DOI:
10.1039/D0RA04705H
(Paper)
RSC Adv., 2020,
10, 33086-33102
Bioremediation of tetracycline antibiotics-contaminated soil by bioaugmentation
Received
28th May 2020
, Accepted 16th August 2020
First published on 7th September 2020
Abstract
Bioaugmentation using specific microbial strains or consortia was deemed to be a useful bioremediation technology for increasing bioremediation efficiency. The present study confirmed the effectiveness and feasibility of bioaugmentation capability of the bacterium BC immobilized on sugarcane bagasse (SCB) for degradation of tetracycline antibiotics (TCAs) in soil. It was found that an inoculation dose of 15% (v/w), 28–43 °C, slightly acidic pH (4.5–6.5), and the addition of oxytetracycline (OTC, from 80 mg kg−1 to 160 mg kg−1) favored the bioaugmentation capability of the bacterium BC, indicating its strong tolerance to high temperature, pH, and high substrate concentrations. Moreover, SCB-immobilized bacterium BC system exhibited strong tolerance to heavy metal ions, such as Pb2+ and Cd2+, and could fit into the simulated soil environment very well. In addition, the bioaugmentation and metabolism of the co-culture with various microbes was a complicated process, and was closely related to various species of bacteria. Finally, in the dual-substrate co-biodegradation system, the presence of TC at low concentrations contributed to substantial biomass growth but simultaneously led to a decline in OTC biodegradation efficiency by the SCB-immobilized bacterium BC. As the total antibiotic concentration was increased, the OTC degradation efficiency decreased gradually, while the TC degradation efficiency still exhibited a slow rise tendency. Moreover, the TC was preferentially consumed and degraded by continuous introduction of OTC into the system during the bioremediation treatment. Therefore, we propose that the SCB-immobilized bacterium BC exhibits great potential in the bioremediation of TCAs-contaminated environments.
1 Introduction
Tetracycline antibiotics (TCAs) produced by actinomycetes1 constitute a family of antibiotics that exhibit broad-spectrum antimicrobial activity against a variety of Gram-positive and Gram-negative bacteria,2 mycoplasmas, chlamydiae, protozoan parasites,3 and rickettsiae.4,5 Given their low cost,6 low toxicity,7 availability, and desirable and effective antimicrobial properties,1,5 TCAs have been extensively applied in the prevention and treatment of human and animal diseases,6,8,9 and as additives in animal foodstuffs to improve food utilization efficiency.10–12 TCAs were discovered in the 1940s, and oxytetracycline (OTC) and tetracycline (TC) have recorded the highest consumption by livestock.4 It has been demonstrated that once inside the cell, they reversibly bind to the receptors of the 30S ribosomal subunits of susceptible organisms, thereby preventing aminoacyl transfer from binding to DNA, inhibiting protein synthesis, and preventing cell growth.13–15 However, due to incomplete metabolism as well as poor adsorption in intestines of human and animals,16,17 a considerable portion of the TCAs might be excreted as parent compounds and/or their active metabolites via urine as well as feces, which then enter the environment.6,16 Because of lack of effective environmental management and timely degradation and/or elimination, inappropriate and/or abusive use of TCAs has resulted in teeth stains in children,18 damage to kidneys and hematopoietic function,19 accumulation of TCAs in foodstuff,20 presence of numerous TCA-resistant bacteria in the intestines of livestock,21 and even their residual concentrations in the environment. Large quantities of residual TCAs have been frequently detected in various environmental matrices worldwide with concentration levels from ng kg−1 to mg kg−1 such as the surface soil,9 peri-urban agricultural soil,22 agricultural soil,23 vegetable farmland soil,24 and crops.25
TCAs exhibited the highest partitioning coefficients compared to other antibiotics, which indicates that these compounds would be preferentially retained in the soil.26 Interestingly, higher initial concentrations of TCAs in soil seem to retard their degradation and prolong their persistence in soil.27 Furthermore, the TCAs in soil environment not only exert toxic effects to plants28,29 and animals26,30 but also pose high ecological risk to soil microorganisms31 and even pose a significant long-term threat to the ecosystem and human health32 due to their biotoxicity and chemical stability.33 It has been demonstrated that residual TCAs could alter the structure of microbial communities by partially inhibiting their bacterial activities,31 boosting the TCA resistance,34 and enriching the abundance of antibiotic resistance genes (ARGs).35 For example, it has been well documented that soil OTC exposure would make a significant contribution to their bioaccumulation in Enchytraeus crypticus body tissues, alter the structure of microbial communities, and enhance the abundance of antibiotic resistance genes (ARGs) in Enchytraeus crypticus gut, particularly TC-related antibiotic resistance genes (ARGs).36 Furthermore, it has been pointed out that zwitterionic TC is considered to be the most conducive for bacterial uptake and induces the expression of the antibiotic resistance genes (ARGs) most effectively.32 All these manifest that residual TCAs and TCAs-resistant bacteria in the environment pose serious and long-lasting threats to ecosystem security and public health.37,38
Soil contamination by antibiotics and their derivatives has become an unavoidable phenomenon in the environment and the efficacy of some traditional clean-up/remediation methods such as adsorption,39 photocatalytic degradation,40 and bioelectrochemical41 has been seriously questioned because of either their incompetence, high cost, generation of secondary toxic byproducts, non-sustainability, or ecological hazards.42–44 Among a variety of remediation strategies, bioremediation, which utilizes the metabolic potentials of specific microbes for degrading contaminants, has been considered as an efficient, cost-effective, and environmentally sound remediation approach and a more reliable alternative for the remediation of various contaminated sites.44–49 To date, bioremediation has been practiced in various forms such as the use of single strain microorganisms,50–52 the use of genetically engineered microorganisms,53 and the use of microbial consortium.34 So far, bioremediation has been practiced by the means of three broad methods, namely, bioaugmentation (the process refers to the application of either indigenous, allochthonous, or genetically-modified microbes that exhibit enhanced and desirable biodegradative capacities in hazardous contamination sites in order to accelerate the elimination of undesired compounds), biostimulation (the process involves the spurring of microbial growth by the addition of nutrients, supplements, electron acceptors or donors that are capable of elevating the bioavailability), finally bioattenuation (the process refers to the method that relies on natural processes to dissipate contaminants through biological transformation as well as the natural ability of indigenous microorganisms).44,54
Bioaugmentation, as one of the in situ bioremediation strategies, has been deemed to be the most effective and useful technique for improving the biodegradative capacities of contaminated sites by the introduction of specific microbial strains or consortia with enhanced and desirable biodegradative capacities.41,44,55 The inoculated microbial strains are capable of influencing the degradation performance, biomass, diversity, as well as the structure of the indigenous microbial communities.56,57 Nevertheless, sometimes, harsh environmental conditions (e.g., extreme temperature variation, highly acidic or alkaline pH, and saline conditions) and inadequate access to nutrient sources hinder the growth of the introduced biological agents by means of imposing restrictions on their physical activities.54 Moreover, the efficiency of biodegradation is usually prohibited by the low bioavailability of as well as the complex structure of the target contaminants and the harsh environment that either lacks nutrients or is toxic for the growth of the introduced biological agents.58,59
Among various bioaugmentation strategies, the immobilization of the introduced augmented microbial strains on a biocarrier is considered as one of the most promising method that exhibits potential future applications for the enhanced and rapid biological treatment of various contaminants.54 The immobilized process could offer substantial advantages in terms of the enhanced cell permeability, enhanced surface area, high biological density, increased microbial stability, environmental sustainability, strong resistance to toxicity, high tolerance to the targeted contaminants' toxicity, enhanced removal efficiency of the targeted contaminants, protection of the introduced microorganisms, and broader operational range of temperature and pH.60–64 Interestingly, among various immobilization support matrix, sugarcane bagasse (SCB) has the advantages of appropriate porous properties, low-price, abundance, non-toxicity, high affinity to microorganisms, high mechanical strength, and easy biodegradability.45 Simultaneously, it is also capable of supplying essential organics and nutrients so as to support bacterial growth.
To the best of our knowledge, there have been no reported applications of the bacterium Burkholderia cepacia (BC) immobilized on sugarcane bagasse (SCB) for the remediation of TCAs-contaminated soil. Therefore, the present study thoroughly investigated the effectiveness and feasibility of the bioaugmentation performance of the bacterium BC immobilized on SCB for the degradation of TCAs in soil. To determine the optimum microbial degradation conditions, the effect of various inoculation doses, variable temperature, and diverse initial pH on the bioaugmentation performance of the bacterium BC in the process of OTC elimination was investigated. Moreover, the effect of single substrate concentrations, indigenous microorganisms, the presence of Pb2+ as well as Cd2+, co-culture, and double substrates was investigated successively.
2 Experimental
2.1 Chemicals and reagents
Analytical standards of OTC and TC were purchased from Shanghai Macklin Biochemical Co., Ltd. High performance liquid chromatography (HPLC) grade methanol, acetonitrile, and formic acid were obtained from Tianjin Kemiou Chemical Reagent Co., Ltd. Other analytical grade chemicals such as oxalic acid, citric acid monohydrate (C6H8O7·H2O), disodium ethylenediamine tetraacetate (Na2EDTA, C10H14N2Na2O8), sodium phosphate dibasic dodecahydrate (Na2HPO4·12H2O), magnesium nitrate Mg(NO3)2, and ammonia solution (NH3·H2O, 25%) were obtained from Guangzhou Chemical Reagent Technology Co., Ltd (Guangdong, China). The molecules of the TCAs contained a central octahydronaphthacene core and multiple ionizable functional groups such as hydroxyl group, phenolic group, and dimethylamino group that bind to the four rings of the structure.65 The B, C, and D rings mainly absorb light at wavelengths of 250–300 as well as 340–380 nm. Table 1 depicts the molecular formula, INN, CAS registry No., chemical structure, and pKa of oxytetracycline (OTC) and tetracycline (TC).
Table 1 The molecular formula, INN, CAS registry No., chemical structure, and pKa of oxytetracycline (OTC) and tetracycline (TC)
INN |
IUPAC name, molecular formula, INN and CAS Registry No. |
Chemical structure |
pKa |
Oxytetracycline (OTC) |
[4S-(4α,4aα,5α,5aα,-6β,12aα)]-4-(Dimethylamino)-1,4,4a,5,5a,6,11,12a-octa-hydro-3,5,6,10,12,12a-hexahydroxy-6-methyl-1,11-dioxo-2-naphthacenecarbox-amide, C22H24N2O9, 79-57-2 |
|
HA 3.3 (ref. 65) |
HA 7.3 (ref. 65) |
HB+ 9.1 (ref. 65) |
Tetracycline (TC) |
[4S-(4α,4aα,5aα,6β,-12aα)]-4-(Dimethylamino)-1,4,4a,5,5a,6,-11,12a-octahydro-3,6,10,12,12a-pentahydroxy-6-methyl-1,11-dioxo-2-naphthacenecarboxamide, C22H24N2O8, 60-54-8 |
|
HA 3.3 (ref. 65) |
HA 7.7 (ref. 65) |
HB+ 9.7 (ref. 65) |
2.2 Soil samples and soil properties
Uncontaminated soil samples were randomly gathered from an arboretum situated in the South China Agricultural University, which were not exposed to TCAs before collection. Upon collection, the samples of soil were air-dried, followed by homogenization by a pulverizator, and were sieved through a 3 mm pore-size mesh to guarantee their uniformity, which were finally stored at room temperature in dry and temperate conditions prior to use. The soil samples for the extraction method development, validation tests, and biodegradation experiments were previously analyzed to confirm that they were free from the two target analytes, namely, OTC and TC, and heavy metal ions Cd2+ and Pb2+. The physiochemical characteristics of the soil were determined utilizing the routine methods in laboratory. In brief, the soil pH was measured using an electrode-equipped pH meter in distilled water with a soil
:
water ratio of 1
:
2.5 (g
:
mL). The moisture content was determined by drying a pre-weighed soil sample in an oven at 65 °C until it reached constant weight, followed by the calculation of mass loss. Soil organic matter (SOM) was determined by means of the K2Cr2O7–H2SO4 oxidation method. Cation exchange capacity was determined using the barium chloride–sulfuric acid forced exchange method. The total iron in soil was determined by triacid elimination method. All the measurements were performed in triplicate. The characteristics of the soil samples were as follows: moisture content, 0.85%; soil organic matter, 0.57 g kg−1; pH, 4.52; cation exchange capacity 5.90 cmol kg−1, total iron 45 mg kg−1 (Table 2).
Table 2 Basic physiochemical characteristics of the soil
Moisture content (%) |
Soil organic matter (g kg−1) |
pH |
Cation exchange capacity (cmol k·g−1) |
Total iron (g kg−1) |
0.85 |
0.57 |
4.52 |
5.90 |
45 |
2.3 Pig manure and culture medium
The samples of pig manure were collected from a pig farm located in Guangzhou in Guangdong Province, China. The minimal mineral salt medium (MMSM) (pH 7.2), also known the selective liquid culture medium for isolation, growth, and biodegradation experiments, consisted of NH4NO3 (1.0 g L−1), K2HPO4 (0.5 g L−1), KH2PO4 (0.5 g L−1), NaCl (0.2 g L−1), and MgSO4 (0.2 g L−1) supplemented with varying contents of OTC in accordance with the requirements of the experiments and 10 mL of the trace element solution. In total, 1.5–2.0% (w/v) agar was added to prepare the solid medium. In particular, the trace element solution consisted of the following (in g L−1): MgSO4 3, FeSO4 0.1, MnSO4 0.1, ZnSO4 0.1, CaCl2 0.1, CuSO4 0.1, and Na2MoO4 0.01. The inorganic salt solution consisted of NH4NO3 (1.0 g L−1), K2HPO4 (0.5 g L−1), KH2PO4 (0.5 g L−1), NaCl (0.2 g L−1), MgSO4 (0.2 g L−1), and 10 mL of the trace element solution. All the media and solutions were sterilized at 121 °C for 30 min and immediately cooled to room temperature at a clean bench prior to use.
2.4 Stock solutions, extraction solvents, and simulated contaminated soil
For the determination of TCAs, individual stock solutions of the two target analytes OTC and TC standards was prepared by dissolving each standard in 0.01 M CaCl2 solution at the concentration of 1000 mg L−1 in a 1000 mL volumetric flask, and the obtained mother liquor was diluted to the required concentrations in the series of concentrations of 1 mg L−1, 5 mg L−1, 10 mg L−1, 20 mg L−1, 30 mg L−1, 40 mg L−1, 50 mg L−1, and 100 mg L−1, which were freshly prepared just prior to use. Pb2+ and Cd2+ stock solutions were obtained by dissolving PbCl2 and CdCl2 in sterile deionized water and added to the contaminated soil to make the concentrations of 102.6 mg kg−1 and 158.7 mg kg−1, respectively. Furthermore, 0.1 M Na2EDTA–McIlvaine buffer (pH 4) was obtained by dissolving 12.91 g C6H8O7·H2O, 27.61 g Na2HPO4·12H2O, and 37.20 g of Na2EDTA in 1000 mL distilled water. 50% Mg(NO3)2 (w/v) was prepared by dissolving 50.0 g Mg(NO3)2·6H2O in 100 mL distilled water. 2.5% NH3·H2O (the solution was freshly prepared just before use) was obtained by diluting 25% NH3·H2O tenfold, and the Mg(NO3)2–NH3·H2O solution was prepared by mixing the 50% Mg(NO3)2 and 2.5% NH3·H2O solutions in the ratio of 96
:
4 (v/v). The extraction solvents, the mixture of the Na2EDTA–McIlvaine buffer in combination with acetonitrile (v/v, 1
:
1) and Mg(NO3)2–NH3·H2O (v/v, 3
:
1), can be successfully used to extract OTC and TC from the soil samples with satisfactory recoveries.
The tested soil was treated with OTC and TC solutions. The OTC and TC solutions were obtained by dissolving 0.1 g of the target analytes OTC and TC in a moderate amount of methanol until they were completely dissolved, which was then added to 100 g of the tested soil, follow by stirring evenly and air drying. Excess methanol was removed through evaporation. The obtained contaminated soil was mixed with 900 g of the tested soil to obtain the final concentration of OTC and TC of 100 mg kg−1, which was preserved at room temperature for further use.
2.5 Enrichment, acclimation, and isolation of the microbial degrading cultures
Microbial cultures capable of degrading OTC were obtained by the enrichment and acclimation of inoculated cultures with the target antibiotics OTC as the sole carbon source, using approximately 1.5 g pig manure samples as the microbial inoculum. The enrichment processes were as follows: approximately 1.5 g pig manure was added to a 250 mL sterilized Erlenmeyer flask containing 100 mL of sterilized water, followed by incubation at 28 °C for 30 min on a constant temperature incubator shaker with a shaking speed of 160 rpm in the dark, and the cell suspensions were obtained. Subsequently, 1 mL of the cell suspension was evenly plated on agar plates with OTC (50 mg L−1) as the sole carbon source. The process of acclimation was carried out in triplicate for periods of two months under aerobic conditions. The enriched microbial cultures cultured in the solid medium were incubated at 28 °C in the dark on a constant temperature and humidity incubator. After a five-day interval, the resultant microbial cultures possessing diverse morphology were transferred from the former medium to the fresh medium, with increasing OTC concentrations from 50 mg L−1 to 250 mg L−1. Last but not the least, the obtained microbiota were added to the acclimated microbiota with the concentration of OTC at 250 mg L−1. The obtained morphologically distinct acclimated microbiota were purified by repeated plate streaking into the corresponding agar plates and were further preserved at 4 °C for further use.
2.6 Identification of the microbial isolates
The purified microbial isolates acquired from the OTC-enriched cultures were characterized and identified using the biochemical characterization tests, the 16S rDNA gene sequence analysis, as well as the ITS sequence analysis. Traditional biochemical methods face great difficulty in correctly classifying the microorganisms. With the development of molecular biology technology, nucleic acid sequence analysis has been widely used in the identification of microorganisms. Gene sequence analysis can quickly and accurately obtain gene sequences and analyze the microbial species. The identification procedure mainly consisted of DNA extraction, PCR amplification, and sequencing. The PCR amplification reaction system contained the following: 15 μL 2 × PCR mix, 1 μL DNA, 12 μL ddH2O, 2 μL primers. The PCR amplification procedure is described as follows: pre-denaturation at 95 °C from 0 to 5 min, 35 cycles of denaturation for 30 s at 95 °C, annealing for 30 s at 58 °C, extension for 45 s at 72 °C, a final elongation for 5 min at 72 °C, and keeping warm at 4 °C. After PCR amplification, the PCR products were tested by a gel imaging analyzer to confirm the PCR amplified fragments. The obtained nucleotide sequences were compared to those available in the NCBI database by means of a BLAST search.
2.7 Cell growth
The obtained purified cultures were inoculated into a pre-sterilized solid culture medium containing 250 mg L−1 OTC and then incubated at 28 °C in the dark on a constant temperature and humidity incubator for 3 d. Subsequently, the obtained well-grown strains were rinsed with sterilized saline solution (0.9% NaCl; w/v) and stirred evenly, and re-suspended in 250 mL sterilized 0.9% NaCl. In addition, 5 mL of the obtained cell suspensions was added to 250 mL minimal mineral salt medium (MMSM) containing OTC (250 mg L−1) and then incubated at 28 °C in the dark on a rotary shaker at 160 rpm for 2 d. Abiotic controls without microbial inoculation were incubated under the same conditions. The experimental treatments as well as the controls were set up in triplicate. In the course of the incubation, microbial growth was monitored by measuring the absorbance at 600 nm with a UV-1100 ultraviolet spectrophotometer (Mapada, China).
2.8 Inoculum preparation
The obtained purified cultures were inoculated into a pre-sterilized solid culture medium containing 250 mg L−1 OTC and then incubated at 28 °C in the dark on a constant temperature and humidity incubator for 3 d. Subsequently, the obtained well-grown strains were rinsed with sterilized saline solution (0.9% NaCl; w/v) and stirred evenly, and resuspended in sterilized 0.9% NaCl solution. The cell suspensions were adjusted to an absorbance of 0.5 at an optical density (OD) of 600, which was referred to as the inoculum for further experiments.
2.9 Cell immobilization procedure
Cell immobilization by adsorption was carried out by adding 1 g of SCB into a 100 mL beaker and an adequate amount of inorganic salt solution before inoculation with the inoculum (Scheme 1). Prior to immobilization, the obtained purified cultures were inoculated into a pre-sterilized solid culture medium containing OTC (250 mg L−1) and then incubated at 28 °C in the dark on a constant temperature and humidity incubator for 3 d. Subsequently, the obtained well-grown strains were rinsed with sterilized saline solution (0.9% NaCl; w/v) and stirred evenly, which was referred to as the inoculum for further experiments. The initial optical density (OD600) of the inoculum was approximately 0.5. Then, to avoid other microbiological interferences, all the prepared media, solutions used, and all the 100 mL beakers supplied with 1 g of SCB that was directly used as a support matrix for the cell immobilization were sterilized by autoclaving at 121 °C for 30 min. Ultimately, an adequate amount of the sterilized inorganic salt solution and the inoculum were added into a sterilized 100 mL beaker supplied with 1 g of SCB, followed by incubated at 28 °C in the dark on a constant temperature and humidity incubator for 3 d.
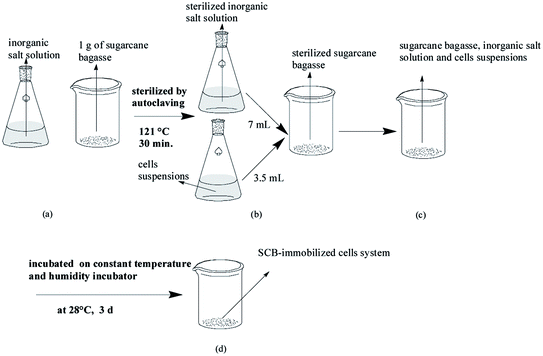 |
| Scheme 1 The cells' immobilization procedure. | |
2.10 Soil extraction procedure
In this work, the soil samples were successfully extracted with a mixture of Na2EDTA–McIlvaine buffer in combination with acetonitrile (v/v, 1
:
1)
:
Mg(NO3)2–NH3·H2O (v/v, 3
:
1) with ultrasonic assistance. The extraction was carried out following the procedure described herein: 10 g of the contaminated soil samples were accurately weighed in triplicate into a 50 mL centrifuge tube and mixed with the extraction solvents. The centrifuge tubes were placed on a rotary shaker at 150 rpm for 10 min for homogenization, followed by ultrasonic treatment for 30 min in an ultrasonic bath and then centrifugation at 5000 rpm for 10 min. The supernatant was collected in a clean and dry beaker and the above-mentioned procedure was repeated for each soil sample two more times. All of the obtained supernatants were mixed thoroughly and filtered through a 0.2 μm syringe filter and pipetted into a sample tube, and then preserved at 4 °C in a refrigerator prior to HPLC analysis. All the experiments were performed in triplicate.
2.11 Experimental design
2.11.1 The reaction system set-up.
The experiments of microbial degradation of the TCAs in soil were carried out as follows. The cell immobilization procedure used in the current study has been described above (Scheme 1). With regard to the freely suspended cells, all of the 100 mL beakers for the freely suspended cells group were sterilized by autoclaving at 121 °C for 30 min, followed by the addition of an adequate amount of the sterilized inorganic salt solution and the inoculum into the sterilized 100 mL beakers, which were finally incubated at 28 °C in the dark on a constant temperature and humidity incubator for 3 d. After 3 d of incubation, 10 g of contaminated soil (dry weight) was weighed into the pre-sterilized 100 mL beakers containing the introduced microorganisms that were already grown (Scheme 2). All of the beakers were placed on a constant temperature incubator in the dark to minimize any possible photodegradation,27 mainly because the antibiotics in the soil may be degraded or transformed through biotic or abiotic reactions, particularly photochemical transformations and chemically mediated transformations such as hydrolysis, oxidation, and reduction, which are the most important abiotic reactions.26 The water content of the soil in the reaction system was maintained at 50% of the water holding capacity (WHC) by the addition of a certain amount of the sterilized inorganic salt solution and the initial nominal concentration of 100 mg kg−1 for OTC and TC in soil. This was mainly because the water content of soil is generally low, and so it was necessary to add a certain amount of water prior to target analytes' extraction in order to hydrate the sample, which would improve the extraction efficiency of the polar target analytes.66 It was noteworthy that all of the above experiments were carried out at a constant temperature of 28 °C in triplicate and the residual concentration of the TCAs was monitored at 5 d intervals for a month.
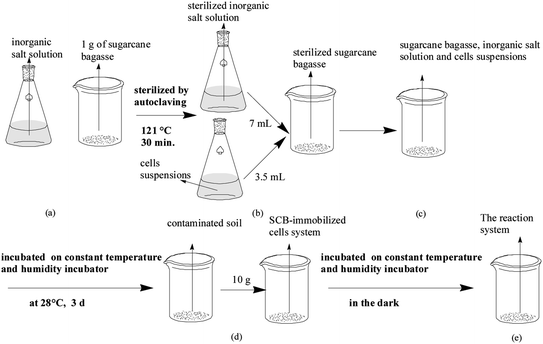 |
| Scheme 2 The reaction system set-up. | |
2.11.2 Bioaugmentation assay of the freely suspended cells and immobilized cells.
To further confirm the advantages of the microbial degradation of TCAs in soil by the immobilized cells, the microbial degradation of the selected antibiotics OTC and TC in non-sterilized soils by the freely suspended cells and immobilized cells was investigated under the same conditions. In order to assay the bioaugmentation properties of the freely suspended and immobilized bacterium BC, the experimental design was as follows: control group without microbial inoculation (control), free bacterium BC (free), and SCB-immobilized bacterium BC (immobilized). The experimental controls were also established in parallel to estimate the abiotic degradation of the target analytes and their adsorption potential.67
2.11.3 Factors influencing bioaugmentation.
To facilitate the biodegradation studies, the original experimental conditions were set as follows: at room temperature (ca. 25 °C), pH 4.5, 3.5 mL of the cell suspensions (107 CFU mL−1), 7 mL sterilized inorganic salt solution, protected from light and in static conditions. In practical applications, it is still vitally important to evaluate the effects of the degradation conditions and to determine the most suitable parameters for the degradation of target contaminants. Thus, critical intrinsic and extrinsic environmental factors such as inoculation dose, temperature, and initial pH were varied to optimize the biodegradation potential of the bacterium BC. To explore the bioaugmentation properties of the SCB-immobilized bacterium BC, serial batch-type biodegradation experiments were carried out at various inoculation doses (5%, 10%, 15%, 20%, and 25%; v/w, namely 1 mL, 2 mL, 3.5 mL, 4.5 mL, and 6 mL, 107 CFU mL−1), various temperatures (23 °C, 28 °C, 33 °C, 38 °C, and 43 °C), various initial pH values (2.5, 3.5, 4.5, 5.5, and 6.5) in order to determine the optimal conditions. The initial pH of contaminated soil was adjusted by HCl or NaOH. The effects of the substrate concentrations, the indigenous microorganisms, the presence of Pb2+ as well as Cd2+, and co-culture were also determined. In addition, we also investigated the dual-substrate co-biodegradation behaviors of TC and OTC because the TCA contaminants usually co-exist in the environment with interactional effects.
In order to investigate the dual-substrate co-biodegradation behaviors of OTC and TC and the interaction mechanism, we established the dual-substrate biodegradation systems of OTC-TC. In dual-substrate biodegradation, two groups of co-biodegradations of OTC and TC was carried out. Group one was conducted with TC and OTC concentrations of 10 mg kg−1 and 100 mg kg−1, and 20 mg kg−1 and 100 mg kg−1 to study the effect of low-concentration TC on the maximum OTC degradation efficiency, respectively. The other group was performed with 100 mg kg−1 of OTC, while the concentration of TC was respectively varied as 40 mg kg−1, 60 mg kg−1, 80 mg kg−1, 100 mg kg−1, and 120 mg kg−1 in order to study the effects of TC concentration on the OTC degradation velocity.
2.12 Analytical methods
The growth of the introduced strains was observed according to the intensity of optical density at 600 nm. In the course of the incubation, microbial growth was monitored by measuring the absorbance at 600 nm with a UV-1100 ultraviolet spectrophotometer (Mapada, China).
Owing to its high separation efficiency and accuracy in quantitative analysis, high-performance liquid chromatography (HPLC) has been most frequently utilized in the separation, identification, and determination of the TCAs residues in various matrices.5,68 In accordance with the previous research reports, the quantitative determination of the TCAs residues in soil was carried out by means of a reversed phase high-performance liquid chromatograph (HPLC) (Agilent 1100 HPLC) equipped with a UV-Vis detector.11,19 Chromatographic separation was achieved with an Agilent ZORBAX Eclipse XDB-C18 reverse-phase column φ 4.5 mm × 280 mm (250 mm × 4.6 mm, 5 μm) at the flow rate of 1.00 mL min−1 at 25 °C. The composition of the mobile phase is a very important parameter in adjusting the retention time, selectivity, and peak shape in HPLC separation.66 The addition of acidic additives in the mobile phase could improve the positive ESI ionization.3 It has been demonstrated that the addition of formic acid (0.1%) into the mobile phase can increase the ionization efficiency of the analytes and improve chromatographic separation.69,70 The mobile phase was comprised of an aqueous phase (pure water containing 0.02% formic acid with pH adjusted to 3.2 approximately) and an organic phase (acetonitrile) in the proportion 85
:
15 (v/v), which was confirmed to be the best combination and provided the best peak shapes for all the analytes. All the experiments and measurements were performed in triplicate and arithmetic averages were taken for data analysis. The error bars are representative of the standard deviation of the mean of the triplicate experiments.
3 Results and discussion
3.1 Isolation and identification of high efficiency TCA-degrading bacteria
After repeated acclimatization and purification, a total of 9 strains with high tolerance to and high degrading ability of TCAs were isolated from the acclimated microbiota and were used to form colonies on a solid medium with OTC (250 mg L−1) as the sole carbon source. These isolates immobilized on SCB were able to degrade OTC in soil to varying degrees. In comparison to other isolates, strain OTC-1 immobilized on SCB exhibited the highest average degradation efficiency during a month's treatment at room temperature (ca. 25 °C), followed by strain OTC-9 and strain OTC-6, with the average degradation efficiencies of OTC reaching 79.20 ± 0.32%, 78.07 ± 1.36%, and 75.12 ± 0.35%, respectively. The obtained TCA-degrading strains were identified in view of the physiological and biochemical tests, and 16S rRNA sequence analysis. From the 16S rRNA gene sequence, strain OTC-1, strain OTC-6, and strain OTC-9 were identified as Burkholderia cepacia (BC), Pseudomonas nitroreducens NBRC (PN), and Acinetobacter johnsonii (AJ), respectively. The bacterium BC was subjected to further microbial degradation experiments due to its outstanding ability to bio-transform the OTC in soil. Biochemical characterization tests of the bacterium BC are presented in Table 3, which show that the bacterium BC was starch hydrolysis-positive, catalase-positive, indole-positive, methyl red-negative, facultatively anaerobic or anaerobic, and motile.
Table 3 Characterization of the BC bacterium, Burkholderia cepacia (‘−’ negative result, ‘+’ positive result)
Test |
Phenomenon |
Result |
Starch hydrolysis |
Hydrolyzed |
+ |
Catalase |
Oxygen |
+ |
Indole |
A red ring appears at the interface |
+ |
Methyl red |
Faded to yellow |
− |
Aerobic or anaerobic |
Bacteria grows on puncture lines and medium surfaces |
+ |
Kinetic |
Bacterial growth is not limited to puncture lines |
+ |
3.2 Cell growth
Cell growth for the bacterium BC is shown in Fig. 1. In the first 3 h, cell growth was relatively slow while the OD600 increased from 0.004 to 0.033. This observation indicated that the bacterium BC began to adapt to the environment in the culture medium. From 3 h to 21 h, cell growth with a specific growth rate of 0.033 h−1 reached the logarithmic growth period as the OD600 increased to 0.626, indicating that the bacterium BC had a stronger capability to adapt to the environment in the minimal mineral salt medium (MMSM) with OTC (250 mg L−1) as the sole carbon source. However, cell growth passed through a short stationary phase during 21–30 h and then reached its decline phase after 30 h probably due to the decrease in the carbon source in the culture medium as well as the limited space environment.
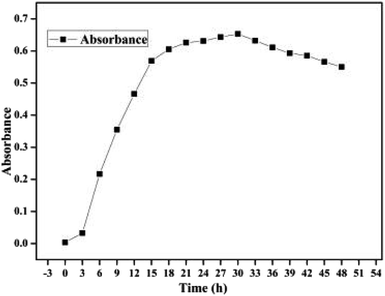 |
| Fig. 1 Cell growth for the bacterium Burkholderia cepacia. | |
3.3 Bioaugmentation assay of the bacterium Burkholderia cepacia
To explore the bioaugmentation potential of the bacterium BC, the microbial degradation of OTC in soil mediated by the freely suspended cells or immobilized cells was investigated successively. It can be observed from Fig. 2 that the average degradation efficiency of OTC in soil by the immobilized cells (79.20 ± 0.32%) was remarkably higher than that by the freely suspended cells (60.07 ± 1.22%) at the initial concentration of 100 mg kg−1 within a month's incubation. This indicated that the SCB-immobilized bacterium BC exhibited better performance than the freely suspended bacterium BC with regard to the elimination of OTC in soil under identical experimental conditions. On the one hand, this could be due to the excellent structure of SCB, which contributed to the adhesion and proliferation of microbes on the SCB, resulting in an outstanding mass transfer performance, enhanced cell permeability, and high biological density.61,62 On the other hand, the porous SCB was capable of providing an ideal shelter and biocompatible environment for the introduced microorganisms and for partially resisting the adverse effects of substrate OTC inhibition,66 and its decomposition that supplied carbon and nitrogen could increase soil nutrients so as to create a condition that was favorable for the growth of the introduced microorganisms.60,61 Moreover, in terms of the bioprocess mediated by the freely suspended cells or immobilized cells, the degradation rate reached a maximum of 4.27 mg kg−1 d−1 and 4.75 mg kg−1 d−1 on the 5th d post-inoculation, respectively, and gradually reduced as the incubation time increased, which revealed that the bacterium BC could rapidly adapt to the OTC-contaminated soil and had potential for the remediation of OTC-contaminated soils. It can be observed that the biodegradation rate of OTC in soils mediated by the freely suspended cells or immobilized cells was rapid in the initial stage, then gradually slowed down. The observed decrease in the biodegradation rate of OTC at the later stage was most likely due to the accumulation of toxic intermediates, the lower nutrient levels, as well as the cell densities, thereby possibly impacting its biodegradation capacity.71 The findings were consistent with a previous research showing that the nutrient level significantly affected the removal and microbial growth of the target contaminants, with a higher nutrient level favoring a better performance.62 In contrast, in the control group without microbial inoculation, only 4.99 ± 0.39% OTC reduction was observed, which indicated that OTC was difficult to degrade in a short time in a natural soil environment, abiotic mechanisms played a weak role in the elimination of OTC, and the overall process was largely controlled by the microbial metabolism.65
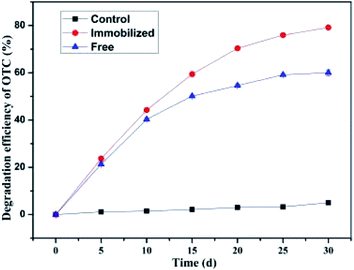 |
| Fig. 2 Microbial degradation of OTC in soil by the freely suspended cells or immobilized cells. | |
3.4 Optimization of the bioaugmentation potential of the SCB-immobilized Burkholderia cepacia
Understanding the factors affecting the biodegradation or bioremediation is of great significance to determine the cost-effective protection and restoration of OTC-contaminated sites. Microbes derived from various environments possess various optimal living conditions and are capable of adapting to contaminants and contaminated environments via self-regulation.73 In practical applications, it is still vitally important to evaluate the effect of the degradation conditions and to determine the most suitable parameters for the degradation of the target contaminants. Thus, critical intrinsic and extrinsic environmental factors such as inoculation dose, temperature, and initial pH were varied to optimize the bioaugmentation potential of the bacterium BC.
3.4.1. Effect of inoculation doses.
It can be seen from Fig. 3 that the inoculation doses of the bacterial culture had no obvious effect on the degradation efficiency of OTC in soil. After a month's incubation, with the inoculation doses of 5%, 10%, 15%, 20%, and 25% (v/w) of the bacterium BC, the average OTC degradation efficiencies of the SCB-immobilized bacterium BC were 68.00 ± 0.93%, 71.04 ± 0.41%, 79.19 ± 0.66%, 78.87 ± 0.50%, and 76.27 ± 0.24%, respectively. The finding of this research regarding the effect of the inoculation doses on OTC biodegradation was consistent with that of the previous studies.52 On increasing the incubation time, the average OTC degradation efficiencies increased rapidly (0–15 d) and then decreased slowly (15–30 d) during the reaction period. From the above analysis, it can be seen that the average OTC degradation efficiency increased with the increase in the inoculation dose from 5% to 15%. However, further increasing the inoculation dose did not improve the average OTC degradation efficiency, which could be due to the depletion of the nutrient sources in the reaction system.72 The obtained results indicated that no significant difference was observed in the average OTC degradation efficiency with the bacterium BC inoculation doses of 15% and 20% (v/w) within a month's incubation. The inoculation dose was an important indicator to evaluate the practical application of the engineered functional bacteria.52 In the actual practical application of the engineered functional bacteria, the lesser the engineered functional bacteria inoculation dose directed at ensuring effective treatment, the lower would be the cost incurred.52 Considering the details mentioned above, the inoculation dose of 15% (v/w), i.e., 3.5 mL, was regarded as the optimum initial bacterial in the present study.
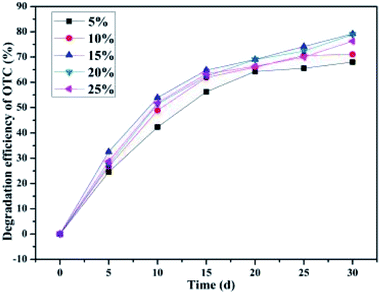 |
| Fig. 3 Effect of the inoculation doses. | |
3.4.2. Effect of the incubation temperature.
Generally speaking, temperature, as one of the crucial external factors, has an influence on the cellular enzyme activity as well as the reaction rate of the cells.52 A vast majority of microorganisms require a suitable temperature for cell growth and metabolism.52 As illustrated in Fig. 4, when the incubation temperature was maintained at 23 °C, 28 °C, 33 °C, 38 °C, and 43 °C, the average OTC degradation efficiencies obtained were 69.64 ± 0.24%, 72.77 ± 0.89%, 76.43 ± 0.08%, 85.11 ± 0.75%, and 76.63 ± 0.13%, respectively. As the incubation temperature increased from 23 °C to 43 °C, the average OTC degradation efficiencies rapidly increased first (23–38 °C) and then dramatically reduced (38–43 °C) within a month's incubation. The optimal incubation temperatures for OTC degradation were found to be 33 °C, 38 °C, and 43 °C, at which the maximum degradation rates of 2.56 mg kg−1 d−1, 2.85 mg kg−1 d−1, and 2.58 mg kg−1 d−1, respectively, were achieved. This observation was consistent with the study of Shao et al., who also found that with the increase in temperature, the OTC degradation efficiency by the strain Ochrobactrum sp. KSS10 first increased (20–35 °C) and then decreased (35–40 °C) during the reaction period.50 These results demonstrated that the incubation temperature had a significant influence on the biodegradation activity of the bacterium BC, and temperatures between 23 °C and 38 °C accelerated the biodegradation of OTC in the SCB-immobilized cells system. Comparatively, a temperature between 30–35 °C was the best suited for TC biodegradation by the strain Klebsiella sp. SQY5.52 Previous studies have indicated that the influences of temperature and pH variation on OTC biodegradation could be explained by the fact that enzymatic activity in organisms is directly affected by temperature and pH,50 while the enzymatic activity in microorganisms is one of the main factors in the course of biodegradation.72 Based on the results and the findings in previous studies,50 it has been postulated that the ability of the enzymes in the bacterium BC involved in the process of OTC biodegradation could be considerably influenced by temperature. It could be concluded that the higher the temperature, the greater the enzymatic activity of the bacterium BC, and the better the degradation ability of the bacterium BC. This could be explained by the collision theory, in which higher temperatures resulted in higher reaction rates and an increase in the contact area with the bacteria by means of more collisions between the particles.52 A previous study has also pointed out that temperature had a significant impact on microbial activity as well as the enzymatic activity, with the optimal temperatures shown for specific microorganisms.73 Thus, the obtained results indicated that the optimal temperature for the degradation of OTC by the bacterium BC was 38 °C, whereas the optimal temperatures for OTC degradation by the strain Ochrobactrum sp. KSS10 were found to be 30 °C and 35 °C, at which the maximum degradation rates of 2.748 mg L−1 d−1 and mg L−1 d−1, respectively, were achieved.50 Interestingly, even when the incubation temperature rose to 43 °C, the SCB-immobilized cells system could maintain more than 76 percent of the maximum OTC average degradation efficiency, suggesting their strong adaptability to high incubation temperature. Furthermore, in all the temperature ranges, the SCB-immobilized cell system could still maintain more than 70 percent of the maximum average degradation efficiency, which indicated that the SCB-immobilized cells system exhibited high degradation activity over a broad temperature range. In order to reduce the economic cost of bioremediation of OTC-contaminated soil, the temperature should be set at the lowest possible.74 Thus, temperatures between 23–38 °C were the best suited for OTC biodegradation by the SCB-immobilized bacterium BC.
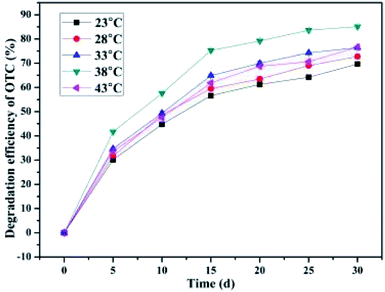 |
| Fig. 4 Effect of the incubation temperature. | |
3.4.3 Effect of initial pH.
Simultaneously, pH is also one of the crucial external factors that affects the enzyme activity as well as the reaction rate of the cells.52 To determine the effect of initial pH value of the contaminated soil on OTC degradation by the immobilized cells, batch experiments were carried out at various pH values (2.5, 3.5, 4.5, 5.5, and 6.5). Fig. 5 depicts the average OTC degradation efficiency with the initial pH value in the range of 2.5–6.5. As the pH increased from 2.5 to 6.5, the average OTC degradation efficiencies of 63.96 ± 0.62%, 65.93 ± 0.41%, 75.68 ± 0.49%, 71.42 ± 0.46%, and 66.34 ± 1.16% were achieved within a month's incubation, respectively. This observation indicated that the average OTC degradation efficiencies in the SCB-immobilized cell system first increased (pH 2.5–4.5) and then appeared to the show a declining trend (pH 4.5–6.5). The highest OTC average degradation efficiency reached 75.68 ± 0.19% when the initial pH value was 4.5. The results showed that slightly acidic pH (4.5–6.5) was the most suitable for OTC degradation by the SCB-immobilized bacterium BC. The pH value was an important factor in the process of microbial metabolism because it could exert an influence on the microbial growth as well as the enzymatic activity.63,75 Nevertheless, Shao et al. revealed that the strain Ochrobactrum sp. KSS10 could tolerate highly alkaline environments and the strain could perform its OTC degradation functions at a wide pH range (5–8).50 Previous studies have demonstrated that a higher or lower pH value might be responsible for the partial inactivation of the enzyme, which led to slower biodegradation of the targeted contaminants.75 On the other hand, the SCB-immobilized cells still maintained higher degradation efficiencies (more than 60%) under a wide range of pH range from 2.5 to 6.5, indicating the good tolerability of the SCB-immobilized cells to the initial pH and its high degradation activity over a wide range of pH values (2.5–6.5), which would be a clear benefit for the actual practical application. This was probably because the immobilized carrier could provide the buffering effect, which is attributed to the adsorption of the target contaminants and toxic compounds such as intermediate products of OTC on the immobilized carrier, thereby protecting cells from toxic compounds and providing resistance against adverse environments.63 The results indicated that the immobilized carrier SCB could act as a barrier that could protect the cells from the target contaminants and toxic compounds such as the intermediate products and exhibited a strong adaptability to unfavorable environment such as acidic and alkaline environments. Based on these observations and combined with the observations of the effect of the incubation temperature, it is reasonable to infer that the bacterium BC could degrade OTC in soil effectively at a relatively broad range of temperature and pH, which was advantageous compared with the bacterial strains described in most previous studies50,52 that were just applied in the basal medium (BM).
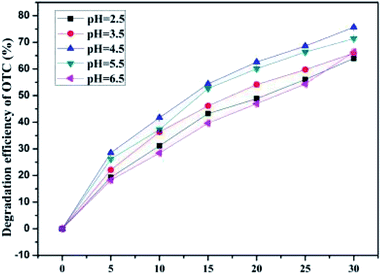 |
| Fig. 5 Effect of the initial pH. | |
3.5 Effect of the initial OTC concentration
In addition, batch experiments were carried out to evaluate the effect of initial OTC concentration on the average OTC degradation efficiency in the SCB-immobilized cell system. As illustrated in Fig. 6, the average initial concentration of OTC changed from 77.11 ± 0.43, 100.36 ± 0.29, 117.02 ± 0.31, 130.65 ± 0.69, 150.12 ± 0.81, and 165.35 ± 0.28 mg kg−1 to 19.58 ± 0.25, 20.87 ± 0.32, 19.1 ± 0.23, 18.98 ± 0.37, 40.07 ± 0.49, and 52.19 ± 0.85 mg kg−1, respectively, and the average OTC degradation efficiencies of 74.61 ± 0.25%, 79.20 ± 0.32%, 83.68 ± 0.23%, 85.50 ± 0.37%, 73.31 ± 0.49%, and 68.62 ± 0.85% were remarkably achieved as the initial OTC concentrations gradually increased from 80 mg kg−1 to 180 mg kg−1, respectively, within a month's incubation. It was found that the maximum OTC degradation rates of 1.93 mg kg−1 d−1, 2.56 mg kg−1 d−1, 3.26 mg kg−1 d−1, 3.72 mg kg−1 d−1, 3.67 mg kg−1 d−1, and 3.77 mg kg−1 d−1 were achieved by the SCB-immobilized bacterium BC within a month's incubation, respectively. These results illustrated that the average OTC degradation efficiency first significantly increased (from 80 mg kg−1 to 140 mg kg−1) and then appeared to decline (from 140 mg kg−1 to 180 mg kg−1). The lower OTC degradation efficiency at low initial OTC concentrations was probably attributed to the mass transfer control, i.e., less OTC was accessible for the biomass.63 The changing trends of the effect of initial OTC concentration on the average OTC degradation efficiency were consistent with that of the previous study.50,52 Shao50et al. found that the degradation efficiency of OTC increased first and then decreased when the initial OTC concentration was gradually increased from 10 mg L−1 to 100 mg L−1, while the OTC degradation efficiency was not improved when the initial OTC concentration increased up to 100 mg L−1. On the other hand, the TC degradation ratio reached its maximum value of 89.66% of the initial TC concentration of mg L−1.52 No obvious change in the final residual OTC in soil was observed when the OTC concentration was increased from 80 mg kg−1 to 140 mg kg−1 but the final residual OTC was greater when the initial OTC concentration increased from 160 mg kg−1 to 180 mg kg−1. These findings are different from those from the studies by Shao et al., which found that higher the initial concentration of OTC or TC, greater the residual concentration of OTC or TC in the basal medium (BM).50,52 The obtained results demonstrated that the SCB-immobilized cells were capable of using OTC as a carbon and energy source, and could withstand a selection pressure of 180 mg kg−1 OTC in soil. The decline in the OTC average degradation efficiency in the SCB-immobilized cell system at the initial OTC concentration of 160 mg kg−1 and 180 mg kg−1 could be presumably attributed to substrate inhibition, toxicity of OTC higher concentrations, and toxicity of the metabolites to the degradation ability of the bacterium BC. This phenomenon could be explained by the fact that the substrate OTC at high concentrations would cause toxicity to the introduced microorganism bacterium BC and inhibit the enzyme synthesis as well as cellular metabolism. Some previous studies are strongly in support of these findings in the present research. For instance, previous studies have suggested that a higher initial concentration of the targeted contaminants could cause toxicity to the introduced microorganisms and substrate inhibition in the biodegradation process.45,63,76,77 On the other hand, it was found that the average OTC degradation efficiency still reached 68% with the initial OTC concentration of 180 mg kg−1, indicating that the bacterium BC may resist a high concentration of OTC, which could be due to the advantage of protecting the cells from the inhibition of high concentrations of target contamination substrate provided by the immobilization carrier.63,78 The obtained results further confirmed that the bacterium BC was capable of using OTC as a carbon and energy source and exhibited high tolerance to a high concentration of OTC in the soil, which once again indicated that the bacterium BC was advantageous compared with the bacterial strains described in most previous studies50,52 that were just applied in the basal medium (BM).
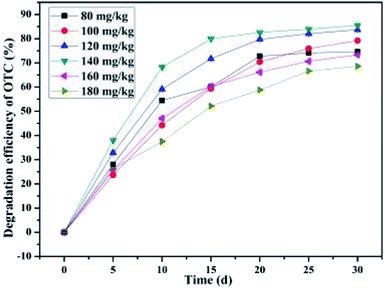 |
| Fig. 6 Effect of the initial concentration. | |
3.6 Effect of indigenous microorganisms
Previous studies have indicated that environmental isolates that serve as seeds for bioremediation must be capable of competing favorably with the autochthonous community in nutritionally limited, highly compacted, and varyingly contaminated soil environments.79 Thus, we also investigated the effect of the existence of indigenous microorganisms (NSB) in soil on the degradation ability of the bacterium BC. As illustrated in Fig. 7, it was found that the SCB-immobilized bacterium BC could efficiently degrade OTC in soils with or without the existence of indigenous microorganisms. The OTC average degradation efficiencies in soils with or without the existence of indigenous microorganisms were 79.20 ± 0.32% and 75.02 ± 0.90%, respectively, within a month's incubation, indicating that the presence of indigenous microbes increased the biodegradation of OTC by the bacterium BC. It should be noted that the existence of indigenous microorganisms (NSB) in soil exerted little effect on the degradation ability of the strain Burkholderia cepaci. This increase in the OTC average degradation efficiency was accounted for by the degradation efficiency of the indigenous microbial population alone. The finding was consistent with previous studies,80 which demonstrated that the presence of indigenous microbes enhanced the bioleaching of monazite by phosphate solubilizing microorganisms (PSMs) at rates greater than that recorded with either the indigenous microbes or the phosphate solubilizing microorganisms (PSMs) individually. In addition, in the control group without cell inoculation, with or without the existence of indigenous microbes, only 4.99 ± 0.39% and 3.68 ± 1.02% OTC reduction was observed, indicating that a slight decrease in the OTC reduction was found in the sterilized soil in comparison with the non-sterilized soil, thus once again implying that the indigenous microorganisms contributed to the OTC reduction and the presence of abiotic degradation of OTC in the soil environment. It was reported that it was difficult to achieve complete degradation of OTC in the environment, i.e., it required a long time for the completion of the degradation reaction.65 In the environment, the degradation pathways of OTC consisted of non-biodegradation (mainly photodegradation, hydrolysis, and oxidative degradation) and biodegradation (largely plant and microbial degradation).65 The results indicated that the bacterium BC could efficiently degrade OTC in soil with or without the existence of indigenous microorganisms with satisfactory degradation efficiency, indicating that the bacterium BC could fit into the simulated soil environment very well, without competition with indigenous microbes in the soil environment.
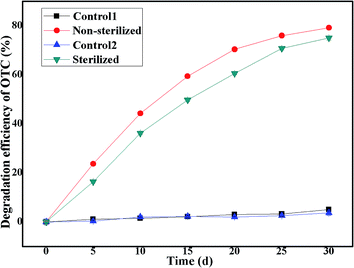 |
| Fig. 7 Effect of indigenous microorganisms. | |
3.7 Effect of the metal ion
The long-term continuous and heavy application of manures would exacerbate the persistence of antibiotics as well as heavy metals in agricultural soils.22 Zhao et al.22 have confirmed the co-contamination of antibiotics and metals in soils, and that metals significantly enhanced the absorption of TCs on soil organic matter. Metallic ions were typically simultaneously presented in contaminated soil with antibiotics; thus, their roles in the decomposition of OTC by the bacterium BC were investigated. Heavy metals (e.g., Cu, Zn, Mn, Ni, Pb, and Cd) could accumulate in the soil up to the level of toxicity, and among these heavy metals, Cd and Pb were of particular concern because of their highly hazardous nature.81 In order to investigate the conditions for the application, the influences of other contaminants such as Pb2+ and Cd2+ metal ions on the degradation of OTC by the bacterium BC were also assayed (Fig. 8). It can be seen from Fig. 8 that the presence of heavy metal ions Pb2+ and Cd2+ indeed exerted inhibitory influences on the average OTC degradation efficiency by the SCB-immobilized bacterium BC to some extents. The OTC degradation efficiency in the two groups with the presence of heavy metal ions Pb2+ and Cd2+ was consistently inferior to that in the control group during a month's treatment at room temperature. The inhibitory effect of Pb2+ on the OTC degradation (74.85 ± 0.31%) performance was greater than that of Cd2+ treatment (75.03 ± 0.45%). The findings were consistent with some previous research. For instance, it has been demonstrated that the microbial decomposition rate of organic contaminants such as glyphosate by indigenous soil bacterial isolates was decreased with the application of metal ions such as Fe3+ or Cu2+.82 The inhibition of OTC degradation by the bacterium BC was partly attributed to the enhancement of cell membrane permeability and the decrease in the cell membrane fluidity caused by the metal ions Pb2+ and Cd2+. Cd2+ is known to be cytotoxic to cells and exerts a direct and serious impact on the enzymatic activities involved in bacterial metabolism.83 The results from Qin et al. indicated that Cd2+ significantly inhibited the anaerobic biodegradation of benzo(a)pyrene by a novel strain Cellulosimicrobium cellulans CWS2 isolated from polycyclic aromatic hydrocarbon-contaminated soil.83 In addition, Liu et al. indicated that the presence of Pb2+ exerted a great inhibitory effect on the bacteria and caused the inhibition of protein synthesis, which has an adverse effect on the cellular metabolism of microbial communities as well as the abundance of microbial communities.84 Nonetheless, in the present study, with the existence of heavy metal ions Pb2+ and Cd2+, the SCB-immobilized cell system could still maintain more than 74 percent of the maximum average degradation efficiency, suggesting that the SCB-immobilized bacterium BC exhibited a high activity and biodegradation efficiency in the presence of heavy metal ions Pb2+ and Cd2+ and showed a strong resistance to heavy metals ions Pb2+ and Cd2+. Thus, we can speculate that the better adaptability of the bacterium BC to changing harsh contaminated soil environments make it more adaptable in the remediation of soils contaminated with various contaminants.
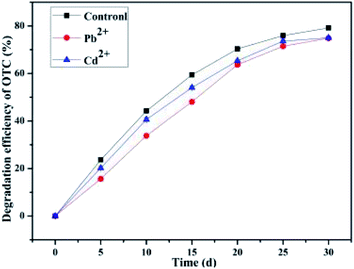 |
| Fig. 8 Effect of the metal ion. | |
3.8 Effect of co-culture
It has been demonstrated that the target contaminant degradation ability of the co-cultured pure isolates or microbial consortium was more effective than a single bacterium.85,86 This phenomenon could be due to metabolite exchange between the two strains.85 Culturing two strains together is a new biostimulation strategy in order to enhance the biodegradation of contaminants.85 The results given in Fig. 9 revealed that all the co-cultures of these three isolates might initially be capable of biodegrading the OTC in soil. Their biodegradation capabilities were in view of their adaptability in the OTC-contaminated soil environment. The biodegradation capabilities of the individual isolates of the bacterium BC were 79.20 ± 0.32% in comparison to the co-culture of the bacterium BC and the bacterium PN, which exhibited up to 85.31 ± 0.25% degradation efficiency within a month's incubation. This result reveals that the biodegradation capabilities of the co-culture of bacterium BC and bacterium PN were higher than that of the bacterium BC alone. The superior bioaugmentation performance of the bacterium BC and the bacterium PN may be attributable to the synergistic effect of similar enzymes in similar metabolic pathways.87 Previous research has found that bacterial co-cultures are of great significance and practical value because their synergistic action might improve their survivability in actual microenvironments.88 Moreover, the synergistic capacities of the bacterium BC and the bacterium PN exposed to OTC substrates in soil enable them to convert OTC substrates into intermediate products, while they used intermediate products of the other as their substrates to further transform them into to smaller molecular intermediate products or the final products, namely, water and carbon dioxide.89 However, the biodegradation capability of the co-culture of bacterium BC and bacterium AJ (72.66 ± 0.43%) was slightly lower than that by the individual isolates of bacterium BC (79.20 ± 0.32%). The SCB-immobilized bacterium BC exhibited the highest average degradation efficiency during a month's treatment at room temperature (ca. 25 °C), followed by SCB-immobilized bacterium AJ and SCB-immobilized bacterium PN, with the average degradation efficiencies of OTC reaching 79.20 ± 0.32%, 78.07 ± 1.36%, and 75.12 ± 0.35%, respectively. These results indicated that the biodegradation capabilities of the co-culture of bacterium BC and bacterium AJ (72.66 ± 0.43%) was even inferior to that by individual isolates of bacterium AJ and bacterium PN, which might be attributed to the inhibition effects of the intermediate products of OTC by bacterium AJ arising due to the their combined metabolic activities.
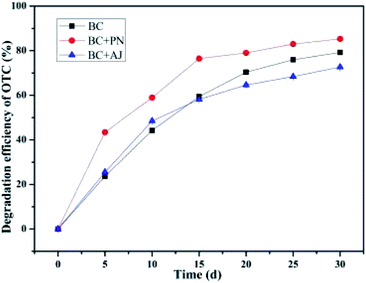 |
| Fig. 9 Effect of the co-culture. | |
3.9 Degradation of other TCAs
As illustrated in Fig. 10, the SCB-immobilized bacterium BC also exhibited efficient degradation ability for TC and its average degradation efficiency was 82.31 ± 0.62% with a degradation rate of 2.26 mg kg−1 d−1, which was far higher than that by the freely suspended cells that exhibit an average degradation efficiency of 67.21 ± 0.60% at a degradation rate of 1.85 mg kg−1 d−1. It can be observed that the biodegradation rate of TC in soils by the SCB-immobilized bacterium BC was rapid in the initial stage within 10 d, then gradually slowed down and eventually reached a plateau phase. In addition, the variation tendency of the biodegradation rate of TC in soils by the freely suspended cells was similar to that of the SCB-immobilized bacterium BC. The results demonstrated that the bacterium BC also was capable of degrading other TCAs such as TC with satisfactory degradation efficiency. In contrast, in the control group without cell inoculation, only 5.40 ± 0.54% TC reduction was observed, which once again demonstrated that the TCAs were difficult to degrade in a short time in a natural soil environment and the elimination of the TCAs was due to microbial degradation rather than abiotic activity.65 Comparing the degradation efficiency of OTC and TC, TC was more degradable than OTC under identical experimental conditions. Some previous studies are strongly in support of these findings in the present research. For instance, according to Chen et al., the three TCs including chlortetracycline (CTC), tetracycline (TC), and oxytetracycline (OTC) exhibited similar structures and similar physicochemical properties but their degradation efficiency in biological systems such as A/O moving-bed biofilm (MBBR) reactor system varied greatly.90 Under the same reaction conditions, when mixed TCs (50 μg L−1) were added to the MBBR reactor system, the removal efficiencies of CTC, TC, and OTC reached 52.03, 41.79, and 38.42%, respectively, indicating that under the same reaction conditions, the MBBR reactor system degraded CTC most effectively, followed by TC, while OTC was degraded the least effectively.90 The degradation efficiencies of the three TCs in MBBR reactor systems followed the order of CTC > TC > OTC in a previous study.90 Based on the results, they speculated that the degradation efficiencies of OTC, CTC, and TC seemed to be inversely related to the complexity of their molecular structures.90 They speculated that the difference in the biodegradation efficiency among CTC, TC, and OTC might be due to the fact that the hydroxyl group on the third benzene of OTC was prone to form a hydrogen bond with the ortho amino group, thereby making it relatively difficult to hydrolyze. In addition, the chlorine atom on the first benzene ring of CTC was particularly prone to electrophilic substitution, which could decompose the benzene ring into smaller molecular groups.90
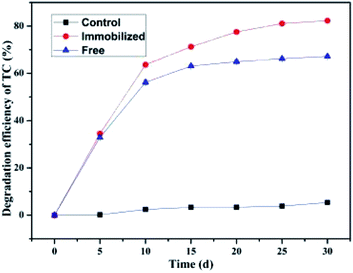 |
| Fig. 10 Microbial degradation of TC in soil by the freely suspended cells or immobilized cells. | |
3.10 Dual-substrate co-biodegradation behaviors of OTC and TC
3.10.1 Effect of low-concentration TC on the biodegradation potential of OTC.
The effect of low-concentration TC on the maximum mean OTC degradation efficiency is presented in Fig. 11. It was found that the low-concentration TC with concentrations of 10 mg kg−1 and 20 mg kg−1 exerted a marginal influence on the maximum biodegradation potential of OTC (100 mg kg−1) by the SCB-immobilized bacterium BC. In the experimental group with 10 mg kg−1 of TC, OTC was degraded at a high degradation velocity of 4.17 mg kg−1 d−1 on the 15th d post-inoculation, which was higher than that in the experimental group with 10 mg kg−1 of TC (3.79 mg kg−1 d−1) and without TC (3.95 mg kg−1 d−1). This may be because TC is more degradable than OTC under identical experimental conditions;90 thus, TC was always preferentially utilized and degraded prior to OTC. Moreover, the addition of TC at low concentrations (10 mg kg−1) enhanced the drug resistance of bacterium BC and thus increased the bioaugmentation capability the of SCB-immobilized bacterium BC in the early stages of incubation, which was supported by previous research showing that the addition of TC at low concentrations (1–10 μg L−1) enhanced the drug resistance of the bacteria and thus increased the removal rate of the reactor.90 However, after a month's incubation, the average OTC degradation efficiency without the existence of TC (79.2 ± 0.32%) was higher than that with the existence of TC at 10 mg kg−1 (77.97 ± 0.93%) and 20 mg kg−1 (76.34 ± 0.53%). This could be explained by carbon catabolite repression, which is a general regulatory phenomenon.91 After the introduced bacteria adapted to the preferred (i.e., rapidly biodegradable) carbon as well as energy sources, the synthesis of the enzymes involved in utilizing the other carbon as well as the energy sources would be inhibited.91 Previous studies have found that background organic compounds are capable of providing microbes with additional sources of energy, nutrients, and growth factors but may also trigger carbon catabolite repression.91 They also demonstrated that the background substrates that promoted biomass growth lowered the biotransformation rate of TC by a novel bacterial strain Stenotrophomonas maltophilia DT1 (ref. 91) in the dual-substrate system because TC was more degradable than OTC under identical experimental conditions. Thus, TC was preferentially degraded by microorganisms, i.e., TC served as the background substrate, which would promote biomass growth and then lower the OTC biodegradation. Thus, the presence of TC at low concentrations contributed to substantial biomass growth but simultaneously led to a decline in the OTC biodegradation efficiency of the SCB-immobilized bacterium BC.
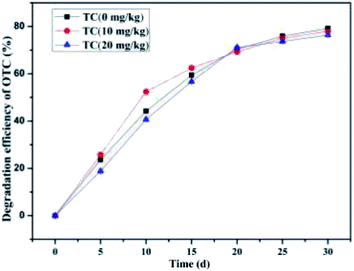 |
| Fig. 11 Effect of low-concentration TC on the biodegradation potential of OTC. | |
3.10.2 Effect of TC on the OTC degradation velocity.
As illustrated in Fig. 12, the SCB-immobilized bacterium BC could still degrade OTC even in the presence of TC (from 60 mg kg−1 to 140 mg kg−1). With the increase in the TC concentration from 60 mg kg−1 to 140 mg kg−1, some phenomena in such dual-substrate co-biodegradation systems exhibited enormous sophistication. To begin with, as the TC concentration increased from 60 mg kg−1 to 140 mg kg−1 with constant OTC concentration (100 mg kg−1), the average OTC degradation efficiencies of 73.96 ± 0.55%, 72.25 ± 0.87%, 72.04 ± 0.52%, 69.56 ± 0.65%, and 64.45 ± 1.11% were achieved within a month's incubation, respectively. Next, as the TC concentration increased from 60 mg kg−1 to 140 mg kg−1 compared to that without TC, the degradation velocity of OTC markedly declined from 2.65 mg kg−1 d−1 to 2.43 mg kg−1 d−1, 2.34 mg kg−1 d−1, 2.31 mg kg−1 d−1, 2.22 mg kg−1 d−1, and 2.01 mg kg−1 d−1, respectively. These results indicated that the influence of TC concentration on the OTC degradation velocity was getting more and more remarkable along with increasing TC concentration in the dual-substrate biodegradation systems. Moreover, it was found that as the TC concentration increased from 60 to 140 mg kg−1, the average TC degradation efficiencies of 50.98 ± 0.75%, 62.63 ± 0.83%, 67.59 ± 0.63%, 66.58 ± 0.39%, and 69.16 ± 0.46% were achieved within a month's incubation, respectively (Fig. 13). These results indicated that as the TC concentration increased from 60 mg kg−1 to 140 mg kg−1 with constant OTC concentration (100 mg kg−1), i.e., as the total antibiotic concentration increased, the OTC degradation efficiency decreased gradually, while the TC degradation efficiency still exhibited a slow rise tendency. The former was consistent with a previous study showing that with the increase in the antibiotic concentration, the degradation of each antibiotic was impaired.90 Nevertheless, with the increase in the total antibiotic concentration, the TC degradation efficiency increased gradually and the efficiency of total antibiotic degradation first increased (TC concentration increased from 60 mg kg−1 to 100 mg kg−1) and then decreased gradually (TC concentration increased from 100 mg kg−1 to 140 mg kg−1). These findings may be due to the following reasons. On the one hand, TC is more degradable than OTC under identical experimental conditions;90 thus, TC is always preferentially utilized and degraded prior to OTC. Simultaneously, with the increase in the TC concentration, more TC is accessible for the biomass,63 thereby enhancing the TC degradation efficiency. On the other hand, since OTC was the intermediate products of TC,92,93 is preferentially consumed, and TC biodegradation is always dominant, the degradation of TC would produce the intermediate product OTC, which meant that OTC was continuously introduced into the system during bioremediation treatment, which further lead to the decline in the OTC degradation efficiency.
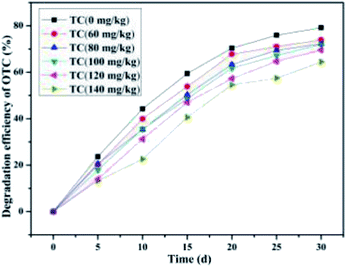 |
| Fig. 12 Effect of TC on the OTC degradation velocity. | |
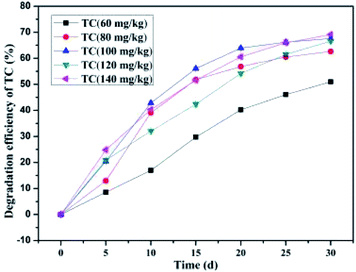 |
| Fig. 13 Degradation efficiency of TC in dual-substrate biodegradation systems. | |
4 Conclusions
The novel bacterium Burkholderia cepacia (BC) was successfully isolated and identified, which could degrade OTC efficiently with a high degradation efficiency (79.20 ± 0.32%) and could also degrade other TCAs such as TC (82.31 ± 0.62%). The present study confirmed the effectiveness of porous sugarcane bagasse particles as an immobilization support matrix and the effectiveness and feasibility of the bioaugmentation capability of the bacterium BC immobilized on sugarcane bagasse (SCB) for the degradation of tetracycline antibiotics (TCAs) in soil. The SCB-immobilized bacterium BC exhibited better performance than the freely suspended bacterium BC with regard to the elimination of OTC in soil under identical experimental conditions. The inoculation dose of 15% (v/w), 28–43 °C, slightly acidic pH (4.5–6.5), and the addition of OTC (from 80 mg kg−1 to 160 mg kg−1) favored the bioaugmentation capability of the bacterium BC, indicating its strong tolerance to high temperature, pH, and high substrate concentrations, which would be a clear benefit in the actual practical application. However, the inhibition of the substrate OTC to the bacterium BC was observed at a higher OTC concentration (180 mg kg−1). In addition, the SCB-immobilized bacterium BC system exhibited a strong tolerance to heavy metals ions such as Pb2+ and Cd2+, and could fit into the simulated soil environment very well. It was found that the co-culture of bacterium BC and bacterium PN could significantly improve the biodegradation efficiency for the degradation of OTC.
In dual-substrate co-biodegradation, it was found that the presence of TC at low concentration contributed to substantial biomass growth but simultaneously led a decline in the OTC biodegradation efficiency in the SCB-immobilized bacterium BC. As the total antibiotic concentration was increased, the OTC degradation efficiency decreased gradually, while the TC degradation efficiency still exhibited a slow rise tendency. These could be because TC was more degradable than OTC under identical conditions; thus, TC was always preferentially utilized and degraded prior to OTC, which thereby continuously introduced OTC into the system during the bioremediation treatment.
Taking all the observations together, we conclude that the SCB-immobilized bacterium BC system exhibited great potential in the remediation of TCAs-contaminated environments. However, assessing the potential biotransformation of the targeted contaminant in soil requires careful consideration of a wide variety of factors that cover bioavailability change and the accumulation of toxic transformation intermediates or biodegradation products during the bioremediation treatment. However, the objective of bioremediation should not be only achieving mass reduction but risk reduction and management as well. As such, it is important to consider these aspects in future research.
Conflicts of interest
There are no conflicts of interest to declare.
Acknowledgements
This project was supported by the Science and Technology Planning Project of Guangdong Province of China (No. 4900E17064).
References
- F. Liu, X. Zhang, Y. Li, H. Gao, P. Ling, X. Li, Q. Chen, A. Ma, H. Shao, M. Li and F. Wang, Chromatographia, 2017, 81(2), 303–314 CrossRef.
- Y. Wang, T. Xie, J. Yang, M. Lei, J. Fan, Z. Meng, M. Xue, L. Qiu, F. Qi and Z. Wang, Anal. Chim. Acta, 2019, 1070, 97–103 CrossRef CAS PubMed.
- D. Moreno-González and A. M. García-Campaña, Food Chem., 2017, 221, 1763–1769 CrossRef PubMed.
- N. Alavi, A. A. Babaei, M. Shirmardi, A. Naimabadi and G. Goudarzi, Environ. Sci. Pollut. Res., 2015, 22(22), 17948–17954 CrossRef CAS PubMed.
- A. Y. Udalova, S. G. Dmitrienko and V. V. Apyari, J. Anal. Chem., 2015, 70(6), 661–676 CrossRef CAS.
- D. Uriarte, C. Domini and M. Garrido, Talanta, 2019, 201, 143–148 CrossRef CAS PubMed.
- W. Guerra, P. P. Silva-Caldeira, H. Terenzi and E. C. Pereira-Maia, Coord. Chem. Rev., 2016, 327–328, 188–199 CrossRef CAS.
- Y. Chen, Q. Wu, N. Bu, J. Wang and Y. Song, Chem. Eng. J., 2019, 373, 192–202 CrossRef CAS.
- J.-R. Yeom, S.-U. Yoon and C.-G. Kim, Chemosphere, 2017, 182, 771–780 CrossRef CAS PubMed.
- X. Zhao, Y. Li, L. Yang, X. Wang, Z. Chen and J. Shen, Clean: Soil, Air, Water, 2018, 46(7), 1700411, DOI:10.1002/clen.201700411.
- H. Xu, H.-Y. Mi, M.-M. Guan, H.-Y. Shan, Q. Fei, Y.-F. Huan, Z.-Q. Zhang and G.-D. Feng, Food Chem., 2017, 232, 198–202 CrossRef CAS PubMed.
- Y. Zhang, S. A. Boyd, B. J. Teppen, J. M. Tiedje, W. Zhang, D. Zhu and H. Li, Environ. Pollut., 2018, 243, 1078–1086 CrossRef CAS PubMed.
- S. Aydin, Appl. Microbiol. Biotechnol., 2016, 100(14), 6491–6499 CrossRef CAS PubMed.
- Z. Cetecioglu, B. Ince, S. Azman and O. Ince, Appl. Biochem. Biotechnol., 2013, 172(2), 631–640 CrossRef PubMed.
- C. Roose-Amsaleg, C. Yan, A.-M. Hoang and A. M. Laverman, Ecotoxicology, 2013, 22(10), 1467–1478 CrossRef CAS PubMed.
- Q. Wang, X. Li, Q. Yang, Y. Chen and B. Du, Ecotoxicol. Environ. Saf., 2019, 171, 746–752 CrossRef CAS PubMed.
- L. Xu, H. Li, W. A. Mitch, S. Tao and D. Zhu, Environ. Sci. Technol., 2018, 53, 710–718 CrossRef PubMed.
- J. D. Orwa, J. W. Matofari, P. S. Muliro and P. Lamuka, Int. J. Food Contam., 2017, 4(1) DOI:10.1186/s40550-017-0050-1.
- D. Guo, L. Ni, L. Wang and L. Shao, Chirality, 2019 DOI:10.1002/chir.23080.
- N. Xu, L. Meng, H.-W. Li, D.-Y. Lu and Y. Wu, Microchim. Acta, 2018, 185(6) DOI:10.1007/s00604-018-2828-0.
- H. Fang, L. Han, H. Zhang, Z. Long, L. Cai and Y. Yu, J. Hazard. Mater., 2018, 357, 53–62 CrossRef CAS PubMed.
- F. Zhao, L. Yang, L. Chen, S. Li and L. Sun, Environ. Sci. Pollut. Res., 2018, 25, 34063–34075 CrossRef CAS PubMed.
- J. Sun, Q. Zeng, D. C. W. Tsang, L. Z. Zhu and X. D. Li, Chemosphere, 2017, 189, 301–308 CrossRef CAS PubMed.
- Y.-W. Li, X.-L. Wu, C.-H. Mo, Y.-P. Tai, X.-P. Huang and L. Xiang, J. Agric. Food Chem., 2011, 59(13), 7268–7276 CrossRef CAS PubMed.
- M. Conde-Cid, C. Álvarez-Esmorís, R. Paradelo-Núñez, J. C. Nóvoa-Muñoz, M. Arias-Estévez, E. Álvarez-Rodríguez, M. J. Fernández-Sanjurjo and A. Núñez-Delgado, J. Cleaner Prod., 2018, 197, 491–500 CrossRef CAS.
- M. Pan and L. M. Chu, Sci. Total Environ., 2017, 599–600, 500–512 CrossRef CAS PubMed.
- M. Pan and L. M. Chu, Sci. Total Environ., 2016, 545–546, 48–56 CrossRef CAS PubMed.
- X. Liu, Y. Lv, K. Xu, X. Xiao, B. Xi and S. Lu, Chemosphere, 2018, 201, 137–143 CrossRef CAS PubMed.
- M. Pan and L. M. Chu, Ecotoxicol. Environ. Saf., 2016, 126, 228–237 CrossRef CAS PubMed.
- D. Zhu, X.-L. An, Q.-L. Chen, X.-R. Yang, P. Christie, X. Ke, L.-H. Wu and Y.-G. Zhu, Environ. Sci. Technol., 2018, 52(5), 3081–3090 CrossRef CAS PubMed.
- F. Zhao, L. Yang, L. Chen, Q. Xiang, S. Li, L. Sun, X. Yu and L. Fang, J. Environ. Sci., 2019, 79, 200–212 CrossRef PubMed.
- Z. Chen, Y. Zhang, Y. Gao, S. A. Boyd, D. Zhu and H. Li, Environ. Sci. Technol., 2015, 49(18), 10903–10910 CrossRef CAS PubMed.
- H. Li, B. Li, J. Ma, J. Ye, P. Guo and L. Li, Chem. Eng. J., 2018, 337, 584–594 CrossRef CAS.
- H. Liu, H. Sun, M. Zhang and Y. Liu, J. Environ. Manage., 2019, 238, 84–91 CrossRef CAS PubMed.
- H. Xia, J. Chen, X. Chen, K. Huang and Y. Wu, Environ. Pollut., 2019, 252, 1068–1077 CrossRef CAS PubMed.
- J. Ma, D. Zhu, G. D. Sheng, P. O'Connor and Y.-G. Zhu, Sci. Total Environ., 2019, 673, 357–366 CrossRef CAS PubMed.
- F. Zhao, L. Chen, L. Yang, S. Li, L. Sun and X. Yu, Chemosphere, 2018, 211, 261–270 CrossRef CAS PubMed.
- R. Wei, F. Ge, L. Zhang, X. Hou, Y. Cao, L. Gong, M. Chen, R. Wang and E. Bao, Chemosphere, 2016, 144, 2377–2383 CrossRef CAS PubMed.
- J. Ma, Y. Lei, M. A. Khan, F. Wang, Y. Chu, W. Lei, M. Xia and S. Zhu, Int. J. Biol. Macromol., 2018, 124, 557–567 CrossRef PubMed.
- J. Wan, P. Xue, R. Wang, L. Liu, E. Liu, X. Bai, J. Fan and X. Hu, Appl. Surf. Sci., 2019, 483, 677–687 CrossRef CAS.
- Y. Zhao, C. Huang, X. Ma, F. Chen, B. Liang and A. Wang, Bioresource Technology Reports, 2019, 7, 100192 CrossRef.
- N. Panigrahy, M. Barik, R. K. Sahoo and N. K. Sahoo, Int. Biodeterior. Biodegrad., 2020, 147, 104837 CrossRef CAS.
- D. Yalaoui-Guellal, S. Fella-Temzi, S. Djafri-Dib, F. Brahmi, I. M. Banat and K. Madani, J. Pet. Sci. Eng., 2019, 184 DOI:10.1016/j.petrol.2019.106554.
- A. S. Nwankwegu and C. O. Onwosi, Environ. Technol. Innovation, 2017, 7, 1–11 CrossRef.
- B. Basak, B.-H. Jeon, M. B. Kurade, G. D. Saratale, B. Bhunia, P. K. Chatterjee and A. Dey, Ecotoxicol. Environ. Saf., 2019, 180, 317–325 CrossRef CAS PubMed.
- H. Yun, B. Liang, J. Qiu, L. Zhang, Y. Zhao, J. Jiang and A. Wang, Environ. Sci. Technol., 2016, 51(1), 291–300 CrossRef PubMed.
- C.-F. Yang, S.-H. Liu, Y.-M. Su, Y.-R. Chen, C.-W. Lin and K.-L. Lin, Sci. Total Environ., 2019, 648, 811–818 CrossRef CAS PubMed.
- F. Anasonye, E. Winquist, M. Räsänen, J. Kontro, K. Björklöf, G. Vasilyeva, K. S. Jørgensen, K. T. Steffen and M. Tuomela, Int. Biodeterior. Biodegrad., 2015, 105, 7–12 CrossRef CAS.
- J. Kaushal, S. Mehandia, G. Singh, A. Raina and S. K. Arya, Biocatal. Agric. Biotechnol., 2018, 16, 192–199 CrossRef.
- S. Shao, Y. Hu, J. Cheng and Y. Chen, Ecotoxicol. Environ. Saf., 2019, 171, 833–842 CrossRef CAS PubMed.
- S. Shao, Y. Hu, J. Cheng and Y. Chen, Chem. Eng. J., 2018, 354, 758–766 CrossRef CAS.
- S. Shao, Y. Hu, C. Chen, J. Cheng and Y. Chen, Chemosphere, 2018, 209, 35–43 CrossRef CAS PubMed.
- Z. Mu, Z. Zou, Y. Yang, W. Wang, Y. Xu, J. Huang, R. Cai, Y. Liu, Y. Mo, B. Wang, Y. Dang, Y. Li, Y. Liu, Y. Jiang, Q. Tan, X. Liu, C. Hu, H. Li, S. Wei, C. Lou, Y. Yu and J. Wang, Synthetic and Systems Biotechnology, 2018, 3(3), 196–203 CrossRef PubMed.
- A. Mazumder, S. Das, D. Sen and C. Bhattacharjee, Environ. Technol. Innovation, 2020, 100630, DOI:10.1016/j.eti.2020.100630.
- B. Liang, H. Yun, D. Kong, Y. Ding, X. Li, A. S. Vangnai and A. Wang, Environ. Res., 2020, 180, 108840, DOI:10.1016/j.envres.2019.108840.
- L. Huang, W. Wang, S. B. Shah, H. Hu, P. Xu and H. Tang, J. Hazard. Mater., 2019, 120833, DOI:10.1016/j.jhazmat.2019.120833.
- H. Wu, J. Shen, X. Jiang, X. Liu, X. Sun, J. Li, W. Han, Y. Mu and L. Wang, Bioresour. Technol., 2018, 264, 98–105 CrossRef CAS PubMed.
- H. Sun, L. Zhu and D. Zhou, Environ. Sci. Pollut. Res., 2017, 25(1), 1–3 CrossRef PubMed.
- J. Zdarta, K. Jankowska, M. Wyszowska, E. Kijeńska-Gawrońska, A. Zgoła-Grześkowiak, M. Pinelo, A. S. Meyer, D. Moszyński and T. Jesionowski, Mater. Sci. Eng., C, 2019, 103 DOI:10.1016/j.msec.2019.109789.
- K. Tao, X. Zhang, X. Chen, X. Liu, X. Hu and X. Yuan, Chemosphere, 2019, 222, 831–838 CrossRef CAS PubMed.
- Z. Z. Ismail and H. A. Khudhair, Biochem. Eng. J., 2018, 131, 17–23 CrossRef CAS.
- C.-H. Chen, L.-M. Whang, C.-L. Pan, C.-L. Yang and P.-W. G. Liu, Int. Biodeterior. Biodegrad., 2017, 124, 62–72 CrossRef CAS.
- B. Ruan, P. Wu, M. Chen, X. Lai, L. Chen, L. Yu, B. Gong, C. Kang, Z. Dang, Z. Shi and Z. Liu, Ecotoxicol. Environ. Saf., 2018, 162, 103–111 CrossRef CAS PubMed.
- B. Li, L. Gan, G. Owens and Z. Chen, Water Res., 2018, 146, 55–66 CrossRef CAS PubMed.
- Z. LI, W. QI, Y. FENG, Y. LIU, S. Ebrahim and J. LONG, J. Integr. Agric., 2019, 18(9), 1953–1960 CrossRef CAS.
- L. Pan, X. Feng, M. Cao, S. Zhang, Y. Huang, T. Xu, J. Jing and H. Zhang, RSC Adv., 2019, 9(28), 15686–15693 RSC.
- M. Harrabi, D. A. M. Alexandrino, F. Aloulou, B. Elleuch, B. Liu, Z. Jia, C. M. R. Almeida, A. P. Mucha and M. F. Carvalho, Sci. Total Environ., 2018, 648, 962–972 CrossRef PubMed.
- L. Lian, J. Lv, X. Wang and D. Lou, J. Chromatogr. A, 2018, 1534, 1–9 CrossRef CAS PubMed.
- K. Wang, K. Lin, X. Huang and M. Chen, A Simple and Fast Extraction Method for the Determination of Multiclass Antibiotics in Eggs Using LC-MS/MS, J. Agric. Food Chem., 2017, 65(24), 5064–5073 CrossRef CAS PubMed.
- D. Chen, J. Yu, Y. Tao, Y. Pan, S. Xie, L. Huang, D. Peng, X. Wang, Y. Wang, Z. Liu and Z. Yuan, J. Chromatogr. B: Anal. Technol. Biomed. Life Sci., 2016, 1017–1018, 82–88 CrossRef CAS PubMed.
- C.-X. Liu, Q.-M. Xu, S.-C. Yu, J.-S. Cheng and Y.-J. Yuan, Sci. Total Environ., 2020, 710 DOI:10.1016/j.scitotenv.2019.136329.
- S. Murshid and G. P. Dhakshinamoorthy, Int. Biodeterior. Biodegrad., 2019, 144, 104756, DOI:10.1016/j.ibiod.2019.104756.
- L. Cao, J. Zhang, R. Zhao, Y. Deng, J. Liu, W. Fu, Y. Lei, T. Zhang, X. Li and B. Li, Environ. Int., 2019, 131, 104961, DOI:10.1016/j.envint.2019.104961.
- T. Song, S. Li, Y. Lu, D. Yan, P. Sun, M. Bao and Y. Li, Chemosphere, 2019, 231, 184–193 CrossRef CAS PubMed.
- J. Tang, X. Rong, D. Jin, C. Gu, A. Chen and S. Luo, Int. Biodeterior. Biodegrad., 2020, 147, 104867, DOI:10.1016/j.ibiod.2019.104867.
- Y. Wang, W. Zhan, Q. Ren, S. Cheng, J. Wang, X. Ma, C. Zhang and Y. Wang, Sci. Total Environ., 2019, 689, 645–651 CrossRef CAS PubMed.
- M. Wang, C. Cai, B. Zhang and H. Liu, Chemosphere, 2018, 193, 611–617 CrossRef CAS PubMed.
- B. B. Hameed and Z. Z. Ismail, Biochem. Eng. J., 2018, 137, 71–77 CrossRef CAS.
- O. S. Obayori, L. B. Salam, G. O. Oyetibo, M. Idowu and O. O. Amund, Biocatal. Agric. Biotechnol., 2017, 12, 78–84 CrossRef.
- M. K. Corbett, J. J. Eksteen, X.-Z. Niu and E. L. J. Watkin, Res. Microbiol., 2018, 169, 558–568 CrossRef CAS PubMed.
- M. Xu, Z. Zhao, Y. Song, J. Li, Y. You and J. Li, Geoderma, 2020, 361, 114131, DOI:10.1016/j.geoderma.2019.114131.
- S. Singh, V. Kumar, G. Kaur Sidhu and J. Singh, J. Environ. Chem. Eng., 2019, 7, 103098, DOI:10.1016/j.jece.2019.103098.
- W. Qin, F. Fan, Y. Zhu, X. Huang, A. Ding, X. Liu and J. Dou, Braz. J. Microbiol., 2018, 49(2), 258–268 CrossRef CAS PubMed.
- Z. Liu, Q. Liu, X. Qi, Y. Li, G. Zhou, M. Dai, M. Miao and Q. Kong, Sci. Total Environ., 2019, 694, 133722, DOI:10.1016/j.scitotenv.2019.133722.
- Z. Jiang, X. Zhang, Z. Wang, B. Cao, S. Deng, M. Bi and Y. Zhang, Ecotoxicol. Environ. Saf., 2019, 172, 159–166 CrossRef CAS PubMed.
- L. Kachieng’a and M. N. B. Momba, J. Environ. Chem. Eng., 2018, 6(4), 4820–4827 CrossRef.
- A. Kamyabi, H. Nouri and H. Moghimi, Ecotoxicol. Environ. Saf., 2018, 163, 471–477 CrossRef CAS PubMed.
- M. Li, X. M. Zhao, X. F. Zhang, D. Wu and S. Leng, Sci. Rep., 2018, 8(1) DOI:10.1038/s41598-018-22169-0.
- L. Kachieng'a and M. N. B. Momba, J. Environ. Chem. Eng., 2017, 5(2), 1885–1891 CrossRef.
- H.-Y. Chen, Y.-D. Liu and B. Dong, Bioprocess Biosyst. Eng., 2017, 41(1), 47–56 CrossRef PubMed.
- Y. Leng, J. Bao, G. Chang, H. Zheng, X. Li, J. Du, D. Snow and X. Li, J. Hazard. Mater., 2016, 318, 125–133 CrossRef CAS PubMed.
- K. Sun, Q. Huang and S. Li, J. Hazard. Mater., 2017, 331, 182–188 CrossRef CAS PubMed.
- Z. Yin, D. Xia, M. Shen, D. Zhu, H. Cai, M. Wu, Q. Zhu and Y. Kang, Chemosphere, 2020, 253, 126729, DOI:10.1016/j.chemosphere.2020.126729.
|
This journal is © The Royal Society of Chemistry 2020 |