DOI:
10.1039/D0RA04835F
(Paper)
RSC Adv., 2020,
10, 24374-24385
Optimizing the optical and biological properties of 6-(1H-benzimidazole)-2-naphthalenol as a fluorescent probe for the detection of thiophenols: a theoretical study†
Received
1st June 2020
, Accepted 19th June 2020
First published on 25th June 2020
Abstract
The study presents the influence of structure modulation by introduction of selected donor and acceptor substituents on the properties of 6-(1H-benzimidazole)-2(2,4-dinitrobenzenesulfonate)naphthalene used in thiophenol identification. The presence of –OH and –OR groups enhances the non-linear optics (NLO) response of the marker. The –NO2 substituent maximizes the non-linear response and increases the amount of transferred charge and the charge-transfer distance. The introduction of the –OH, –NO2 and –CN groups into the marker structure significantly improves the solubility and optical availability. The –NO2 group however contributes to mutagenicity and carcinogenicity. The –OH and –OR groups can be successfully used in bioimaging to detect specific molecules containing the –SH group in their structure. At the same time, the –OR group minimizes the energy barrier necessary to break the bond between the chromophore and the linker. The paper also includes a comparison of optical and biological properties of structures before and after identification of thiophenols.
1 Introduction
Thiophenols are widely used in the synthesis of many industrial products, primarily polymers, pesticides and pharmaceutical products.1,2 Despite their widespread use, they are highly toxic and the average lethal dose (LC50) for fish is only 0.01–0.04 mM.3 Also, the human body is very exposed to the toxic effects of these substances due to inhalation and rapid absorption through the skin. Exposure of the human body to these compounds results in many serious illnesses, including polyposis, nausea, vomiting, muscle weakness and in special cases even death.4,5 Against this background, it is particularly important to search for new solutions for the easy and quick detection of thiophenols, especially in pharmaceuticals and in biological material. In this context, fluorescent probes, which are dyes relatively easy to manufacture with a wide spectrum of Stokes shifts, have become of practical importance. It is easy to modulate the structure of such compounds in order to optimize desirable photophysical features and they can be used in in vivo and in vitro tests. For detecting thiophenol derivatives, fluorescent probes are designed in such a way that the fluorophore is linked to an appropriate quencher unit to transfer the transformed molecule to another group with no quenching capability or cleaved from the fluorophore permanently. In this way, fluorescence is recovered and the last derivative is responsible for marker fluorescence. An example is 2,4-dinitrophenylsulphonyl (DNS).6–9 Many probes tailored for the detection of thiophenols are designed on the basis of the reaction between thiophenols and the sulfamide unit (RNH–SO2R′). However, thiophenol detection via such probes is relatively long and takes on average 20–30 min.10–12 The search for more effective solutions leads to the use of a sulfonyl ester (RO–SO2R ′), which significantly reduces the detection time.13
Based on this second strategy of fluorophore construction, the W. Lv14 group has synthesized 6-(1H-benzimidazole)-2-naphtalenol (BIN) as a satisfactory probe for the detection of thiophenols. It has been shown to have excellent photostability, good biocompatibility and a large Stokes' shift in aqueous solutions. By connecting the BIN to the sulfonyl ester group, the 6-(1H-benzimidazole)-2(2,4-dinitrobenzenesulfonate)naphtalene (BIN-T) probe was synthesized. In this construction, BIN is a fluorophore, while the 2,4-dinitrobenzenesulfonyl group is both a thiophenol recognition group and a fluorescence quenching unit (Scheme 1). For the marker constructed in this way, the average detection time of thiophenols was about 10 min.
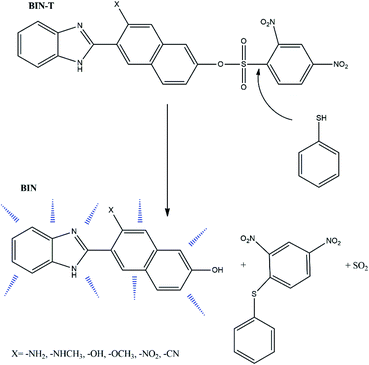 |
| Scheme 1 Detection pathway of thiophenol derivatives.14 | |
Due to the possibility of using BIN for the detection of thiophenols in pharmaceutical products and in biological material, it was decided to check which factors enhance the linear and non-linear optical response and improve biological properties. For this purpose, electron-donating and electron-withdrawing substituents have been attached to the naphthalene part. In this way, six derivatives (Scheme 1) with different photochemical and biological properties were obtained. The presented research results allow to answer the question how the probe structure should be modulated to obtain a marker with the desired photochemical properties required for molecular imaging. The study of marker structure modulation by changing substituents has a very important cognitive purpose. On the one hand, it allows achieving the desired optical and biological characteristics. On the other hand, it allows the creation of a fluorescent probe operating in a specific absorption and fluorescence range, depending on the research requirements.
2 Synthesis path
The method of synthesis of the tested derivatives is presented in Scheme 2.
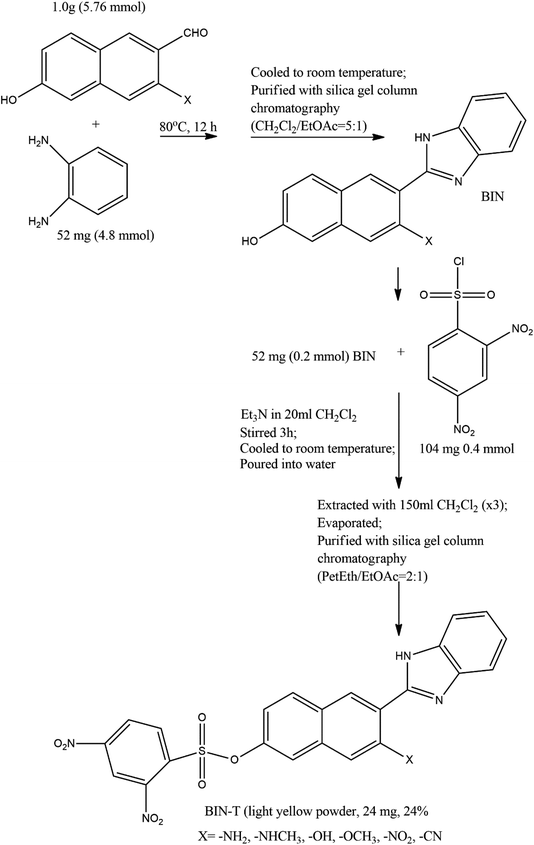 |
| Scheme 2 Synthesis of BIN-T probes (see ref. 14 for further details). | |
3 Computational details
All geometrical parameters of investigated molecules in their ground (SGS) and excited states (SCT) were calculated using density functional theory (DFT) approach implemented in Gaussian 09 program package15 with TIGHT threshold option and PBE0/6-311++G(d,p) basis set. In order to verify that all the structures correspond to the minima on the potential energy surface, an analysis of Hessians was performed. The electronic properties were characterized by computations of the vertical absorption and emission spectra, which were obtained using the time-dependent density functional theory (TDDFT/PBE0)16 and by including the state-specific (SS) corrected linear response (cLR) approach.17 Due to the high compatibility of theoretical and experimental data,18–21 all spectroscopic calculations were performed using PBE0 functional.
For the best consideration of the solvent impact on the fluorescence spectra, the ground state should be calculated with non-equilibrium solvation.22,23 This was taken into account by including the state-specific (SS) corrected linear response (cLR) approach24 to the theoretical calculations. In the SS approach the solvent dynamic polarizations are determined by the difference of the electron densities of the initial and final states.25–27
The dipole moments and polarities of the charge-transfer state (SCT) were evaluated by numerical differentiation of the excitation energies (E) in the presence of an electric field F of 0.001 a.u. strength:
|
 | (1) |
where
i stands for the Cartesian component of the dipole moment difference and g is ground state (S
g). The isotropic average polarizability (〈
α〉), polarizability anisotropy (Δ
α) and first-order hyperpolarizability (
βvec) were determined based on the Gaussian 09 program and defined as:
|
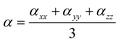 | (2) |
|
 | (3) |
|
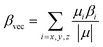 | (4) |
where
βi (
i =
x,
y,
z) is given by
The density differences were obtained at the PBE0/6-311++G(d,p) level and are represented with a contour threshold of 0.02 a.u. In these graphs, the blue (purple) zones indicate density decrease (increase) upon electronic transition. The charge transfer parameters, namely the charge-transfer distance (DCT) and the amount of transferred charge (qCT), have been determined following a Le Bahers' procedure.28 The solvent effect on the linear and nonlinear optical properties has been taken into account using the Integral Equation Formalism for the Polarizable Continuum Model (IEF-PCM).29,30
Experimentally, the two-photon absorption (TPA) can be obtained by the dissipation of the incident light, which for a single beam 2PA experiment is twice the transition rate. In this case, the two-photon cross-section of the degenerate process is written as:31–33
|
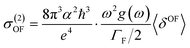 | (5) |
where
α is a fine structure constant,
ω is the frequency of absorbed photons (assuming one source of photons),
ΓF is the broadening of the final state (F) due to its finite lifetime and
g(
ω) provides the spectral line profile, which often is assumed to be a
δ-function and 〈
δOF〉 is the two-photon transition probability for the transition from the ground state to a final state.
In the case of a molecule absorbing two photons of the same energy in isotropic media, the degenerate 〈δOF〉 in an isotropic medium using a linearly polarized laser beam given by:34
|
 | (6) |
In this equation, SOFij is the second-order transition moment given by:
|
 | (7) |
where
ℏω1 +
ℏω2 should satisfy the resonance condition and

stands for the transition moment between electronic states |0〉 and |
K〉, respectively.
ζ is the vector defining polarization of photons. To describe the two-photon allowed states, the quadratic response functions formalism
35,36 within the DFT framework was used as implemented in the DALTON 2011 program.
37,38 Solvent effects were taken into account with the self-consistent reaction field (SCRF) model. All the 2PA calculations were carried out employing the CAM-B3LYP functional and the 6-311++G(d,p) basis set.
The biological activities were simulated using a combination of the 3D/4D QSAR BiS/MC and CoCon algorithms.39–43
The binding properties of considered dyes were studied by performing series of AutoDock 4.2 (ref. 44–46) and AutoDock Vina47 simulations. For each active complex comprising Human Serum Albuminum (HSA), their crystal structures were taken from PDB ID: 1AO6.48 The cubic grid box with the dimensions of 16 Å and a grid spacing of 1 Å was set up in such way that the reactive –NH2 groups of lysine and –SH of cysteine were at its centre. In order to identify appropriate binding energy and conformation of compounds, the Lamarckian genetic algorithm was employed. For each atom of the receptor molecule Gasteiger charges were calculated. The investigation of the binding site was performed using a united-atom scoring function. For each amino acid the docking simulations were performed tenfold.
4 Results
4.1. Physicochemical properties
For all investigated molecules, the charge-transfer (CT) excitation corresponds to the HOMO → LUMO transition (Fig. 1 and 2). In the case of the BIN-T probe, HOMO electrons are located on the 6-(1H-benzimidazole)-2-naphtalenol part, while LUMO on the 2,4-dinitrobenzenesulfonyl group. The attachment of substituents, as well as their change, does not affect the position of these frontier orbitals. Reaction with thiophenol and obtaining BIN fluorophore significantly changes this distribution. HOMO electrons accumulate throughout the entire molecule, while LUMO mainly accumulates on the naphthalene part. This is particularly apparent for the BIN–NO2 derivative. For BIN-T derivatives, there are slight differences in the case of energy separation between HOMO–LUMO orbital (EGAP) – Table SI1.† Attachment of the –NH2 and –NHR substituents reduces EGAP by 0.55 eV on average. In turn, the presence of –OH reduces it by 0.2 eV and the presence of –OR brings this value closer to the BIN-T level. The presence of electron-acceptor substituents increases the EGAP value by more than 0.3 eV. After thiophenol detection (Table SI2†), the EGAP value increases significantly for water ΔEBIN–BIN-TGAP = 1.5569 eV. In this case, the influence of substituents is slightly different. The presence of –NH2 reduces it by 0.2 eV while –NHR does not change this value. The –OH and –OR substituents slightly increase EGAP and the electron-acceptor ones decrease it. For both BIN and BIN-T derivatives, no significant effect of solvent presence on the EGAP value is observed. In general, for BIN-T derivatives with increasing polarity of the medium EGAP increases (
= 0.1970 eV) and for BIN decreases (
= 0.0160 eV). In addition, a significant decrease (by more than 1 eV) in chemical hardness (η) is observed for BIN-T derivatives. High electronegativity (χ) value suggests an easy formation of covalent bonds during various chemical processes.
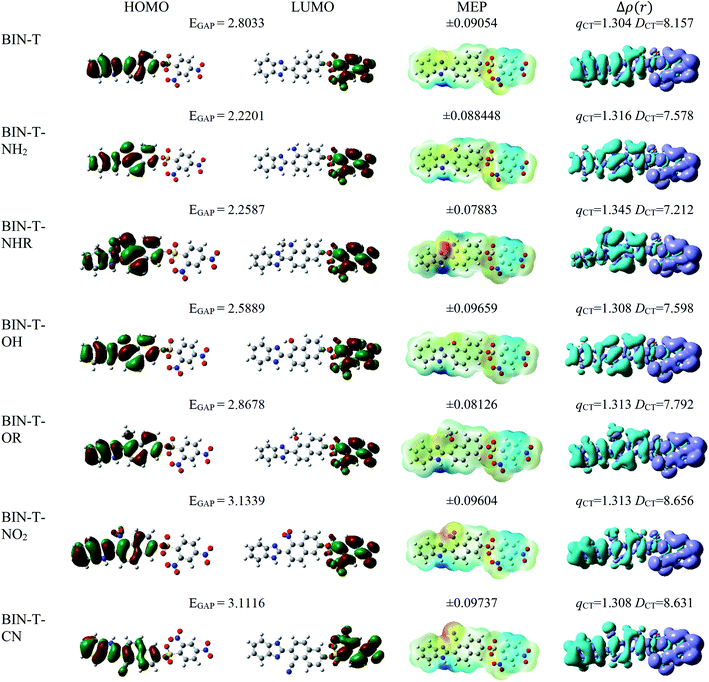 |
| Fig. 1 Physicochemical properties of BIN-T derivatives. | |
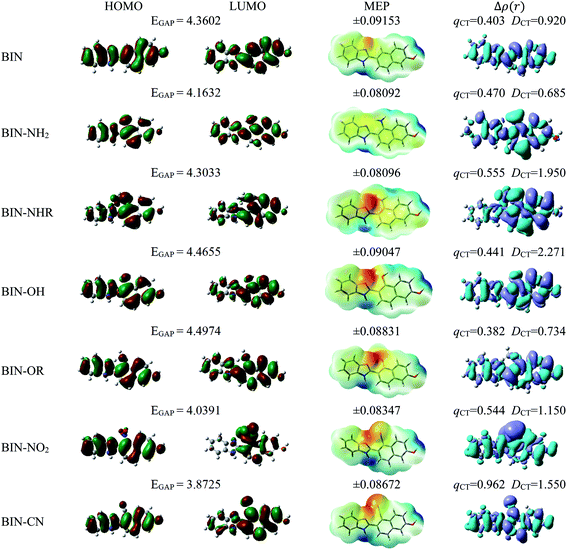 |
| Fig. 2 Physicochemical properties of BIN derivatives. | |
In order to assess the sites of potential electrophilic and nucleophilic attack, the molecular electrostatic potential (MEP) analysis was performed (Fig. 1 and 2). In the case of BIN-T, the place with the highest electronegativity and thus the most exposed to nucleophilic attack (positive, blue zones) is the nitrogen atom with hydrogen attached in the imidazole part. Benzene in the 2,4-dinitrobenzenesulfonyl part is also a region that may undergo nucleophilic reactions. Substitution with subsequent substituents does not change the location of these places. Only for BIN-T–NH2 and BIN-T–NHR positive zones are also observed on the nitrogen atoms of substituents. Sites for electrophilic attack (red and yellow, negative zones) are mainly the second of the nitrogen atoms of the imidazole part and the oxygen atom in the sulfonylester group. The presence of substituents forces the negative zones to shift into the regions of the connected groups. Among the considered derivatives, the highest risk of electrophilic attack is experienced by BIN-T–CN, where the charge on the nitrogen atom of the –CN group is as much as −0.09737 a.u. After the detection of thiophenols and obtaining the BIN structure, the nitrogen atom of the imidazole group is still the place with the highest risk of nucleophilic attack. In addition, an important place for such a reaction is the hydrogen atom of the hydroxyl group. The place for the electrophilic attack remains the second nitrogen atom of the imidazole ring and substitution with subsequent substituents does not change these regions. Similar to BIN-T, negative zones also appear on substituents. It can also be seen that the attachment of –NH2 slightly reduces the charge on the nitrogen atom, reducing the risk of an electrophilic reaction.
The studies have been devoted to many reflections on spectral properties, corresponding to the HOMO → LUMO photoexcitation (π–π* transitions). In order to estimate contributions from other orbitals and determine the nature of electronic states, the density variation upon photoexcitation (Δρ(r)) was computed for the first electronic transitions, which is graphically depicted in Fig. 1 and 2. For BIN-T, the figure indicates that the depletion zones (blue) are located on 2-(naphthalen-2-yl)-1H-benzo[d]imidazole, while in contrast, growth zones (purple) on 2,4-dinitrobenzenosulfonyl part. The presence and change of donor–acceptor substituents does not change the position of these zones. In the case of BIN derivatives, the zones of electron density decay are visible throughout the entire molecule, and the increase zones mainly on naphthalene. Also in this case, the substituent change does not move these zones. On the other hand, the polarity of the environment affects the parameters describing Δρ(r) (Table SI3†). For both BIN-T and BIN derivatives, the amount of the transferred charge drops as a function of solvent polarity. In addition, the value of qCT is higher for BIN-T, and the ΔqBIN-T–BINCT difference is 0.9 a.u. The same analogy applies to other derivatives. In addition, the introduction of the –NO2 substituent increases the value of qCT and
is 0.5 a.u. The polarity of the environment also significantly affects the charge-transfer distance and decreases it from 1.682 Å in 1,4-Dx to 0.920 Å in water for BIN. In turn, for BIN-T, this quantity increases from 7.503 Å to 8.157 Å. At the same time, BIN-T derivatives have a higher DCT value and ΔDBIN-T–BINCT = 7.2 Å. The presented analysis indicates the charge-transfer nature of BIN and BIN-T derivatives. It also confirms the contributions from the HOMO–LUMO transition. At the same time, it indicates the possibility of the presence of contributions from other orbitals. Therefore, additional low intensity peaks can be seen in absorption and fluorescence spectra.
The BIN-T molecule is characterized by good solubility in all discussed media. ΔGsolv increases with increasing solvent polarity, going from −17.62 kcal mol−1 in 1.4Dx to −20.37 kcal mol−1 in water, with the highest value being reached in MeCN (−29.06 kcal mol−1). While similar behavior is observed for other molecules, the introduction of –NHR, –OH, –NO2 and –CN significantly improves the solubility of the compound. The presence of the remaining substituents slightly increases the ΔGsolv value by reducing solubility. Similar conclusions can be drawn after analyzing BIN derivatives. More importantly, the change in structure after thiophenol identification results in a significant reduction in solubility and for BIN ΔGBIN-T–BINsolv in 1,4Dx is 5.59 kcal mol−1 and in water 1.85 kcal mol−1. It should also be clearly stated that in terms of ΔGsolv the desired substituents will be –OH, –NO2 and –CN, the presence of which significantly improves the solubility of the compound and thus its optical availability.
4.2. Linear optical properties
Theoretical linear optical properties of analyzed markers are shown in Tables 1 and SI5.† Due to the high compatibility of theoretical and experimental values obtained on the basis of PBE0,18–21 the spectroscopic parameters were determined using this function. It also accurately predicts BIN-T values experimentally recorded after thiophenol detection. For vertical values, the ΔλTDDFT-ExpABS difference is only 1.63 nm. In the case of BIN-T derivatives, the presence of donor–acceptor substituents results in a shift in absorption maximum (λABS). In the presence of water, donor groups cause bathochromic shift, and acceptor groups cause hypsochromic shift. In the first case, the largest shift of maximum λABS is a result of the presence of the –NHR group. Considering the effect of substituents on the magnitude of the solvatochromic shift, the analyzed derivatives can be ordered as follows: BIN-T–NHR (31.25 nm) > BIN-T–NH2 (24.97 nm) > BIN-T–OR (9.39 nm) > BIN-T–OH (3.94 nm) > BIN-T (0 nm) > BIN-T–CN (−9.60 nm) > BIN-T–NO2 (−10.08 nm). Slightly different conclusions can be drawn after analyzing BIN derivatives. In this case, acceptor substituents cause bathochromic shift, while –OH and –OR moves the maximum of λABS towards shorter wavelengths: BIN–NO2 (84.63 nm) > BIN–CN (33.25 nm) > BIN–NH2 (21.85 nm) > BIN–NHR (13.58 nm) > BIN (0 nm) > BIN–OH (−6.35 nm) > BIN–OR (−8.62 nm). Moreover, detection of thiophenols and achieving BIN structure induces hypsochromic shift. The highest ΔλBIN-T–BINABSABS values are observed for donor substituents. In –NO2 there is a bathochromic shift: –OR (88.86 nm) > –NHR (88.52 nm) > –OH (81.14 nm) > –NH2 (73.97 nm) > BIN (70.85 nm) > –CN (28.00 nm) > –NO2 (−23.86 nm).
Table 1 Linear and nonlinear optical properties in water
|
λTDDFTABS |
λcLRABS |
λTDDFTEM |
μGS |
μCT |
〈α〉 |
Δα |
βvec |
〈δOF〉 |
σ(2)OF |
BIN |
331.63 |
330.62 |
460.31 |
3.43 |
7.49 |
342.96 |
340.39 |
1169.43 |
27.74 |
0.13 |
BIN–NH2 |
353.48 |
350.81 |
471.48 |
1.92 |
4.08 |
364.64 |
387.01 |
2223.18 |
339.40 |
1.44 |
BIN–NHR |
345.21 |
351.50 |
436.62 |
5.26 |
7.05 |
363.73 |
307.71 |
799.39 |
351.51 |
1.56 |
BIN–OH |
325.28 |
334.03 |
462.75 |
5.81 |
6.78 |
340.17 |
332.24 |
1020.66 |
103.13 |
0.49 |
BIN–OR |
323.01 |
322.42 |
462.65 |
5.18 |
7.91 |
356.04 |
328.75 |
514.84 |
45.17 |
0.23 |
BIN–NO2 |
416.26 |
400.71 |
540.55 |
7.87 |
14.07 |
359.18 |
337.84 |
88.14 |
217.52 |
0.91 |
BIN–CN |
364.88 |
364.84 |
507.30 |
8.39 |
11.18 |
363.82 |
350.91 |
257.54 |
442.17 |
1.87 |
BIN-T |
402.48 |
405.31 |
582.65 |
1.83 |
5.11 |
443.19 |
517.69 |
7.07 |
3608.61 |
13.17 |
BIN-T–NH2 |
427.45 |
426.44 |
627.74 |
2.38 |
5.22 |
462.83 |
540.67 |
645.13 |
2872.63 |
7.47 |
BIN-T–NHR |
433.73 |
435.31 |
623.63 |
3.49 |
5.97 |
383.38 |
539.01 |
694.93 |
1806.53 |
4.95 |
BIN-T–OH |
406.42 |
409.95 |
520.00 |
3.95 |
5.46 |
451.19 |
525.36 |
2049.36 |
2197.89 |
7.14 |
BIN-T–OR |
411.87 |
416.81 |
607.05 |
1.69 |
4.84 |
407.28 |
529.48 |
1760.67 |
2599.40 |
9.76 |
BIN-T–NO2 |
392.40 |
390.72 |
606.48 |
5.23 |
10.46 |
418.86 |
534.72 |
2184.23 |
2403.32 |
10.27 |
BIN-T–CN |
392.88 |
394.25 |
525.50 |
5.92 |
10.37 |
403.73 |
501.07 |
1496.24 |
5864.79 |
25.33 |
Considering the effect of the solvent, negative solvatochromism is observed for BIN-T derivatives and the maximum absorption is shifted in the direction of shorter wavelengths with increasing solvent polarity. This effect is observed regardless of the nature of the attached substituent, both for vertical values and the ones determined based on the cLR model. Also, for all BIN derivatives non-monotonic solvatochromism is observed. The excitation energy values EEX increase as a function of the polarity of the medium, however the transition from MeCN to DMSO is followed by a slight decrease in EEX. The only exception from the above results is the BIN–NO2 derivative, for which positive solvatochromism is observed.
The interconnectedness of the analysis of spectroscopic parameters with MEP indicates the possibility of specific interactions in the solute–solvent system. A non-monotonic increase in excitation energy indicates on larger polarization and better Sg stabilization. However, this is not consistent with the polarity of the excited state (ΔμCT-g, eqn (1)). Hypsochromic shift, as the effect of the increase in environmental polarity, should result in the μg > μCT relationship. In contrast, for both BIN-T and BIN derivatives there is an inverse relationship (Table SI6†), which is characteristic of positive solvatochromism. In any case, the polarity of the excited state also changes non-monotonously. In water, for BIN-T derivatives, the highest ΔμCT-g value is characteristic for markers with electron-withdrawing substituents:
(5.23 D) > Δμ–CNCT-g (4.45 D) > ΔμBIN-TCT-g (3.28 D) > Δμ–ORCT-g (3.15 D) >
(2.84 D) > Δμ–NHRCT-g (2.48 D) > Δμ–OHCT-g (1.51 D). In the case of BIN derivatives, again the presence of –NO2 results in the highest polarity of the excited state, however the BIN molecule has a higher ΔμCT-g value than BIN–CN:
(6.20 D) > ΔμBINCT-g (4.06 D) > Δμ–CNCT-g (2.79 D) > Δμ–ORCT-g (2.73 D) >
(2.16 D) > Δμ–NRCT-g (1.79 D) > Δμ–OHCT-g (0.97 D). In addition, after thiophenol identification, the polarity of the excited state decreases, except for BIN–NO2 and BIN.
Tables 1 and SI7† show the values of the de-excitation energy (λFL). Also in this case the functional PBE0 perfectly reproduces the values and ΔλTDDFT-ExpFL is only 0.31 nm. The increasing polarity of the environment causes non-monotonic solvatochromism, both for BIN-T and BIN derivatives. More importantly, thiophenol identification results in a hypsochromic shift at the maximum fluorescence position. The highest value of this shift (ΔλFL) is characteristic for donor substituents: Δλ–NHRFL (187.01 nm) >
(156.26 nm) > Δλ–ORFL (144.40 nm) > ΔλBINFL (122.34 nm) >
(65.93 nm) > Δλ–OHFL (57.25 nm) > Δλ–CNFL (18.20 nm). In addition, in line with experimental relationships, for BIN-T fluorescence intensity is negligible (Fig. 3). Identification of thiophenols and detachment of 2,4-dinitrobenzenesulfonyl group significantly increases the intensity of λFL. At the same time, it can be observed that with the exception of BIN and BIN–NHR, a second, less intense fluorescence band appears shifted towards shorter wavelengths. In general, the used substituents significantly shift the maximum λFL towards longer wavelengths, and only the –NHR substituent results in hypochromic shift relative to BIN:
(80.24 nm) > Δλ–CNFL (46.99 nm) >
11.17 nm) > Δλ–OHFL (2.44 nm) > Δλ–ORFL (2.34 nm) > ΔλBINFL (0 nm) > Δλ–NHRFL (−23.59 nm).
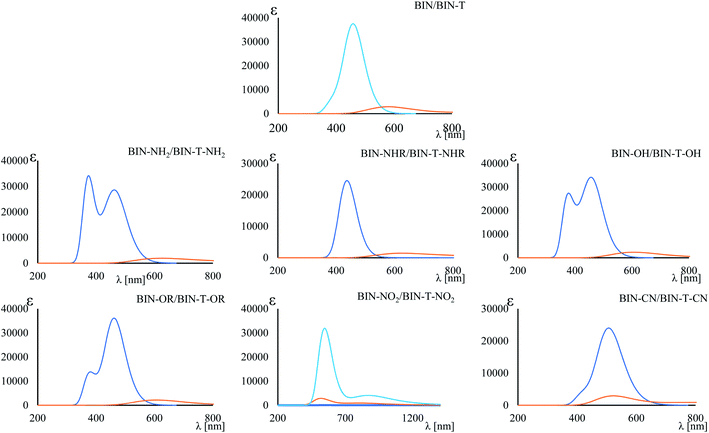 |
| Fig. 3 Maximum fluorescence band. Blue line – BIN derivatives, orange line – BIN-T derivatives. | |
All BIN derivatives have a high Stokes shift value (ΔνSt). In each case, its value increases monotonously as a function of the solvent polarity. However, while for λABS and λFL donor and acceptor substituents have some relationship, in this case there is none. For water, the largest ΔνSt values are found for BIN–OH and BIN–OR derivatives, which are 9132.80 cm−1 and 9344.82 cm−1, respectively. The lowest value is observed for BIN–NO2, amounting to 5523.77 cm−1. By ordering these derivatives in terms of ΔνSt versus BIN: BIN–OR (914.58 cm−1) > BIN–OH (703.21 cm−1) > BIN (0 cm−1) > BIN–CN (−735.53 cm−1) > BIN–NH2 (−1349.26 cm−1) > BIN–NHR (−2364.93 cm−1) > BIN–NO2 (−2905.83 cm−1), it can be concluded that the presence of the –OH and –OR substituents maximizes the linear response, thus increasing their detection capabilities. Although all derivatives are described by a high ΔνSt value, the presence of –NO2 will be the least desirable substituent in the marker structure.
4.3. Nonlinear opical properties
The polarizability (Δα, eqn (3)) and first hyperpolarizability (βvec, eqn (4)) of molecules irradiated with intense laser light giving the electric field is the subject of many research in terms of understanding various nonlinear optical properties (NLO). In particular, these studies include the interrelationship of NLO with the electronic structure to design new multifunctional fluorescence probes. The calculated values of Δα and βvec are collected in Tables 1 and SI8.† Firstly, for both BIN and BIN-T derivatives, the Δα and βvec values depend on the presence of the solvent. They increase monotonously as a function of the polarity of the medium. In addition, the polarizability values for BIN-T derivatives are on average 150 a.u. higher than BIN. In the case of the first hyperpolarizability, it depends on the structure of the probe and e.g. βBINvec > βBIN-Tvec,
, βBIN-T–NHRvec > βBIN–NHRvec, βBIN-T–ORvec > βBIN–ORvec etc. More importantly, changing substituents causes changes in the nonlinear response of the system. In the case of Δα, if the values are similar, the analyzed compounds can be ordered in the following way: BIN-T–NH2 > BIN-T–NHR > BIN-T–NO2 > BIN-T–OR > BIN-T–OH > BIN-T > BIN-T–CN and BIN–NH2 > BIN–CN > BIN–NHR > BIN–NO2 > BIN–OR > BIN > BIN–OH. In the case of βvec, these series are as follows: BIN-T–NO2 > BIN-T–OH > BIN-T–OR > BIN-T–CN > BIN-T–NHR > BIN-T–NH2 > BIN-T and BIN–NH2 > BIN > BIN–OH > BIN–NHR > BIN–OR > BIN–CN > BIN–NO2. Since the non-linear response will be derived from the structure obtained after thiophenol identification, the factor enhancing the NLO response will be the presence of the –NH2 group and can be efficient in Second Harmonic Generation (SHG).
Tables S1 and SI9† show the value of two-photon absorption cross section (TPA, 〈δOF〉 eqn (6)). In general, it is difficult to decisively conclude about the effect of the solvent on the 〈δOF〉 value. Non-monotonic solvatochromism is observed for BIN-T, whereas negative solvatochromism is observed for BIN. Positive solvatochromism is revealed in the values describing BIN–NH2 and BIN-T–OR. In addition, the values for BIN-T derivatives are on average 3.000 a.u. higher than for BIN. However, in order to compare the calculated values of the TPA with those determined experimentally (σ(2)OF, eqn (5)), the relation (5) was used. In this equation, the broadening of the final state due to its finite lifetime 0.25 eV was assumed. The effect of the solvent on the σ(2)OF value remains the same as for 〈δOF〉. More importantly, although BIN-T derivatives have a higher σ(2)OF value, none of the analyzed markers meet the requirements for use in two-photon imaging. BIN-T–CN (25.33 GM) has the highest value, while BIN (0.13 GM) has the lowest one. Thus, these values indicate a very poor NLO response signal. In the case of BIN-T derivatives, only the presence of the –CN substituent is a factor increasing the non-linear response. The remaining substituents reduce the σ(2)OF value, with the highest minimization observed for –NHR. For BIN derivatives, each substituent increases the two-photon absorption cross section value. The largest increase is observed for BIN–NH2, –NHR and –CN. Based on this analysis, to obtain a probe with a high TPA value, used as tools in real-time dynamic in vivo and in vitro research, an additional chromophore group intensifying the NLO response should be added to the structure of the analyzed dyes.
4.4. AutoDock simulations
BIN-T is a probe for detecting thiophenols in solutions. As mentioned earlier (Scheme 1), the identification reaction occurs by 6-(1H-benzimidazole) −2 (2,4-dinitrobenzenesulfonate) naphthalene reacting with thiophenol sulfur. Therefore, this marker can also be an alternative as a fluorescent probe used in medical imaging. In this case, protein conjugation occurs by reaction with cysteine. Human Serum Albuminum (HSA) was selected for this study. For BIN-T derivatives, the presence of a substituent or its change does not alter the site of active binding to the protein. In each case, conjugation to HSA occurs via CYS448 (Fig. 4). The BIN-T–CN marker has the highest affinity for this active center, for which binding energy (ΔGb) is −9.9 kcal mol−1 (Table SI10†). This active binding site has an inhibition constant (Ki) of 1.00 μM. The BIN-T–CN is inserted into the aromatic cage formed by CYS448, ASN295, ARG218, LYS444, PRO447, TYR452 and VAL455. In this active site, no π–π* interactions occur and the system is not stabilized by the presence of a H-bond. BIN-T–OH and BIN-T have slightly lower affinity for HSA and ΔGb = −9.2 kcal mol−1. Inhibition constant is 1.57 μM and 2.53 μM, respectively. The active impact cavity here is: LYS199, LYS195, LEU198, VAL455, ASN295, ARG218 and LYS444. In the case of BIN-T–OH, TRP214 also interacts here and in BIN-T – ASP451. Also in this case no hydrogen bonds are observed and no π–π* interactions. For other probes ΔGb is −9.1 kcal mol−1. Only for BIN-T–NHR a hydrogen bond is formed between the oxygen of the sulfone group and the hydrogen of LYS444. In addition, the lowest Ki value of 0.63 μM was recorded for BIN-T–NO2.
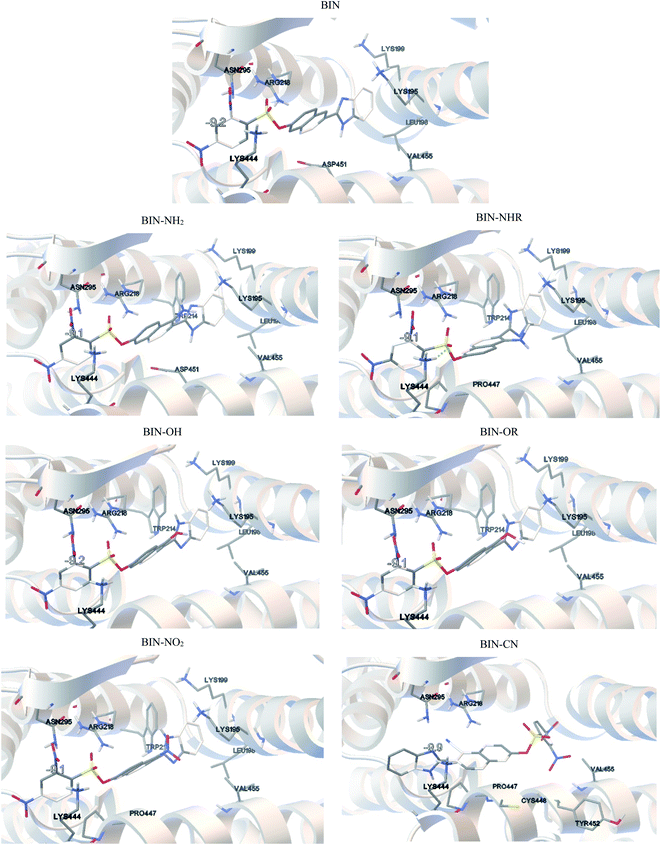 |
| Fig. 4 Results of the AutoDock simulations. | |
During conjugation, the O–S bond raptures and then a new one forms with the protein. The speed of biocomplex creation will therefore also be affected by the energy barrier (ΔE) necessary to overcome. According to Fig. SI1,† the lowest ΔE value when moving the oxygen atom away from the sulfur atom at a distance of 2.5 Å is observed for BIN-T–OR and is 14.5 kcal mol−1. A slightly higher value is observed for BIN-T-OH, for which ΔE = 16.87 kcal mol−1. The strongest bond, and thus hindering the conjugation, is the O–S in BIN–CN, where ΔE = 26.63 kcal mol−1.
4.5. Biological activities
The BIN-T derivatives are described by relatively good bioavailability (log
P > 5). Only for BIN-T–NHR this value drops slightly and amounts to 4.70 ± 0.25. This suggests good permeability through cell membranes and achieving adequate concentration at the site of interaction with thiophenols. In turn, BIN derivatives are described with a log
P value in the range of 3–4. The exceptions are BIN–NH2 and BIN–NHR, for which log
P < 3. The log
BCF value calculated for BIN-T in the range from −4.2 to −4.5 indicates the lack of bioaccumulation in the tissues of living organisms and the ease of excretion with urine. After identifying thiophenols, log
BCF decreases by one unit (in the range from −3.0 to −3.3). Despite this, BIN derivatives should not bioaccumulate after fulfilling their optical role. In addition, both BIN-T and BIN derivatives are characterized by high metabolism by CYP450-2D6 and CYP450-3A4 (probability above 80%) (Tables SI11 and SI12†). This indicates that both forms of markers will be rapidly excreted from tissues without interacting with other biomolecules and drugs.
The calculated oral toxicity value is LD50 > 1500 mg kg−1. Therefore BIN-T and BIN derivatives should be classified in class 4 in terms of the degree of toxicity and can be considered as practically nontoxic for humans. While the presence and change of a substituent does not affect the toxicity of presented molecules, it does, however, affect other toxicological parameters (Fig. 5). None of the derivatives shows hepatotoxicity and cytotoxicity. The presence of the NO2 substituent causes carcinogenicity (probability P = 58%) and mutagenicity (P = 72%). The –OR group may affect the occurrence of immunotoxicity (P = 66%). Immunotoxicity can elicit the reference BIN marker (P = 57%). Other probes should not cause any toxic effects. Thus, the introduction of a substituent into the BIN structure eliminates the possible toxic effects of the molecule. In addition, both BIN-T and BIN derivatives have other biological activities suggesting their potential use in other areas of medicine (Tables SI11 and SI12†). First of all, BIN-T derivatives are characterized by high antioxidant activity (P> 48%), in particular BIN-T–CN (P = 90%). For BIN derivatives probability of this activity drops to zero, except for BIN–NHR (P = 27%) and BIN–OR (P = 39%). In turn, for BIN-T derivatives no antibacterial activity is observed, while it can be attributed to BIN derivatives, in particular BIN–OH (P = 79%), BIN–NO2 (P = 79%) and BIN–CN (P = 80%). The following tables indicate the occurrence of many other activities, such as: alpha-radioprotector, analgetic, anti-psychotic activity diazepine site, anti-tumor alkylic, anti-tumor antimiotic, HIV1-proteaze inhibitory activities and many others. At the same time, the influence of subsequent substituents on the maximization or reduction of these activities is demonstrated there.
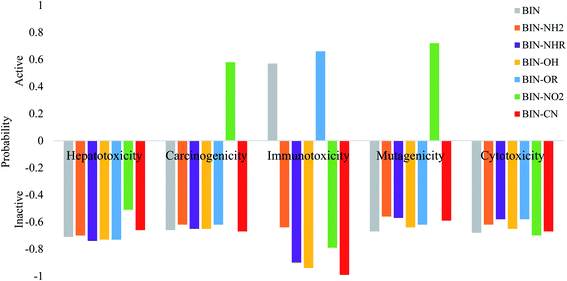 |
| Fig. 5 Theoretically determined toxicological parameters. | |
5 Conclusion
The manuscript presents previously unavailable observations on the influence of donor–acceptor substituents (D/A) on optical parameters and biological activity of the fluorescent probe used to identify thiophenols. This type of research is the first for the derivatives in question and is valuable information for researchers synthesizing new compounds on the benzimidazole backbone. It has been demonstrated that the used D/A substituents do not significantly affect the position of HOMO–LUMO orbitals and only slightly affect the EGAP value. They also do not change the sites for nucleophilic and electrophilic attack and density depletion/increment zones upon photoexcitation. However, it has been shown that the –NO2 substituent increases the amount of transferred charge and the charge-transfer distance. Studies have shown that the introduction of the –OH, –NO2 and –CN groups into the marker structure significantly improves the solubility and thus the optical availability of the probe. The used substituents cause the solvatochromic shift of the absorption and fluorescence maxima with respect to the experimentally tested marker, and the solvatochromic relationships depend on the structure before and after identification of thiophenols. These observations is extremely important for scientists synthesizing and studying benzimidazole derivatives. Taking into account the Stokes shift value, it can be concluded that the presence of the –OH and –OR substituents maximizes the linear response and increasing detection of the marker. However, the reducing factor will be the presence of –NO2. On the other hand, the –NO2 substituent maximizes the non-linear response. Nevertheless, the presence of –OR and –OH also enhances NLO values relative to pure dye. The tested derivatives are not used in two-photon imaging. However, this observation may be a clue to search for other substituents enhancing the two-photon story. The analyzed structures show high affinity for HSA, and a change in the D/A substituent slightly reduces binding energy. Therefore, the presented compounds can be used with great success in in vivo and in vitro tests as fluorescent probes for detecting specific biomolecules containing the –SH group. Therefore, the considered derivatives constitute valuable compounds for the pharmaceutical and medical market. At the same time, the –OR group minimizes the energy barrier necessary to break the bond between the chromophore and the linker, which speeds up and facilitates accessibility during conjugation with protein. All derivatives are characterized by good permeability through cell membranes and no bioaccumulation. A very valuable observation is the fact that, toxicity studies excluded the attractiveness of using the –NO2 substituent in in vivo and in vitro studies due to the potential for carcinogenicity and mutagenicity. By modulating the appropriate D/A substituents on the BIN structure, many valuable drugs can be obtained in the treatment of cancer, HIV, oxidative stress regulation, etc. Therefore, the conducted research indicates the high value of these derivatives in medicine. Considering all the above analyzes, it should be clearly stated that the presence of the –OR and –OH substituents significantly maximizes the linear and non-linear optical response of the molecule used as a fluorescent probe in medical imaging.
Abbreviations and notations
1,4-Dx | 1,4-Dioxane |
MeCN | Acetonitrile |
DMSO | Dimethylsulfoxide |
CH2Cl2 | Dichloromethane |
EtOAc | Ethyl acetate |
PetEth | Petroleum ether |
CT | Charge-transfer excitation |
ΔGsolv | Free energies of solvation |
G | Ground state |
HSA | Human serum albuminum |
ΔGb | Binding energy |
Ki | Inhibition constant |
ΔE | Energy barrier |
log P | Octanol–water partition coefficients |
CYP2D6 | Cytochrome P450-2D6 |
CYP450-3A4 | Cytochrome CYP450-3A4 |
P | Probability of occurrence |
LD50 | Lethal dose, determination of the toxicity of the substance |
Conflicts of interest
The authors declare that there are no potential financial or non-financial conflicts of interest.
Acknowledgements
This research was supported in part by PLGrid Infrastructure.
References
- K. Shimada and K. Mitamura, J. Chromatogr. B: Biomed. Sci. Appl., 1994, 659, 227–241, DOI:10.1016/0378-4347(93)e0444-u.
- A. Eychmuller and A. L. Rogach, Pure Appl. Chem., 2000, 72, 179–188, DOI:10.1351/pac200072010179.
- T. P. Heil and R. C. Lindsay, J. Environ. Sci. Health, Part B, 1989, 24, 349–360, DOI:10.1080/03601238909372654.
- P. Amrolia, S. G. Sullivan, A. Stern and R. Munday, J. Appl. Toxicol., 1989, 9, 113–118, DOI:10.1002/jat.2550090208.
- T. R. Juneja, R. L. Gupta and S. Samanta, Toxicol. Lett., 1984, 21, 185–189, DOI:10.1016/0378-4274(84)90204-2.
- K. B. Li, D. Zhou, X. P. He and G. R. Chen, Dyes Pigm., 2015, 116, 52–57, DOI:10.1016/j.dyepig.2015.01.013.
- W. Y. Zhang, X. J. Liu, H. Y. Zhang, C. C. Feng, C. X. Liu, M. M. Yu, L. H. Wei and Z. X. Li, J. Mater. Chem. C, 2015, 3, 8248–8254, 10.1039/c5tc01363a.
- D. Kand, P. S. Mandal, A. Datar and P. Talukdar, Dyes Pigm., 2014, 106, 25–31, DOI:10.1016/j.dyepig.2014.02.001.
- W. Sun, W. Li, J. Li, J. Zhang, L. Du and M. Li, Tetrahedron Lett., 2012, 53, 2332–2335, DOI:10.1016/j.tetlet.2012.02.098.
- Z. Wang, D. M. Han, W. P. Jia, Q. Z. Zhou and W. P. Deng, Anal. Chem., 2012, 84, 4915–4920, DOI:10.1021/ac300512b.
- X. L. Liu, X. Y. Duan, X. J. Du and Q. H. Song, Chem.–Asian J., 2012, 7, 2696–2702, DOI:10.1002/asia.201200594.
- X. M. Shao, R. X. Kang, Y. L. Zhang, Z. T. Huang, F. F. Peng, J. Zhang, Y. Wang, F. C. Pan, W. J. Zhang and W. L. Zhao, Anal. Chem., 2015, 87, 399–405, DOI:10.1021/ac5028947.
- Y. K. Yue, F. J. Huo, Y. B. Zhang, J. B. Chao, R. Martinez-Manez and C. X. Yin, Anal. Chem., 2016, 88, 10499–10503, DOI:0.1021/acs.analchem.6b02520.
- W. Lv, Y. Chen, L. Bian and X. Chen, Talanta, 2019, 197, 204–210, DOI:10.1016/j.talanta.2019.01.022.
- M. J. Frisch, G. W. Trucks, G. B. Schlegelet al., Gaussian 09, Revision A.1, Gaussian, Inc., Wallingford CT, 2009 Search PubMed.
- C. Adamo, G. E. Scuseria and V. Barone, J. Chem. Phys., 1999, 111, 2889–2899, DOI:10.1063/1.479571.
- C. Guido and S. Caprasecca, https://www1.dcci.unipi.it/molecolab/tools/white-papers/pisalr/, 2016, DOI:10.13140/RG.2.1.1903.7845.
- P. Krawczyk, M. Pietrzak, T. Janek, B. Jędrzejewska and P. Cysewski, J. Mol. Model., 2016, 22(125), 1–11, DOI:10.1007/s00894-016-2990-4.
- P. Krawczyk, B. JędrzejewskA, M. Pietrzak and T. Janek, J. Photochem. Photobiol., B, 2016, 164, 112–122, DOI:10.1016/j.jphotobiol.2016.07.044.
- P. Krawczyk, B. Jędrzejewska, M. Pietrzak and T. Janek, J. Photochem. Photobiol., B, 2017, 166, 74–85, DOI:10.1016/j.jphotobiol.2016.11.008.
- P. Krawczyk, B. Jędrzejewska, P. Cysewski and T. Janek, Org. Biomol. Chem., 2017, 15, 8952–8966, 10.1039/c7ob02439h.
- N. Minezawa, Chem. Phys. Lett., 2014, 608, 140–144, DOI:10.1016/j.cplett.2014.05.104.
- G. S. Ming TonG, K. T. Chan, X. Chang and Ch.-M. Che, Chem. Sci., 2015, 6, 3026–3037, 10.1039/c4sc03697b.
- C. Guido and S. Caprasecca, https://www1.dcci.unipi.it/molecolab/tools/white-papers/pisalr/, DOI:10.13140/RG.2.1.1903.7845.
- L. V. Slipchenko, J. Phys. Chem. A, 2010, 114, 8824–8830, DOI:10.1021/jp101797a.
- K. Sneskov, T. Schwabe, O. Christiansena and J. Kongsted, Phys. Chem. Chem. Phys., 2011, 13, 18551–18560, 10.1039/C1CP22067E.
- M. Caricato, J. Chem. Phys., 2013, 139, 044116, DOI:10.1063/1.4816482.
- T. Le Bahers, C. Adamo and I. Ciofini, J. Chem. Theory Comput., 2011, 7, 2498–2506, DOI:10.1021/ct200308m.
- M. T. Cancés, B. Mennucci and J. Tomasi, J. Chem. Phys., 1997, 107, 3032–3041, DOI:10.1063/1.474659.
- M. Arivazhagan, P. Muniappan, R. Meenakshi and G. Rajavel, Spectrochim. Acta, Part A, 2013, 105, 497–508, DOI:10.1016/j.saa.2012.11.033.
- R. W. Boyd, in Nonlinear Optics, Academic, London, 2nd edn, 2003, p. 521 Search PubMed.
- D. P. Craig and T. Thirunamachandran, Molecular quantum electrodynamics: an introduction to radiation-molecule interaction, Dover Publications, Inc., Mineola, New York, 1st edn, 1998, ch. 5 Search PubMed.
- K. Ohta, L. Antonov, S. Yamada and K. Kamada, J. Chem. Phys., 2007, 127, 084504–084515, DOI:10.1063/1.2753490.
- R. Zaleśny, W. Bartkowiak, S. Styrcz and J. Leszczynski, J. Phys. Chem. A, 2002, 106, 4032–4037, DOI:10.1021/jp0142684.
- J. Olsen and P. Jorgensen, J. Chem. Phys., 1985, 82, 3235, DOI:10.1063/1.448223.
- P. Sałek, O. Vahtras, J. D. Guo, Y. Luo, T. Helgaker and H. Ågren, Chem. Phys. Lett., 2003, 374, 446–452, DOI:.
- DALTON, a molecular electronic structure program, Release Dalton 2011, 2011, see http://daltonprogram.org/ Search PubMed.
- LSDALTON, a linear scaling molecular electronic structure program, Release Dalton 2011, 2011, see http://daltonprogram.org Search PubMed.
- V. Potemkin and M. Grishina, Drug Discovery Today, 2008, 13(21–22), 952–959, DOI:10.1016/j.drudis.2008.07.006.
- V. Potemkin and M. Grishina, J. Comput.-Aided Mol. Des., 2008, 22(6–7), 489–505, DOI:10.1007/s10822-008-9203-x.
- V. Potemkin, A. A. Pogrebnoy and M. A. Grishina, J. Chem. Inf. Model., 2009, 49(6), 1389–1406, DOI:10.1021/ci800405n.
- O. P. Chzhu, D. E. Araviashvili and I. G. Danilova, Emerg. Sci. J., 2020, 4, 37–43, DOI:10.28991/esj-2020-01208.
- K. Z. W. Lae, S. S. Su, N. N. Win, N. N. Than and H. Ngwe, SciMedicine Journal, 2019, 4, 199–216, DOI:10.28991/scimedj-2019-0104-5.
- S. Cosconati, S. Forli, A. L. Perryman, R. Harris, D. S. Goodsell and A. J. Olson, Expert Opin. Drug Discovery, 2010, 5, 597–607, DOI:10.1517/17460441.2010.484460.
- S. Forli and A. J. Olson, J. Med. Chem., 2012, 55, 623–638, DOI:10.1021/jm2005145.
- O. Trott and A. J. Olson, J. Comput. Chem., 2010, 31, 455–461, DOI:10.1002/jcc.21334.
- C. S. Panjikar, P. A. Tucker and M. S. Weiss, Acta Crystallogr., Sect. D: Biol. Crystallogr., 2005, 61, 1263–1272, DOI:10.2210/pdb2a7e/pdb.
- V. Potemkin and M. Grishina, J. Comput.-Aided Mol. Des., 2008, 22(6–7), 489–505, DOI:10.1007/s10822-008-9203-x.
Footnote |
† Electronic supplementary information (ESI) available: Spectroscopic properties and biological activities for investigated compounds. See DOI: 10.1039/d0ra04835f |
|
This journal is © The Royal Society of Chemistry 2020 |