanied by an increase in the intensity of the (100) upon increasing the concentration of dopant indicated the key morphological changes in the material, most probably due to the lattice contractions. The broadening of the XRD peaks of the Fe-doped CuS/Cu2S NPs compared to those of pristine CuS shows the decrease in size of the nanoparticles with Fe dosage. The 2θ positions also shifted with an increase in Fe doping, which can be credited to the smaller ionic radii of Fe2+ (0.064 nm) compared to that of Cu2+ (0.065 nm). Furthermore, the average crystallite size (D) could be calculated using the FWHM (Full Width Half Maximum) of the diffraction peaks by using the Debye–Scherrer formula (eqn (1)), |
D = 0.9λ/B cos θ
| (1) |
where θ and λ are the diffraction angle and the wavelength of Cu Kα radiation (λ = 1.54178 Å) used as a source, respectively. The average crystallite sizes calculated for each dopant were found in the range of 10.51 to 21.96 nm.
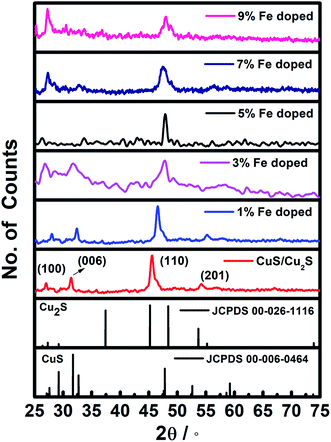 |
| Fig. 1 XRD pattern of CuS/Cu2S and (1, 3, 5, 7, 9%) Fe doped CuS/Cu2S NPs. | |
3.2 Optical analysis
UV-visible spectrophotometry is one of the most useful techniques to investigate the optical properties of nanoparticles. The absorption spectra of the synthesized nanoparticles lie in the visible region (Fig. 2), that makes them promising photocatalysts. For pristine CuS/Cu2S, the absorption edge was observed at 589 nm, i.e., there is a blue shift compared to bulk CuS (1032 nm).16 Likewise, with the addition of the dopant, there is a noticeable blue shift observed, indicating an enhancement in the band gap. This enhancement is due to sp-d exchange interactions among localized d-electrons of the dopant (Fe2+) and the CuS band electrons.17 Increases in absorption intensity after 600 nm up to the near IR region were observed owing to a surface plasmonic resonance (SPR), a distinctive characteristic of the covellite phase of CuS nanoparticles.18,19 The energy band gap “Eg” was calculated using Planck's equation, Eg = hc/λ where h is the Planck's constant, c is the speed of light and λ is the wavelength at which the maximum absorbance occurs.20 The calculated band gaps of the doped Cu1−xFexS (x = 0, 1, 3, 5, 7 and 9%) nanoparticles were 2.1, 2.27, 2.44, 2.45, 2.46 and 2.48 eV, respectively.
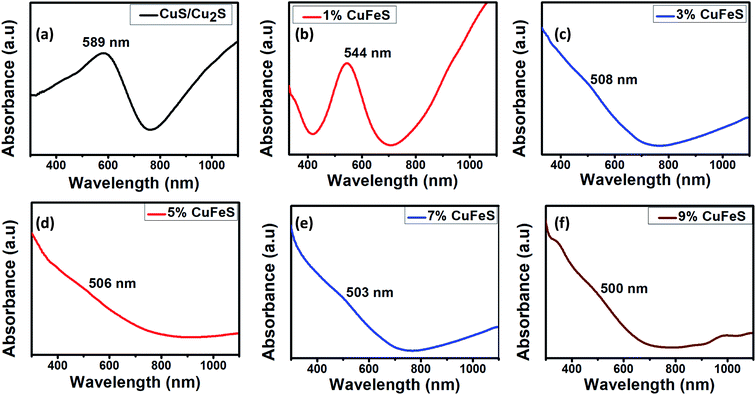 |
| Fig. 2 UV-vis absorption images of (a) pure CuS/Cu2S, (b) Fe (0.01)-CuS/Cu2S, (c) Fe (0.03)-CuS/Cu2S, (d) Fe (0.05)-CuS/Cu2S, (e) Fe (0.07)-CuS/Cu2S and (f) Fe (0.09)-CuS/Cu2S. | |
3.3 Structural characterization
Scanning electron microscopy is an excellent way of examining the morphologies of nanoparticles by monitoring the cross sections of samples. Fig. 3a–f illustrates the SEM images of the 0–9% Fe-doped CuS/Cu2S NPs. On the basis of these results, it can be assumed that the Fe has considerable control over the morphologies of the particles, which may be due to the significant reactions of iron under solvothermal conditions. The morphology of pristine CuS/Cu2S NPs is plate-like with a particle size around 1 μm. Once Fe is introduced, the morphology transforms completely as compared to that of the pristine copper sulfide nanoparticles. The increase in doping has clearly changed the morphology from plates to rods and then finally to flowers and spheres with a decrease in size.
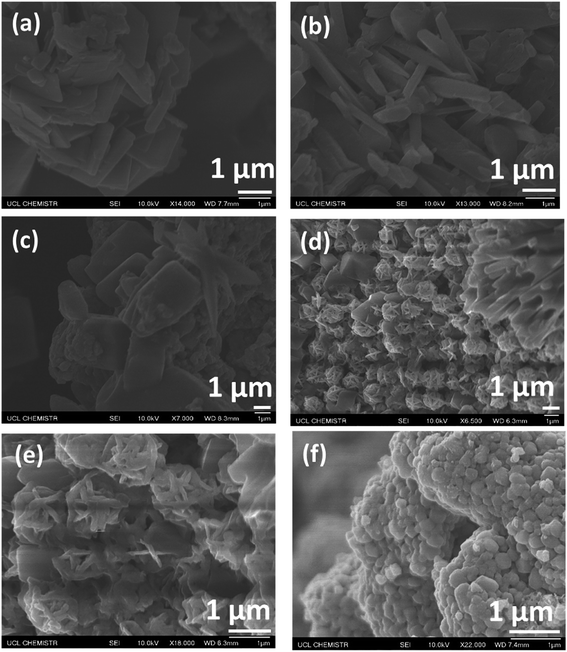 |
| Fig. 3 SEM images of (a) pure CuS/Cu2S, (b) Fe (0.01)-CuS/Cu2S, (c) Fe (0.03)-CuS/Cu2S, (d) Fe (0.05)-CuS/Cu2S, (e) Fe (0.07)-CuS/Cu2S and (f) Fe (0.09)-CuS/Cu2S. | |
3.4 Energy dispersive X-ray spectroscopy (EDX) analysis
The compositions of the Fe-doped CuS/Cu2S samples were studied using energy dispersive X-ray spectroscopy (EDX). Fig. S1 (ESI†) shows the EDX results for pure and the 1, 3, 5, 7, 9% Fe-doped CuS/Cu2S nanoparticles. The stoichiometric ratios of Cu, S and Fe from these data are as expected, thus indicating the absence of any other elements in the nanoparticles. There was a contribution from C and O from the sample mounting tape. Eliminating the percentages of C and O enabled us to establish the purity of the Fe doped CuS/Cu2S nanoparticles.
3.5 X-ray photoelectron spectroscopy (XPS)
High resolution X-ray photoelectron spectroscopy was employed to determine the chemical composition and valance states on the surface of the Fe doped CuS/Cu2S. Fig. 4a–c shows the core level spectra of Cu 2p, Fe 2p and S 2p. The core level spectra of Cu 2p (Fig. 4a) shows two major peaks at 934.5 eV and 954.5 eV due to the spin orbit splitting of Cu 2p into Cu 2p3/2 and Cu 2p1/2, respectively. In addition, the Cu 2p3/2 shows a small shoulder peak so it is further deconvoluted into two peaks located at 932.7 eV and 934.5 eV which correspond to the monovalent and divalent Cu species. The presence of satellite peaks (942.4 eV and 962.5 eV) confirms the existence of Cu2+ species. It is observed from the main and satellites peaks that the Cu2+ species are in a higher ratio than Cu+, which thus further confirms that the formation of CuS is more pronounced than that of Cu2S. Fig. 4b shows the Fe 2p core level spectra. The peaks for Fe 2p are well defined and broad due to the high concentration of doping (9%) and can further be deconvoluted. The peaks located at 710.8 eV and 723.8 eV are attributed to the Fe 2p3/2 and Fe 2p1/2, respectively. The satellite peaks located at higher binding energies (718.8 eV and 733.1 eV) indicate that the Fe ion is in a divalent state. The broad Fe 2p3/2 peak was deconvoluted into two peaks at 710.2 eV and 712.2 eV and could be assigned to divalent and trivalent Fe 2p3/2, respectively. The core level spectra of S 2p (Fig. 4c) is deconvoluted using a spin–orbit separation of 1.0 eV. The peaks at position 161.4 eV and 162.5 eV are merged into a single broad peak that appeared at 162.7 eV and can be assigned to the S 2p state of S2− ions, which verifies the coordination of S with Cu in CuS.21 Besides, a strong peak located at 168.3 eV can be assigned to the other species of S.
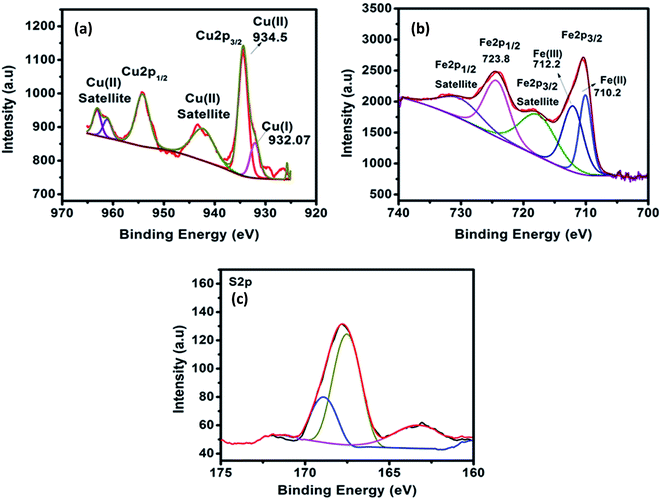 |
| Fig. 4 XPS spectra and fitted results of (a) Cu2p3/2, Cu2p1/2, (b) Fe 2p3/2, Fe 2p1/2 and (c) S 2p. | |
4. Photocatalytic reduction of CrVI
The photoreduction efficiencies of the undoped and Fe-doped (1, 3, 5, 7, 9%) CuS/Cu2S nanoparticles were assessed by studying the reduction of Cr(VI) (10−4 M K2Cr2O7) using a UV vis spectrophotometer under different experimental conditions. The reaction's progress was recorded by monitoring the behavior of the typical peak (352 nm) of CrVI over time. The effect of formic acid on the removal of CrVI was examined in the absence of the doped CuS/Cu2S NPs and no significant reduction occurred even after 24 h at room temperature. Similarly, in the absence of formic acid, the CrVI reduction proceeded slowly, indicating clearly that CrVI reduction could not be achieved using the catalyst alone (Fig. 5).
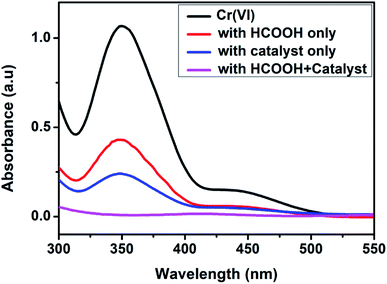 |
| Fig. 5 Behavior of the Cr(VI) peak in the UV-vis spectra in the presence and absence of the catalyst and HCOOH. | |
With the addition of catalyst together with formic acid, the absorption band at 352 nm diminished gradually and eventually a change in color from yellow to colorless, substantiated the CrVI reduction to CrIII.22–23 After reduction, the presence of trivalent chromium was demonstrated by the addition of excess NaOH. The formation of hexahydroxochromate(III) ions resulted in the appearance of a blue-green solution,23 which upon slight warming with the oxidant H2O2, resulted in a color change to yellow, confirming oxidation of the Cr(III) back to Cr(VI),24 as shown in Fig. 6.
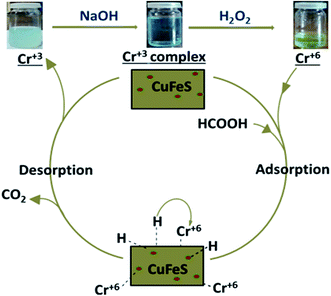 |
| Fig. 6 The color change of the Cr(VI) solution. | |
4.1 Photocatalytic activity in solar and diffused light
The photocatalytic performance of the synthesized nanoparticles in the CrVI to CrIII reductions was studied under sunlight and diffused light. About 90% of the CrVI was removed by pristine CuS/Cu2S under sunlight in 56 min, while only 62% was reduced under diffused light. It is worth noting that the CrVI conversion efficiency was considerably improved with an increase in the Fe doping in the copper sulfide NPs. All five doped Cu1−xFexS nanostructures (x = 1, 3, 5, 7 and 9%) showed a 100% reduction according to the UV-vis spectra in both sunlight and diffused light. With an increase in the percentage of dopant (Fe2+), a remarkable enhancement in the performance of the catalyst was observed. Among all the synthesized catalysts, the 9% doped sample showed the best performance. In sunlight, a complete reduction was observed in 6 min, while in diffused light the reduction took 9 min. Moreover, the photocatalytic performance of each of the five Cu1−xFexS nanostructures in diffused light was lower than that under sunlight (Fig. 7).
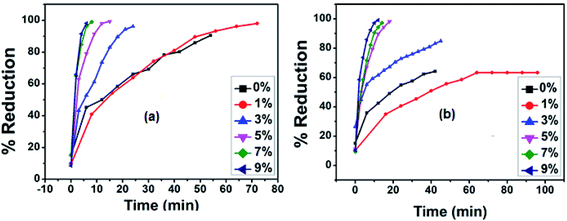 |
| Fig. 7 Plots for the concentration change of Cr(VI) with time for CuS/Cu2S and 1%, 3%, 5%, 7% and 9% Fe doped CuS/Cu2S NPs (a) in sunlight (b) in diffused light. | |
4.2 Effect of pH
The pH plays a key part in the photocatalysis as it effects the surface charge of the catalyst, thus directly affecting the adsorption, photoreduction and interfacial electron transfer processes.25 In our experiments, HCOOH functioned as a reductant and also performed an imperative role in regulating the pH of the reaction medium. The pH of the solutions was varied as 2.5, 3 and 4 by the addition of 1 M NaOH solution. A drastic decrease in the reduction rate was observed with an increase in pH, which agrees with the mechanism for CrVI reduction. The impact of pH on the photoreduction of CrVI was studied for 9% Fe-doped CuS nanoparticles with the fastest photoreduction occurring at pH 2.5. At low pH, there will be an excess of H+ ions that will boost the reduction rate.26 |
Cr2O72− + 8H+ + 3HCOOH → 2Cr3+ + 7H2O + 3CO2
| (2) |
At high pH, however, excess OH− ions become adsorbed on the catalyst surface and inhibit the dichromate oxyanions reaching the surface of the catalyst, resulting in a reduced rate of adsorption of the CrVI species and consequently a reduced CrVI reduction. Moreover, CrVI can be spontaneously reduced in acidic media due to its high redox potential, 1.33 eV. The mechanism for CrVI reduction involves the adsorption of oxyanions of Cr(VI), e.g., CrO42−, HCrO41− and Cr2O72−, by electrostatic attraction, since at a pH less than the point of zero charge (PZC), the CuS catalyst bears a positive charge. For CuS, a pH of 5.2 value is reported to be the PZC, which is why the surface of CuS bears a negative charge above pH 5.2 owing to the adsorbed OH− ions. At pH below 5.2 (under acidic conditions), the CuS catalyst has a positive charge.27
Thus, the efficacy of the catalyst for CrVI photoreduction is less at elevated pH values. Additionally, the poisoning of the photocatalyst may occur at a basic pH owing to the deposition of CrIII as Cr(OH)3 on the photocatalyst surface (Fig. 8).
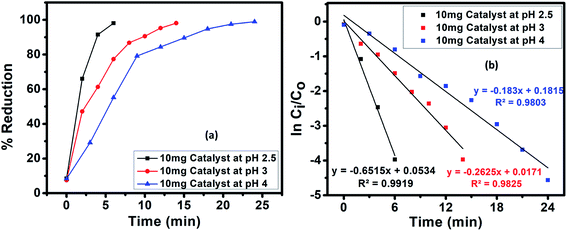 |
| Fig. 8 (a) Effect of pH on the solar-assisted catalytic reduction of Cr(VI) using an initial concentration of 100 mg L−1 of Cr(VI) and (b) the pseudo-first-order graph of ln(Ci/C0) versus time for the Cr(VI) photocatalytic reduction. | |
4.3 Effect of catalyst's amount
The catalyst amount is one of the key factors in enhancing the reduction conditions. In our experiments, the impact of the catalyst on the reduction of CrVI was examined using a catalyst dosage varying from 2.5 to 10 mg to evade an ineffective surplus amount of the catalyst (Fig. 9).
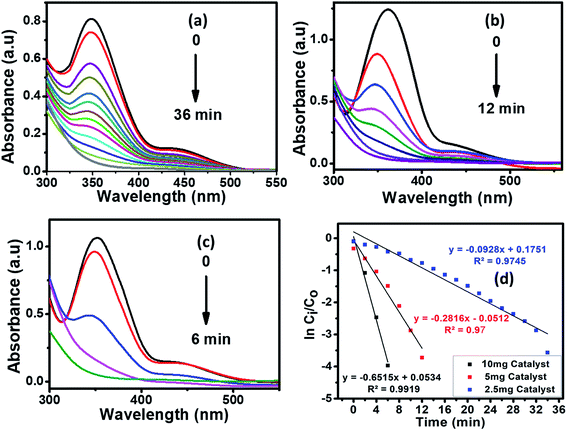 |
| Fig. 9 The photocatalytic reduction of aqueous Cr(VI) using HCOOH as the reducing agent, in the presence of various amounts (a) 2.5, (b) 5, (c) 10 mg of Cu1−xFexS as the catalyst (where x = 9%) and (d) the pseudo-first-order graph of ln(Ci/C0) versus time for the Cr(VI) photocatalytic reduction. | |
The results clearly indicated the gradual increase in the photocatalytic reduction of CrVI with the amount of catalyst. This situation may be attributed to the number of active sites on the surface of the photocatalyst and the penetration of the visible light through the solution. The kinetics of the CrVI reduction by Cu1−xFexS are illustrated in the Fig. 9 inset. A pseudo-first-order kinetic equation (eqn (3)) was applied to the hexavalent chromium reduction in the presence of an excess of formic acid.
|
ln Ci/C0 = −kt
| (3) |
where
k represents the apparent rate constant (min
−1),
Ci is the dichromate ion concentration at reaction time “
t” and
C0 is the initial concentration of the dichromate ion.
4.4 Effect of temperature
The influence of temperature on the CrVI reduction was studied by reacting 40 mL of 10−4 M CrVI with 10 mg Cu1−xFexS (x = 9%) nanoparticles and 1 mL of 4.99 × 102 mM HCOOH at 35, 40 and 45 °C (Fig. 10). With increasing temperature, the conversion of CrVI to CrIII was significantly improved (Fig. S2†) with a 100% conversion attained at 45 °C. This clearly indicated that higher temperatures can enhance the activity of the catalyst28 due to an increase in the accessible active surface area of the photocatalyst.29 This state of affairs arises because of the increased decomposition of formic acid to nascent hydrogen [H] at elevated temperatures, which also leads to an increase in the surface acidity of the catalyst, further accelerating the reduction reaction.30
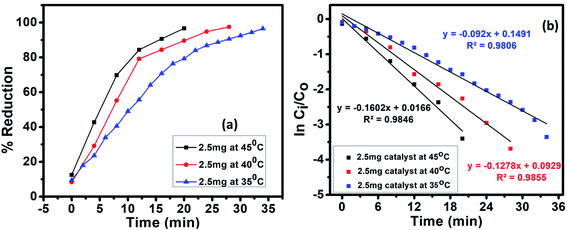 |
| Fig. 10 (a) Effect of temperature on the solar assisted catalytic reduction of Cr(VI) using an initial concentration of 100 mg L−1 of Cr(VI) and (b) the pseudo-first-order graph of ln(Ci/C0) versus time for Cr(VI) photocatalytic reduction. | |
4.5 Stability of the catalyst
The catalyst's stability was tested for five cycles and the results revealed that, in all cycles, CrVI was almost completely reduced, but it took a bit longer time wise (Fig. S3†). This small loss in stability is attributed to a slight loss of the catalyst during the cycling process. The XRD results for Cu1−xFexS (x = 9%), shown in Fig. S4 (ESI†), confirmed the stability and reusability of the catalyst. Therefore, the Cu1−xFexS (x = 9%) catalyst does exhibit considerable potential for the purification of contaminated water containing CrVI ions.
4.6 Proposed mechanism for the reduction of CrVI on Cu1−xFexS
A proposed mechanism for the conversion of CrVI to CrIII using Cu1−xFexS nanostructures is given in Fig. 11. This mechanism assumes that the CuS/Cu2S nanoparticles are the active centers for reduction. Photoexcitation of electrons to the CB leaves behind positive holes (h+) that facilitate the decomposition of the formic acid adsorbed on the surface of the catalyst to CO2 and hydrogen ion (H+). The photoexcited electrons are trapped by the dopant (Fe2+) to reduce hydrogen ions (H+) to nascent hydrogen [H] that subsequently reduces CrVI to CrIII.
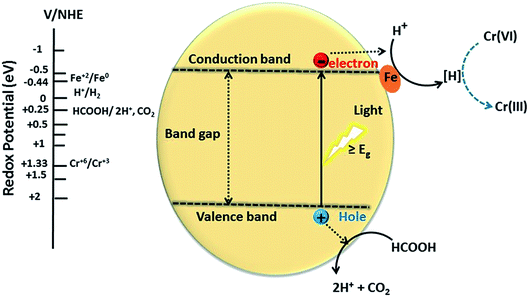 |
| Fig. 11 Proposed mechanism for the photocatalytic reduction of Cr(VI) over Cu1−xFexS. | |
The dopant (Fe2+) on the surface of the CuS acts as a co-catalyst that scavenges photoexcited electrons from the CB, thus facilitating an electron-transfer process in reduction and preventing the electron–hole recombination. The photo-excited electrons from the CB progress to the catalyst surface and transform the hydrogen ion to nascent hydrogen [H]. This hydrogen radical is highly reactive and efficiently reduces the CrVI of Cr2O72− to CrIII with water as a by-product:
|
3HCOOH(aq) → 2CO2 + 6e− + 6H+
| (4) |
|
Cr2O72− + 14H+ + 6e− → 2Cr3+ + 7H2O
| (5) |
Formic acid has a reduction potential (+0.25 eV) that is less positive or more negative than the CuS VB reduction potential (+2.05 eV). Consequently, CuS can oxidize HCOOH to CO2 and H+. On the other hand, the reduction potential of Fe2+/Fe0 (−0.44 eV) is more positive than the CuS CB reduction potential (−0.5 eV), so the transfer of electrons from the CB of CuS to the Fe2+ (dopant) is feasible due to the band off-set. The Fe0, therefore, provides a stable platform to reduce the protons to nascent hydrogen [H], which in turn acts as a reductant for hexavalent chromium.11,26,31
4.7 Apparent rate constant dependence on temperature
To observe the detailed kinetics of the CrVI photocatalytic reduction, the temperature dependence of the Langmuir–Hinshelwood mechanism was examined for Cu1−xFexS (x = 9%) nanoparticles. The hexavalent chromium reduction was performed at three different temperatures (35, 40 and 45 °C). The Ea (43 kJ mol−1) was calculated from the Arrhenius equation i.e., ln
k = ln
A − Ea/RT, by plotting ln
k vs. 1/T (Fig. S5†). The kinetic parameters, activation entropy (ΔS#) and enthalpy (ΔH#), were calculated using the Eyring equation i.e., ln
k/T = ln(kb/h) + ΔS#/R − ΔH#/R(1/T). The plot of ln
kapp/T vs. 1/T (Fig. S6†) have given a straight line with slope −ΔH#/R and intercept (ln(kb/h) + ΔS#/R) from which −ΔH# (156 kJ mol−1) and ΔS# (−197.51 J mol−1 K−1) were calculated. The positive ΔH# value reflects the endothermic nature of the reduction process.
The Fe doped CuS/Cu2S nanoplates are superior to previously reported32–35 nanoparticles (Table 1) for CrVI reduction: a 100% Cr reduction with a very small amount of catalyst in much less time. Iron doping improved the morphologies of NPs to provide a high contact area to trigger the reduction process with more surface atoms and unsatisfied valences. The iron dopant further enhanced the reduction process by acting as electron scavenger that prevented electron hole recombination.
Table 1 Comparison of the present work with the literature
S. no. |
Nanomaterial |
Catalyst loading |
Cr(VI) concentration |
Time (min) |
% reduction |
Reference |
1 |
9% Fe doped CuS/Cu2S |
2.5 mg/40 mL, 5 mg/40 mL, 10 mg/40 mL |
100 ppm |
36, 12, 6 |
100% |
Our work |
2 |
CM-n-TiO2 |
0.5 g L−1, 1 g L−1, 1.5 g L−1, 2.0 g L−1, 2.5 g L−1 |
3 ppm |
240, 120, 105, 30, 10 |
100% |
32 |
3 |
CZnO-dots |
3 mg mL−1 |
100 ppm |
300 |
100% |
33 |
4 |
TiO2 |
1 g L−1 |
100 ppm, 500 ppm |
120 |
89%, 84% |
34 |
5 |
FeOOH |
0.5 g L−1 |
20 ppm |
120 |
100% |
35 |
5. Conclusions
The results presented in this paper suggest that Fe as a dopant not only induced the morphological change in the CuS/Cu2S lattice, but also created a morphology-dependent redox shuttle mechanism. This morphology-dependent photoreduction of CrVI by Cu1−xFexS NPs under solar light irradiation is, indeed, a novel technique for harvesting solar energy and for the reduction of toxic CrVI ions in wastewater. Furthermore, Cu1−xFexS is a more efficient photocatalyst than the pristine CuS/Cu2S and other reported photocatalysts. This is because of the suitable reduction potential of Fe that facilitates electron transfer between CuS and H+ (generated from the oxidation of HCOOH by a hole). For Cu1−xFexS, a high activity was found at a high dopant concentration (9%), low pH (2.5) and elevated temperature.
Conflicts of interest
There is no conflict of interest.
Acknowledgements
We are thankful to the Higher Education Commission of Pakistan for their financial support.
References
- J. C. Crittenden, Y. Zhang, D. W. Hand, D. L. Perram and E. G. Marchand, Water Environ. Res., 1996, 68, 270–278 CrossRef CAS.
- L. Khalil, W. Mourad and M. Rophael, Appl. Catal., B, 1998, 17, 267–273 CrossRef CAS.
- S. N. Frank and A. J. Bard, J. Phys. Chem., 1977, 81, 1484–1488 CrossRef CAS.
- K. I. Okamoto, Y. Yamamoto, H. Tanaka, M. Tanaka and A. Itaya, Bull. Chem. Soc. Jpn., 1985, 58, 2015–2022 CrossRef CAS.
- J. Domenech and J. Costa, Photochem. Photobiol., 1986, 44, 675–677 CrossRef CAS.
- J. Doménech and A. Prieto, Electrochim. Acta, 1986, 31, 1317–1320 CrossRef.
- Y. Deng, L. Tang, G. Zeng, Z. Zhu, M. Yan, Y. Zhou, J. Wang, Y. Liu and J. Wang, Appl. Catal., B, 2017, 203, 343–354 CrossRef CAS.
- L. Shi, T. Wang, H. Zhang, K. Chang, X. Meng, H. Liu and J. Ye, Adv. Sci., 2015, 2, 1500006 CrossRef PubMed.
- H. Akcay, A. Oguz and C. Karapire, Water Res., 2003, 37, 813–822 CrossRef CAS.
- Y. Ku and I. L. Jung, Water Res., 2001, 35, 135–142 CrossRef CAS PubMed.
- N. Sreelekha, K. Subramanyam, D. A. Reddy, G. Murali, K. R. Varma and R. Vijayalakshmi, Solid State Sci., 2016, 62, 71–81 CrossRef CAS.
- Z. K. Heiba and M. B. Mohamed, Appl. Phys. A: Mater. Sci. Process., 2018, 6, 124 Search PubMed.
- A. Stroyuk, A. Kryukov, S. Y. Kuchmii and V. Pokhodenko, Theor. Exp. Chem., 2005, 41, 67–91 CrossRef CAS.
- N. u. Ain, Z. u. Rehman, A. Aamir, Y. Khan, M. u. Rehman and D. J. Laen, Mater. Chem. Phys., 2020, 242, 122408 CrossRef CAS.
- S. Gorai, D. Ganguli and S. Chaudhuri, Cryst. Growth Des., 2005, 5, 875–877 CrossRef CAS.
- Q. Lu, F. Gao and D. Zhao, Nano Lett., 2002, 2, 725–728 CrossRef CAS.
- B. Poornaprakash, D. A. Reddy, G. Murali, R. Vijayalakshmi and B. Reddy, Phys. E, 2015, 73, 63–68 CrossRef CAS.
- Y. Zhao, H. Pan, Y. Lou, X. Qiu, J. Zhu and C. Burda, J. Am. Chem. Soc., 2009, 131, 4253–4261 CrossRef CAS PubMed.
- E. Dilena, D. Dorfs, C. George, K. Miszta, M. Povia, A. Genovese, A. Casu, M. Prato and L. Manna, J. Mater. Chem., 2012, 22, 13023–13031 RSC.
- X. S. Hu, Y. Shen, L. H. Xu, L. M. Wang and Y. J. Xing, J. Alloys Compd., 2016, 674, 289–294 CrossRef CAS.
- F. Han, W. C. Li, D. Li and A. H. Lu, ChemElectroChem, 2014, 1, 733–740 CrossRef CAS.
- H. Saikia, B. J. Borah, Y. Yamada and P. Bharali, J. Colloid Interface Sci., 2017, 486, 46–57 CrossRef CAS PubMed.
- Y. Guo, D. Wang, X. Liu, X. Wang, W. Liu and W. Qin, New J. Chem., 2014, 38, 5861–5867 RSC.
- L. Liu, J. Xue, X. Shan, G. He, X. Wang and H. Chen, Catal. Commun., 2016, 75, 13–17 CrossRef CAS.
- M. C. Lu, G. D. Roam, J. N. Chen and C. Huang, J. Photochem. Photobiol., A, 1993, 76, 103–110 CrossRef CAS.
- T. Xu, J. Xue, X. Zhang, G. He and H. Chen, Appl. Surf. Sci., 2017, 402, 294–300 CrossRef CAS.
- A. Nezamzadeh-Ejhieh and N. Moazzeni, J. Ind. Eng. Chem., 2013, 19, 1433–1442 CrossRef CAS.
- M. A. Omole, I. O. K'Owino and O. A. Sadik, Appl. Catal., B, 2007, 76, 158–167 CrossRef CAS.
- J. Wen, X. Li, W. Liu, Y. Fang, J. Xie and Y. Xu, Chin. J. Catal., 2015, 36, 2049–2070 CrossRef CAS.
- S. Li, L. Tang, G. Zeng, J. Wang, Y. Deng, J. Wang, Z. Xie and Y. Zhou, Environ. Sci. Pollut. Res., 2016, 23, 22027–22036 CrossRef CAS PubMed.
- K. Bhowmik, A. Mukherjee, M. K. Mishra and G. De, Langmuir, 2014, 30, 3209–3216 CrossRef CAS PubMed.
- Y. A. Shaban, World J. Nano Sci. Eng., 2013, 3, 154 CrossRef CAS.
- P. Khare, A. Bhati, S. R. Anand, Gunture and S. K. Sonkar, ACS Omega, 2018, 3, 5187–5194 CrossRef CAS PubMed.
- M. A. Ahmed, A. T. Elsir, F. Mohammed, H. Elbushra, S. Tawer and N. Eassa, MRS Adv., 2018, 3, 2667–2674 CrossRef CAS.
- M. R. Samarghandi, J. K. Yang, O. Giahi and M. Shirzad-Siboni, Environ. Technol., 2015, 36, 1132–1140 CrossRef CAS PubMed.
Footnote |
† Electronic supplementary information (ESI) available. See DOI: 10.1039/d0ra04852f |
|
This journal is © The Royal Society of Chemistry 2020 |
Click here to see how this site uses Cookies. View our privacy policy here.