DOI:
10.1039/D0RA04793G
(Paper)
RSC Adv., 2020,
10, 31740-31747
Development of a new bisphenol A electrochemical sensor based on a cadmium(II) porphyrin modified carbon paste electrode
Received
31st May 2020
, Accepted 4th August 2020
First published on 26th August 2020
Abstract
In this study, the (5,10,15,20-tetrakis[(4-methoxyphenyl)]porphyrinato)cadmium(II) complex ([Cd(TMPP)]) was successfully used as a modifier in a carbon paste electrode (CPE) and exploited for bisphenol A (BPA) detection. Analytical performance revealed two linear ranges from 0.0015–15 μM and 0.015–1.5 mM with a detection limit of 13.5 pM. The proposed method was implemented in water samples, which resulted in quantitative signals over the range 6.5–1000 μM with recoveries between 92.6 and 107.7% for tap water and between 96.6 to 106.0% for mineral water.
1. Introduction
Bisphenol A (BPA) is a contaminant of environmental concern and an important component in the production of plastics. It has been widely used as an additive in the production of epoxy resins and polycarbonate substances1,2 which are widely used in food and beverage containers3,4 due to their transparent, strong and light characteristics.5 There is evidence that BPA can act as an endocrine disruptor, and its toxicity6 has been widely reported in the literature, with an LD50 of approximately 3250 mg per kg body weight [bw] per day,7,8 mainly based on body weight changes in two- and three-generation studies in mice and rats. Studies have shown that even low levels of BPA mimic and interfere with hormonal activity by interfering with growth and reproductive development.9 It may be associated with several types of cancer (e.g. testicular, prostate, and breast cancer).10,11 There is concern that the species can migrate from packaging into a wide range of food matrices; from water storage tanks to drinking water for instance. In addition, BPA contamination in the environment has created significant risks to ecosystems during production and recycling. Therefore, due to the harmful effects of BPA on the environment and the health of humans, it is of great importance to control its levels in environmental, biological, and food samples.12 Hence, a rapid, specific and sensitive analytical method for BPA detection is required in the fields of environmental monitoring, food safety, and toxicity evaluation.
It is noteworthy that several analytical methods are currently used to detect BPA, including liquid chromatography-mass spectrometry, electrochemiluminescence, colorimetry, liquid chromatography coupled to UV/vis, fluorescence, enzyme-linked immunosorbent assay (ELISA) and surface-enhanced Raman scattering (SERS)13–15 However, they have many drawbacks, all these methods need complicated pre-treatment due to requirements for the extraction and purification of the sample, they are expensive, complicated and time-consuming, thus restricting their application.16 These methods do not allow fast processing of multiple samples and real-time detection. Therefore, researchers have turned their attention to the use of electrochemical sensors as an alternative solution for BPA quantitation due to their inherent advantages.
Electrochemical sensing lends itself to rapid on-site BPA detection at different electrode materials, including carbon17,18 and metallic transducers.19,20 However, electrode fouling which occurs during the electrooxidation of phenols is a challenge.21–24 It has been found that the most common way to deal with electrode fouling problems is to modify the electrode surface with e.g. composite electrocatalytic materials containing nanoparticles.24–26 As a result, a time-consuming, re-modification step (such as drop-dry or dip-coating method) is usually recommended for the regeneration of the modified electrode for every new measurement.
Carbon Paste Electrodes (CPE), which involve a coupling of pure graphite powder and liquid binder, was initially reported in 1958 by Adams,27 and this type of electrode offers surface renewability, a stable response, high versatility, low cost, low background currents and ease of modification.28–30 The addition of electroactive materials into the paste is advantageous and has been extensively applied in the electroanalytical community. One of the most important roles of modifiers is to reduce the redox potential required for electrochemical reactions and to increase the sensitivity and selectivity of the assay.31 Many materials involving metals or metal alloys, various nanotubes and nanoparticles, graphene and fullerenes, porphyrins and their derivatives, and some organic dyes have been widely used to construct electrochemical sensors.32,33
Amongst such materials, porphyrins and their derivatives have been used as excellent electron donors in recent years and have received extensive attention. Indeed, porphyrins are naturally occurring macrocyclic species that bind metals via N donor atoms on four pyrrole subunits, resulting in versatile chelating systems. Synthetic metalloporphyrins have been investigated as models for hemoproteins and enzymes such as hemoglobin, myoglobin, and cytochromes. In recent decades, researchers have extended the use of porphyrin-based compounds known as porphyrinoids (as free bases or metallated) where they are involved in several areas of chemistry, biochemistry, medicine, and physics. Actually, these applications include catalysis, photodynamic therapy, photodynamic destructions of viruses, semiconductors, nonlinear optics, photovoltaic materials, and chemical sensors.34–36
It is noteworthy that the cadmium ions as well as the Zinc IIB group metal are unambiguously in the +2 oxidation state in porphyrin derivatives. The direct metalation of porphyrin with zinc or cadmium leads to the tetracoordinated porphyrin species type [MII(Porph)] (Porph = porphyrinato and M = Zn or Cd). The [Zn(Porph)] species are very reactive to yield pentacoordinated and hexacoordinated complexes type [Zn(Porph)(L)x] (L = neutral monodentate axial ligand, x = 1 or 2) while [Cd(Porph)] complexes are less reactive than [Zn(porph)] and only few pentacoordinated [Cd(porph)(L)] species are reported.37 This is the major reason why we choose this latter species for our investigation because this Cd(II) coordination compound is inert compared to other metalloporphyrins such as [Zn(Porph)]. On the other hand, very few [Cd(Porph)] complexes are reported in the literature and the only reported Cd-tetravalent porphyrin species is the [Cd(TPP)] complex where TPP is the (5,10,15,20-tetraphenyl)porphyrinato.38 Up to date, there are no reported investigations using a tetracoordinated cadmium(II) complex with the (5,10,15,20-tetrakis)[(4-methoxyphenyl)] porphyrin (TMPP).
In this paper, a carbon paste electrode (CPE) was modified with the (5,10,15,20-tetrakis[(4-metoxyphenyl)]porphyrinato)cadmium(II), complex [Cd(TMPP)], to obtain a novel electrochemical sensor CPE–[Cd(TMPP)] for the determination of bisphenol A (BPA). The [Cd(TMPP)] species were prepared and characterised by elemental analysis, FT-IR, UV/vis and 1H-NMR and the prepared CPE–[Cd(TMPP)] electrode was characterized using cyclic voltammetry (CV). The parameters that influence the electrochemical reaction i.e. pH, scan rate and interferences were examined electrochemically. The prepared sensor showed two linear ranges from 1.5–15
000 nM and 0.015–1.5 mM with a 3.75 × 10−11 M limit of detection (LOD, S/N = 3). The percentage recoveries for two tested water samples (tap water and mineral water) were >92%, which shows the usefulness of this new sensor for the electrochemical detection of BPA in drinking water samples.
2. Experimental
2.1. Chemicals and apparatus
Bisphenol A (≥99.0%), Nujol oil, graphite powder (fine powder pure pH 5–6 (50 g l−1, H2O, 20 °C) and solvents were purchased from Sigma-Aldrich. All chemicals were of analytical grade and used without any further purification. Phosphate buffer was used as a supporting electrolyte and prepared with appropriate amounts of 0.1 M KCl and 0.1 M KH2PO4. A glassy carbon electrode (GCE) was used for electrochemical characterization studies. Working solutions were freshly prepared before use. The 5,10,15,20-tetrakis[(4-metoxyphenyl)]porphyrin) (H2TMPP) was prepared according to the standard literature method.39 The 1H NMR spectra were recorded on a Bruker Advance 300 MHz spectrometer. Infrared spectra were obtained with a Shimadzu FTIR-8400 spectrophotometer in the 4000–400 cm−1 region. UV/Vis spectra were recorded with a WinAspect PLUS (validation for SPECORD PLUS version 4.2) scanning spectrophotometer using dichloromethane solutions in 1.0 cm path length cuvette. Mass analysis was performed by LC-MS spectrometer 2020 (Shimadzu, Japan) through electron-spray ionization (ESI) technique.
2.2. Preparation of the [Cd(TMPP)] complex
The (5,10,15,20-tetrakis[(4-metoxyphenyl)]porphyrinato)cadmium(II) ([Cd(TMPP)]) was synthesized according to the literature.40 H2TMPP (300 mg, 0.42 mmol) and CdCl2·2H2O (1 g, 1.344 mmol) were refluxed in DMF for 5 h. The color of the solution rapidly changed from purple to dark green (Scheme 1) and the reaction was monitored by UV/vis spectroscopy. The reaction mixture was then filtered, and the solvent was removed under reduced pressure to afford 0.45 g (yield ∼80%) of [Cd(TMPP)] as dark blue solid. CHN analysis (calculated) C48H36CdN4O4 (845.25 g mol−1) C, 68.20; H, 4.29; N, 6.63%. Found: C, 68.36; H, 4.32; N, 6.74%. UV/vis [CH2Cl2: λmax in nm (log
ε)]: 437(4.95), 573(3.65), 617(3.66). IR [(solid, cm−1)]: ν(CH).: 2986–2836, ν(O–CH3): 1246, (C
N): 1507, ν(C
C): 1601, ν(CN): 1329, δ(CCH): 999. MS-ESI (CH2Cl2): (m/z) = 845.5 [Cd(TMPP)]+. 1H NMR (300 MHz, CDCl3, 298 K): δ(ppm) 8.86 (s, 8H, Hβ-pyrr.), 8.14 (s, 8H, H-ortho), 8.11 (s, 8H, H-meta), 4.10 (s, 12H, O__CH3).
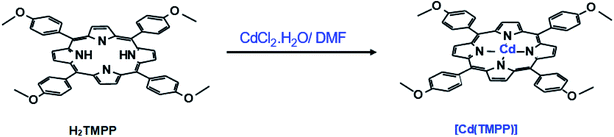 |
| Scheme 1 Synthesis of the [Cd(TMPP)] complex. | |
2.3. Preparation of the electrodes
The CPEs were prepared according to reported procedures.41,43,44 Graphite powder and the Nujol oil were thoroughly mixed in a 7
:
3 (w/w) ratio in a mortar to form a very fine homogeneous paste. The paste was then packed into a glass tube (2 mm diameter) with electrical contact via a copper wire. In the case of the modified CPE–[Cd(TMPP)] sensor, an optimised amount of [Cd(TMPP)] was added to the graphite/oil mixture and mixed to form a well dispersed and homogeneous paste.
3. Results and discussion
3.1. Characterisation of [Cd(TMPP)]
3.1.2. 1H NMR spectra. The proton NMR spectrum of the [Cd(TMPP)] complex is shown in Fig. 3. The phenyl protons (Ho, Ho′ and Hm, Hm′) resonate in the 7.50–8.50 ppm range and the peaks corresponding to the β-pyrrolic protons appear at 8.86 ppm. These chemical shift values are very close to those of the free base H2TMPP porphyrin indicating a diamagnetic character of our Cd(II)–porphyrin derivative.39 The absence of the peak corresponding to the N–H protons of the pyrroles, shown at −2.74 ppm for the free base porphyrin indicates the insertion of the Cd(II) cation in the cavity of the H2TMPP porphyrin.
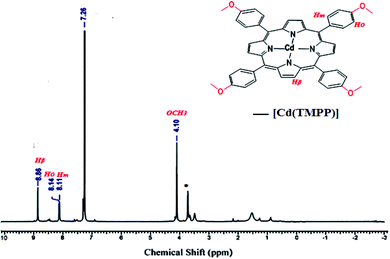 |
| Fig. 3 1H NMR spectrum (300 MHz) of [Cd(TMPP)] recorded in CDCl3. | |
3.1.3. Cyclic voltammetry behavior of [Cd(TMPP)] at GCE. The electrochemical behavior of [Cd(TMPP)] was studied by CV in tetrahydrofuran (THF) solvent using tetrabutylammonium tetrafluoroborate ((n-Bu)4NBF4) as supporting electrolyte (0.1 M). Fig. 4(A) presents the cyclic voltammograms of [Cd(TMPP)] and the background n-Bu4NBF4 supporting electrolyte recorded in THF at a glassy carbon electrode. Fig. 4(A) shows 3 oxidation waves (Ep at 0.63, 0.25 and −0.55 V vs. Ag/Ag+) with corresponding cathodic processes (Ep at 0.125 V, −0.55 V and −1.0 V Ag/Ag+), representing the central metal possibly Cd(0/II) re-oxidation at −0.55 V (O3/R3) and the π conjugated ring system of the porphyrin ligand (O1/R1, O2/R2) with contribution from methoxy electron-donating groups.43–45.
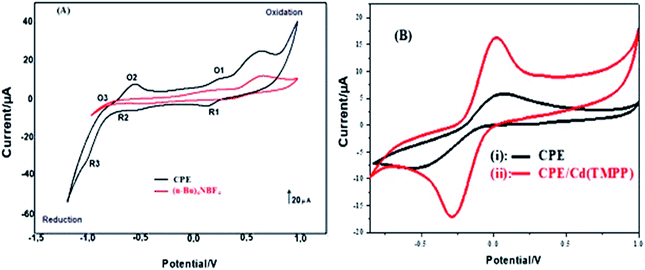 |
| Fig. 4 (A) Cyclic voltammograms of 1 mM [Cd(TMPP)] (black) dissolved in THF with background electrolyte 0.1 M ((n-Bu)4NBF4) (red), scan rate: 100 mVs−1 at a GCE with non-aqueous reference electrode. (B) cyclic voltammograms of (i) CPE and (ii) CPE–[Cd(TMPP)] in 5 mM Fe(CN)63−/4− solution containing 0.1 M KCl. Scan rate of 100 mVs−1. | |
3.1.4. Electrochemical characterisation of CPE–[Cd(TMPP)]. Fig. 4(B) shows the redox behavior of the [Cd(TMPP)] modified CPE using an anionic probe – K3[Fe(CN)6] over the potential range −0.9 to 1 V vs. Ag/AgCl at 100 mVs−1. Fig. 4(B) curve (i) shows a cyclic voltammogram at the unmodified CPE in the presence of 5 mM K3[Fe(CN)6] resulting in a pair of well-defined voltammetric peaks with cathodic peak potential (Epc) at 0.072 V and anodic peak potential (Epa) at −0.286 V with peak-to-peak separation (ΔEp) 267 mV vs. Ag/AgCl. Curve (ii) represents the CPE–[Cd(TMPP)] response in the same solution (Epc of 0.019 V, Epa −0.562 V ΔEp 490 mV). Compared with the bare CPE, the peak currents of the [Cd(TMPP)] modified electrode decreased dramatically with increased ΔEp indicating hindered electron transfer for the Fe(CN)63−/4− species as a result of the porphyrin modifier.
3.2. Optimization of experimental conditions
3.2.1. % [Cd(TMPP)] loading in carbon paste. The purpose of this work was to fabricate a simple, low cost and a highly sensitive electrochemical sensor for the measurement of BPA. In order to obtain the best electrode configuration, different quantities of [Cd(TMPP)] (0–7% w/w) were added to the carbon paste electrode. The effect on the voltammetric response to 1.5 mM BPA (dissolved in 0.1 M phosphate buffer/acetonitrile 20% (v/v)) was studied. The inspection of Fig. 5 shows that the sensor response increases with an increasing amount of [Cd(TMPP)] modifier up to 3%. Then, the signal decreases significantly which could be due to the presence of more adsorption sites at higher % loadings leading to the accumulation of oxidation products.46
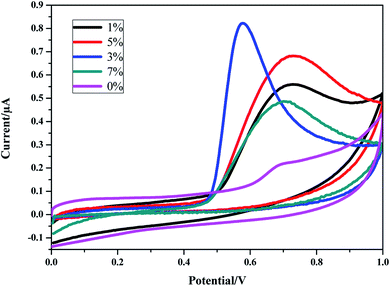 |
| Fig. 5 Response to 1.5 mM BPA for CPE–[Cd(TMPP)] modified electrode over the range 0–7% w/w. Electrolyte concentration of 0.1 M phosphate buffer/20% acetonitrile (v/v) (pH 7 at 100 mV s−1). | |
3.2.2. pH effect. The effect of electrolyte pH on the response of the CPE–[Cd(TMPP)] based sensor was studied by CV over the pH range 4–8. It was found that the intensity of the oxidation peak gradually increases with increasing pH (Fig. 6(A)) where a pH of 7 resulted in the optimal signal. The anodic peak potentials appeared to be strongly dependent on solution pH and the oxidation potential (Epa) shifted negatively with increasing pH in a linear relationship indicating that protons are directly involved in the oxidation of BPA (Fig. 6(B)). This relationship obeys the following equation: Epa = −0.0586pH + 0.7648; (correlation coefficient: R2 = 0.9084). A slope of 55 mV pH−1, which is close to the theoretical value of 0.0576 V pH−1, clearly indicates that equal numbers of electrons and protons were involved in charge transfer.
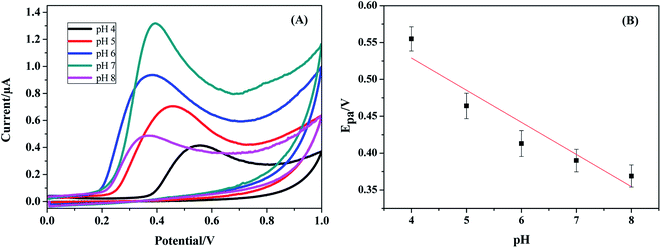 |
| Fig. 6 (A) Cyclic voltammograms of 1.5 mM BPA at CPE–[Cd(TMPP)] (3% loading w/w) over pH 4–8. (B) plot of Epa vs. pH. | |
3.2.3. Scan rate. In order to understand the electrocatalytic mechanism of BPA at the CPE–[Cd(TMPP)] electrochemical sensor, the effect of scan rate (v) on the oxidation current of bisphenol A was examined (Fig. 7(A)). The peak currents (Ip) increased linearly with the scan rate (v) in the [20–300] mV s−1 range, indicating an electrochemical process under adsorption control where the linear regression equation can be estimated by: Ipa (μA) = 0.061 + 0.0042 V (with a correlation coefficient R2 = 0.96) (Fig. 7(B)). Meanwhile, a linear relationship between Epa and lnv was also observed over the 20–300 mV s−1 range. The equation can be expressed as: Epa (V) = −0.113
ln
v + 0.611 (with a correlation coefficient R2 = 0.9) (Fig. 7(C)).
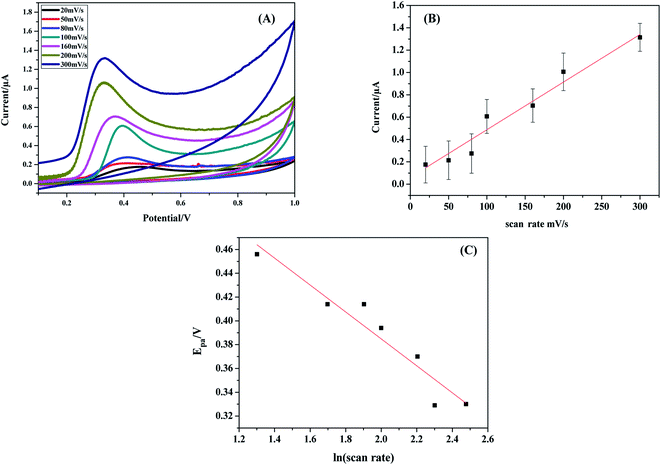 |
| Fig. 7 (A) Cyclic voltammograms of 1.5 mM BPA at CPE–[Cd(TMPP)] over the range 20–300 mV s−1 (B) Ipa vs. scan rate, (C) Epa vs. ln(scan rate). | |
As for a surface controlled and totally irreversible electrode process, Epa is defined by the Laviron equation:47
where
α is the transfer coefficient,
ko is the standard rate constant of the reaction,
n is the electron transfer number involved in rate determining step,
v is the scan rate,
Eo is the formal redox potential,
R is the gas constant,
T is the absolute temperature, and
F is Faraday's constant. According to the linear correlation of
Epa vs. ln
v,
αn was found to be 1.1 from the slope of the plot. Assuming α to be 0.5,
48,49 the number of the electrons included in the electrochemical oxidation of BPA was found to be 2. According to previous reports with regards to the oxidation of BPA and other phenolic compounds and the results obtained from this work, the electrooxidation process of BPA at the modified electrode is as shown by
Scheme 2. The 4 fold enhancement in current in the presence of 3%
[Cd(TMPP)] indicates an electrocatalytic role for the porphyrin modifier. The Cd metal centre and associated redox process may play a role in accelerating the electrooxidation of the BPA molecule, making for more efficient electron and proton transfer with the associated lowering of BPA overpotential (from 0.7 to 0.6 V
vs. Ag/AgCl). The enhancement of the available surface area results in the increase of the oxidation current and the modifier can also act to minimize surface fouling from the oxidation products.
 |
| Scheme 2 Electrooxidation process of BPA at the CPE–[Cd(TMPP)]. | |
3.2.5. Interference study. The selectivity of the electrochemical sensor was also examined in phosphate buffer (pH = 7). Some phenolic species and common inorganic ions have been tested to check their levels of interference in BPA determination in the presence of 1.5 μM BPA (Table 2). In addition, inorganic ions, such as Ca+, K+, Zn2+ and Na+ at 100-fold concentrations were shown to have no influence on BPA signals. These results indicated that CPE–[Cd(TMPP)] electrode exhibits selective reactivity to BPA in the presence of these ionic species.
Table 2 Influence of interferents, 100/50-fold concentration on the electrode response of 1.5 μM BPA at 0.42 V vs. Ag/AgCl
|
Interferents |
Peak change (%) |
50-Fold |
Hydroquinoe |
−3.28 |
Phenol |
+2.54 |
4-Octylphenol |
+4.89 |
Pyrocatechol |
−3.78 |
Dopamine |
+2.96 |
Ascorbic acid |
+2.15 |
100-Fold |
Ca+ |
−4.52 |
K+ |
−1.56 |
Zn2+ |
−3.12 |
Na+ |
−3.21 |
3.3. Real sample analysis
In order to confirm the sensitivity and applicability of the proposed method, the fabricated [Cd(TMPP)]/CPE sensor was used to determine BPA in water samples (tap and mineral water). Under the optimized conditions, a known-amount of the sample was added into pH 7 phosphate buffer and then analyzed according to the above-described procedures. No BPA species were detected in the water samples (Table 3). The same samples were then spiked with different amounts of BPA and the recoveries were in the range 92.6–107.7% and 96.6–106% for tap and mineral water respectively, indicating that the sensor is perfectly adequate for application in natural samples.
Table 3 BPA detection in real drinking water samples
Sample |
Added (M) |
Found (M) |
Recovery% |
RSD% (n = 3) |
Tap water |
1.5 × 10−3 |
1.39 × 10−3 |
92.6 |
1.24 |
1.5 × 10−4 |
1.61 × 10−4 |
107.7 |
2.35 |
1.5 × 10−5 |
1.52 × 10−5 |
101.5 |
3.52 |
1.5 × 10−6 |
1.47 × 10−6 |
98.3 |
2.82 |
1.5 × 10−7 |
1.46 × 10−7 |
97.5 |
1.09 |
Mineral water |
1.5 × 10−3 |
1.45 × 10−3 |
96.6 |
1.34 |
1.5 × 10−4 |
1.56 × 10−4 |
104 |
2.11 |
1.5 × 10−5 |
1.49 × 10−5 |
99.3 |
1.65 |
1.5 × 10−6 |
1.57 × 10−6 |
104.6 |
1.9 |
1.5 × 10−7 |
1.55 × 10−7 |
103.3 |
1.56 |
1.5 × 10−8 |
1.59 × 10−8 |
106 |
1.15 |
4. Conclusions
This article reports the fabrication of a simple, cost-effective, and highly sensitive sensor for BPA based on a carbon paste matrix encapsulating a new cadmium(II) porphyrin derivative. The synthesis, the UV/vis, IR and proton NMR characterisation of the (5,10,15,20-tetrakis[(4-metoxyphenyl)]porphyrin)cadmium(II) complex ([Cd(TMPP)]) was reported. The use of the porphyrin compound as a modifier in a carbon paste electrode improved the analytical performance compared to that exhibited by glassy carbon modified with other nanomaterials. The results show that the bisphenol A oxidation signal was enhanced ad the modified electrode proved to be highly sensitive, with acceptable stability. Two linear ranges were observed from 0.0015–15 μM and 0.015–1.5 mM with a detection limit of 13.5 pM. Quantitation of BPA was enabled at this surface and analytical performance was excellent in spiked drinking water samples.
Conflicts of interest
There are no conflicts of interest to declare.
Acknowledgements
This work was supported by the Tunisian Ministry of Higher Education and Scientific Research.
References
- A. Bhatnagar and I. Anastopoulos, Chemosphere, 2017, 168, 885–902 CrossRef CAS PubMed.
- J. I. Kwak, J. Moon, D. Kim, R. Cui and Y.-J. An, J. Hazard. Mater., 2018, 344, 390–397 CrossRef CAS PubMed.
- L. A. Goulart, R. Gonçalves, A. A. Correa, E. C. Pereira and L. H. Mascaro, Microchim. Acta, 2017, 188, 1–9 Search PubMed.
- X. Zhu, G. Wu, Y. Xing, C. Wang, X. Yuan and B. Li, J. Hazard. Mater., 2020, 381, 120908 CrossRef CAS PubMed.
- H. Filik and A. A. Avan, Curr. Anal. Chem., 2017, 13, 464–473 CAS.
- H. Li, W. Wang, Q. Lv, G. Xi, H. Bai and Q. Zhang, Electrochem. Commun., 2016, 68, 104–107 CrossRef CAS.
- R. E. Chapin, J. Adams, K. Boekelheide, L. E. Gray Jr, S. W. Hayward, P. S. Lee, B. S. McIntyre, K. M. Portier, T. M. Schnorr, S. G. Selevan, J. G. Vandenbergh and S. R. Woskie, Birth Defects Res., B, 2008, 83, 157–395 CrossRef CAS PubMed.
- L. N. Vandenberg, M. V. Maffini, C. Sonnenschein, B. S. Rubin and A. M. Soto, Endocr. Rev., 2009, 30, 75–95 CrossRef CAS PubMed.
- Y. Qiao, J. Li, H. Li, H. Fang, D. Fan and W. Wang, Biosens. Bioelectron., 2016, 86, 315–324 CrossRef CAS PubMed.
- W. Wang, X. Wang, J. Xing, Q. Gong, H. Wang, J. Wang, Z. Chen, Y. Ai and X. Wang, Environ. Sci. Nano., 2019, 6, 809–819 RSC.
- N. J. Cabaton, P. R. Wadia, B. S. Rubin, D. Zalko, C. M. Schaeberle, M. H. Askenase, J. L. Gadbois, A. P. Tharp, G. S. Whitt, C. Sonnenschein and A. M. Soto, Environ. Health Perspect., 2011, 119, 547–552 CrossRef CAS PubMed.
- N. Caballero-Casero, L. Lunar and S. Rubio, Anal. Chim. Acta, 2016, 908, 22–53 CrossRef CAS PubMed.
- E. Ferrer, E. Santoni, S. Vittori, G. Font, J. Mañes and G. Sagratini, Food Chem., 2011, 126, 360–367 CrossRef CAS.
- E. Chung, J. Jeon, J. Yu, C. Lee and J. Choo, Biosens. Bioelectron., 2015, 64, 560–565 CrossRef CAS PubMed.
- C. Peng, N. Pan, Z. Xie, L. Liu, J. Xiang and C. Liu, Anal. Lett., 2016, 49, 1492–1501 CrossRef CAS.
- K. V. Ragavan, N. K. Rastogi and M. S. Thakur, Trends Anal. Chem., 2013, 52, 248–260 CrossRef CAS.
- X. Niu, W. Yang, G. Wang, J. Ren, H. Guo and J. Gao, Electrochim. Acta, 2013, 98, 167–175 CrossRef CAS.
- Y. Li, H. Wang, B. Yan and H. Zhang, J. Electroanal. Chem., 2017, 805, 39–46 CrossRef CAS.
- K. Shim, J. Kim, M. Shahabuddin, Y. Yamauchi, Md. S. A. Hossain and J. H. Kim, Sens. Actuator B. Chem., 2018, 255, 2800–2808 CrossRef CAS.
- J. Yang, S.-E. Kim, M. Cho, I.-K. Yoo, W.-S. Choe and Y. Lee, Biosens. Bioelectron., 2014, 61, 38–44 CrossRef CAS PubMed.
- A. Ghanam, A. A. Lahcen and A. Amine, J. Electroanal. Chem., 2017, 789, 58–66 CrossRef CAS.
- M. Masikini, T. T. Waryo, P. G. L. Baker, L. V. Ngqongwa, A. R. Williams and E. I. Iwuoha, Anal. Lett., 2011, 44, 2047–2060 CrossRef CAS.
- S. Poorahong, C. Thammakhet, P. Thavarungkul, W. Limbut, A. Numnuam and P. Kanatharana, Microchim. Acta, 2012, 176, 91–99 CrossRef CAS.
- Z. Zheng, Y. Du, Z. Wang, Q. Feng and C. Wang, Analyst, 2013, 138, 693–701 RSC.
- J. Zhang, X. Xu and Z. Chen, Ionics, 2018, 24, 2123–2134 CrossRef CAS.
- R. Wannapob, P. Thavarungkul, S. Dawan, A. Numnuam, W. Limbut and P. Kanatharana, Electroanalysis, 2016, 28, 1–10 CrossRef.
- R. N. Adams, Anal. Chem., 1958, 30, 1576 CrossRef CAS.
- F. Hosseini, M. Ebrahimi and H. Karimi-Maleh, Int. J. Electrochem. Sci., 2018, 13, 4923–4932 CrossRef CAS.
- N. Soltani, N. Tavakkoli, Z. S. Mosavimanesh and F. Davar, C. R. Chim., 2018, 21, 54–60 CrossRef CAS.
- M. Kumar, B. E. K. Swamy, M. H. M. Asif and C. C. Viswanath, Appl. Surf. Sci., 2017, 399, 411–419 CrossRef CAS.
- S. Shahrokhian, A. Hamzehloei, A. Thaghani and S. R. Mousavi, Electroanalysis, 2004, 16, 915–921 CrossRef CAS.
- Y. X. Lin, Q. Zhou, Y. P. Lin, D. P. Tang, R. Niessner and D. Knopp, Anal. Chem., 2015, 87, 8531–8540 CrossRef CAS PubMed.
- M. Cui, J. D. Huang, Y. Wang, Y. M. Wu and X. L. Luo, Biosens. Bioelectron., 2015, 68, 563–569 CrossRef CAS PubMed.
- Z.-B. Liu, Y.-F. Xu, X.-Y. Zhang, X.-L. Zhang, Y.-S. Chen and J.-G. Tian, J. Phys. Chem., B, 2009, 113, 9681–9686 CrossRef CAS PubMed.
- Z. L. Dunn, M. A. Hammer, B. J. Topham and T. M. Perrine, J. Phys. Chem., C, 2017, 121, 12018–12024 CrossRef CAS.
- S. Ishihara, J. Labuta, W. V. Rossom, D. Ishikawa, K. Minami, J. P. Hill and K. Ariga, Phys. Chem. Chem. Phys., 2014, 16, 9713–9746 RSC.
- H. Toumi, N. Amiri, M. S. Belkhiria, J.-C. Daran and H. Nasri, Acta Crystallogr., Sect. E: Struct. Rep. Online, 2012, 68, 1557–1558 CrossRef PubMed.
- M. P. Byrn, C. J. Curtis, I. Goldberg, Y. Hsiou, S. I. Khan, P. A. Sawin, S. K. Tendick and C. E. Strouse, J. Am. Chem. Soc., 1991, 113, 6549–6557 CrossRef CAS.
- P. F. Rodesiler, E. A. H. Griffith, N. G. Charles, L. Lebioda and E. L. Amma, Inorg. Chem., 1985, 24, 4595–4600 CrossRef CAS.
- X. Yu, Y. Chen, L. Chang, L. Zhou, F. Tang and X. Wu, Sens. Actuator B. Chem., 2013, 186, 648–656 CrossRef CAS.
- L. Ben Haj Hassen, K. Ezzayani, Y. Rousselin, C. Stern, H. Nasri and C. E. Schulz, J. Mol. Struct., 2016, 1110, 138–142 CrossRef CAS.
- C. Mchiri, S. Dhifaoui, K. Ezzayani, M. Guergueb, T. Roisnel, F. Loiseauc and H. Nasri, Polyhedron, 2019, 171, 10–19 CrossRef CAS.
- G. Simonneaux, V. Schunemann, C. Morice, L. Carel, L. Toupet, H. Winkler, A. X. Trautwein and F. A. Walker, J. Am. Chem. Soc., 2000, 122, 4366–4377 CrossRef CAS.
- A. Ghosh, I. Halvorsen, H. J. Nilsen, E. Steene, T. Wondimagegn, R. Lie, E. Van Caemelbecke, N. Guo, Z. Ou and K. M. Kaddish, J. Phys. Chem., B, 2001, 105, 8120–8124 CrossRef CAS.
- T. H Phan and K. Wandelt, J. Chem. Phys., 2015, 142, 101917–101925 CrossRef PubMed.
- M. Siswana, K. I. Ozoemena and T. Nyokong, Talanta, 2006, 69, 1136–1142 CrossRef CAS PubMed.
- E. Laviron, J. Electroanal. Chem. Interfacial Electrochem., 1974, 52, 355–393 CrossRef CAS.
- Y. Gao, Y. Cao, D. Yang, X. Luo, Y. Tang and H. Li, J. Hazard. Mater., 2012, 199, 111–118 CrossRef PubMed.
- T. Zhan, Y. Song, X. Li and W. Hou, Mater. Sci. Eng., C, 2016, 64, 354–361 CrossRef CAS PubMed.
- Y. Li, Y. Gao, Y. Cao and H. Li, Sens. Actuators, B, 2012, 171–172, 726–733 CrossRef CAS.
- C. Hou, W. Tang, C. Zhang, Y. Wang and N. Zhu, Electrochim. Acta, 2014, 144, 324–331 CrossRef CAS.
- N. Ben Messaoud, M. E. Ghica, C. Dridi, M. B. Ali and C. M. Brett, Sens. Actuators, B, 2017, 253, 513–522 CrossRef CAS.
- A. Shrivastava and V. B. Gupta, Chron. Young Sci., 2011, 2, 21–25 CrossRef.
- W. Huang, Bull. Korean Chem. Soc., 2005, 26, 1560–1564 CrossRef CAS.
- Y. Zhang, L. T. Wang, D. B. Lu, X. Z. Shi, C. M. Wang and X. J. Duan, Electrochim. Acta, 2012, 80, 77–83 CrossRef CAS.
- M. Portaccio, D. D. Tuoro, F. Arduini, M. Lepore, D. G. Mita, N. Diano, L. Mita and D. Moscone, Biosens. Bioelectron., 2010, 25, 2003–2008 CrossRef CAS PubMed.
- Y. Wang, Y. Yang, L. Xu and J. Zhang, Electrochim. Acta, 2011, 56, 2105–2109 CrossRef CAS.
- L. Zhu, Y. Cao and G. Cao, Biosens. Bioelectron., 2014, 54, 258–261 CrossRef CAS PubMed.
- F. Wang, J. Yang and K. Wu, Anal. Chim. Acta, 2009, 638, 23–28 CrossRef CAS PubMed.
- H. Beitollahi and S. Tajik, Environ. Monit. Assess., 2015, 187, 257–267 CrossRef PubMed.
- F. Ianesko, C. A. de Lima, C. Antoniazzi, E. R. Santana, J. V. Piovesan, A. Spinelli, A. Galli and E. Guimarães de Castro, Electroanalysis, 2018, 30, 1946–1955 CrossRef CAS.
- J. Srinivas, R. J. Mascarenhas, O. D'Souza, A. K. Satpati and Z. Mekhalif, Anal. Chem. Lett., 2017, 7, 52–64 CrossRef CAS.
|
This journal is © The Royal Society of Chemistry 2020 |
Click here to see how this site uses Cookies. View our privacy policy here.