DOI:
10.1039/D0RA06238C
(Paper)
RSC Adv., 2020,
10, 33080-33085
The cation–anion co-exchange in CsPb1−xFex(Br1−yCly)3 nanocrystals prepared using a hot injection method
Received
17th July 2020
, Accepted 31st August 2020
First published on 7th September 2020
Abstract
All inorganic perovskite nanocrystals (NCs) have wide practical applications for their remarkable optoelectronic properties. To obtain blue-emitting perovskites with high photoluminescence quantum yield and room-temperature ferromagnetism, CsPb1−xFex(Br1−yCly)3 NCs were synthesized using a hot injection method. The effects of the cation–anion co-exchange on the structural, luminescent and magnetic properties of CsPbBr3 NCs were studied by X-ray diffraction spectroscopy, photoluminescence spectroscopy, transmission electron microscopy, field emission scanning electron microscopy, and vibrating sample magnetometer. The results indicated that there was cation–anion co-exchange in CsPb1−xFex(Br1−yCly)3 NCs, while the band-edge energies and PLQY were mainly affected by the anion exchange. The ferromagnetism of CsPb1−xFex(Br1−yCly)3 NCs had been observed at room temperature, and there was an increase in saturation magnetization with increasing Fe concentration.
1. Introduction
Since the all-inorganic perovskite (CsPbX3, X = Cl, Br, and I) nanocrystals (NCs) with narrow photoluminescence (PL), high photoluminescence quantum yield (PLQY), and high crystallinity were prepared firstly by Protesescu and co-workers in 2015, more and more attention has been on cesium based perovskite NCs.1–9 The PLQYs of CsPbBr3 and CsPbI3 NCs are beyond 90% in the green and red spectral ranges, respectively, while that of the blue-emitting NCs is still low.10–14 Lots of researchers have attempted to solve this question. For example, Ye et al. prepared CsPbBr3 quantum dots with a blue PLQY of 68% through controlling the size of NCs. It is unfortunate that the small size (3 nm) caused the agglomeration of the perovskite NCs.15 At the same time, Zeng et al. obtained blue-emitting CsPbBr3 NCs with approximately 100% PLQY, however, it was difficult to cover the whole blue spectrum range.16 In fact, the optical properties of perovskite NCs can be easily tuned by the individual halide anion exchange to meet the requirements of the new material in optoelectronic applications.17–22 Thus, the mixed-halide perovskite CsPb(Br1−xClx)3 NCs can overcome those shortcomings by varying the atomic proportions of Br and Cl, to make their emission wavelength cover the blue spectrum range.
On the other hand, cation doping has been demonstrated as one powerful method for enhancing PLQY. For example, Song et al. improved PLQY of the CsPbCl3 quantum dots from 3.2 to 10.3% by doping K+.23 Zhao et al. prepared Mn-doped CsPbCl3 NCs with different Mn concentration prepared in the presence of nickel chloride, which PLQY with orange and red emissions peaked at 600 and 620 nm in hexane were 70% and 39%, respectively.24 In addition, the doping of some cations can enhance or introduce novel functionalities into quantum dots. Tang and co-workers obtained the triboelectric performance enhancement through doping Ba2+ into CsPbBr3 lattice for modulating the microstructures and electrical properties of perovskite films.25 Li et al. observed room-temperature ferromagnetism for Co2+ doped CsPbCl3 NCs, and founded the ferromagnetism increased with the increase of Co2+ content.26 Iron ions are not only environment friendly and low cost, but also have good conductivity. Recently, Pradhan et al. synthesized Fe2+ doped CsPbBr3 perovskite NCs and found that the doped NCs enhanced the catalytic activity compared with undoped CsPbBr3.27 Singh et al.28 and Wang et al.29 prepared Fe3+ and Fe2+ doped CsPbCl3 NCs, respectively. It is interesting that they all observed that Fe-doping not only enhanced PLQY, but also improved the homogeneity of size and cubic shape of NCs. In addition, Singh et al. prepared Fe:CdSe quantum dots and found the material was superparamagnetic behavior with a weak ferromagnetic exchange interaction, which would open a possibility of understanding the controversial origin of magnetism in diluted magnetic semiconductor quantum dots for future spintronic devices.30 In this work, to obtain blue-emitting perovskites with high PLQY and room-temperature ferromagnetism, we prepared CsPb1−xFex(Br1−yCly)3 NCs using a hot injection method. Interesting variations in luminescent and magnetic properties were possible by varying the doping concentration of Fe and Cl. The samples were characterized by various techniques and discussed in detail.
2. Experimental
2.1 Materials
Lead bromide (PbBr2, 99.99%), lead chloride (PbCl2, 99.99%), cesium carbonate (Cs2CO3, 99.99%), iron bromide (FeBr2, 99.99%), iron dichloride (FeCl2, 99.99%) and trioctylphosphine (TOP, 90%) were purchased from Aladdin; 1-octadecene (ODE, 90%) was purchased from Alfa Aesar; oleic acid (OA, 90%) and oleylamine (OLA, 70%) were purchased from Aldrich. All chemicals were used without further purification.
2.2 Synthesis of CsPb1−xFex(Br1−yCly)3 NCs
The Cs-oleate precursor, CsPbBr3 NCs was synthesized according to the method described by Protesescu et al.4 For the synthesis of CsPb1−xFex(Br1−yCly)3 NCs in a typical procedure, the PbBr2 (0.2 mmol), FeCl2 (α mmol), OLA (1.5 mL), OA (1.5 mL), TOP (1 mL), and ODE (5 mL) were mixed in a 50 mL three-neck round-bottomed flask. After degassed at 110 °C for 20 min, the reaction mixture was heated up to 190 °C under argon flow. 1 mL Cs-oleate precursor was then rapidly injected, and after 15 s, the reaction mixture was cooled by an ice-water bath. The obtained solution was centrifuged at 5000 rpm for 5 min, the supernatant was discarded and the particles were dispersed in hexane and centrifuged again to remove the residual reaction mixture. The NCs obtained with the mole ratios of PbBr2 and FeCl2 were 1
:
0, 1
:
0.5.1
:
1, 1
:
2, and 1
:
3, respectively.
2.3 Characterization
The actual doping concentration of Fe, structure and morphology were characterized by an inductively coupled plasma mass spectrometer (ICP-MS, PerkinElmer Nexion 350-X), X-ray diffraction (XRD, Rigaku D/max-2500/PC) with CuKα radiation, transmission electron microscopy (TEM, JEOL-JEM-2100), field emission scanning electron microscopy (FE-SEM, JEOL JSE-7800F). UV-visible absorption spectra, the steady-state and time-resolved fluorescence spectra an absolute photoluminescence quantum yield were recorded by Shimadzu UV-2700 spectrophotometer, a Horiba Jobin Yvon fluorologo-3 fluorescence spectrometer and Otsuka QE-2000. The magnetic properties were measured by a vibrating sample magnetometer (VSM, Lake Shore 7407).
3. Results and discussion
Table 1 lists the actual doping concentration of Fe relative to Pb for the CsPb1−xFex(Br1−yCly)3 NCs. It reveals a growth in Fe concentration with increasing FeCl2 concentration. In addition, the values of x in the chemical formula CsPb1−xFex(Br1−yCly)3 are listed in Table 1.
Table 1 The actual Fe doping concentration and the values of x for the NCs
PbBr2/FeCl2 |
1/0 |
1/0.5 |
1/1 |
1/2 |
1/3 |
Fe concentration |
0% |
1.5% |
3.6% |
8.1% |
11.5% |
x |
0 |
0.01 |
0.03 |
0.07 |
0.10 |
Fig. 1 gives the XRD patterns of CsPb1−xFex(Br1−yCly)3 NCs. It is obviously observed there are two strong diffraction peaks at 14.47° and 30.08° for the NCs with PbBr2/FeCl2 being 1/0, which correspond to (100) an (200) directions of CsPbBr3, respectively. It is the typical cubic structure, being consistent with the standard card (PDF#54-0752). With increasing FeCl2 concentration, the diffraction peaks shift obviously to a larger angle, which is due to the exchange of the large ionic radius of Br− (1.820 nm) to the smaller Cl− (1.670 nm) and Pb2+ (0.119 nm) to Fe2+ (0.078 nm). For the NCs with PbBr2/FeCl2 being 1/3, there are two new peaks at 13.79 °and 16.79° in the XRD pattern. According to the standard card, they are the impurity phases FeBr2 (PDF#15-0829) and Cs3Pb6.48Cl16 (PDF#45-1243). Overall, a certain amount of Cl− and Fe2+ can dope in the CsPbBr3 NCs and the structure is not damaged.
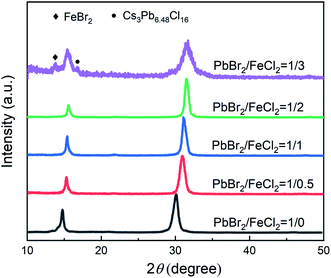 |
| Fig. 1 XRD patterns of CsPb1−xFex(Br1−yCly)3 NCs. | |
Fig. 2(a–e) is the EDAX spectrum of CsPb1−xFex(Br1−yCly)3 NCs. There are only Cs, Pb and Br elements in the Fig. 2(a), and the atomic ratio of Cs
:
Pb
:
Br is 1.2
:
0.9
:
3.0, indicating the CsPbBr3 NCs having been formed. With increasing FeCl2 concentration, Fe and Cl elements appear except for Cs, Pb and Br (seeing Fig. 2(b–e)).
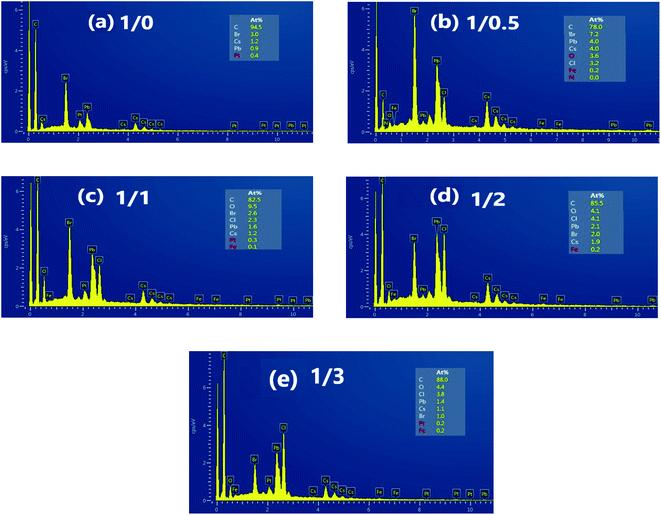 |
| Fig. 2 EDAX spectrum of CsPb1−xFex(Br1−yCly)3 NCs with PbBr2/FeCl2 being (a) 1/0, (b) 1/0.5, (c) 1/1, (d) 1/2, and (e) 1/4 (inset: the atomic ratios of the elements). | |
Table 2 shows the values of the atomic ratio Cl/Br and y for CsPb1−xFex(Br1−yCly)3 NCs from the EDAX spectrum. With increasing FeCl2 concentration, Cl/Br and y increase from 0 to 3.8 and 0 to 0.79, respectively. It is worth noting that the value of Cl/Br is larger than that of Fe/Pb obtained from ICP-MS for all NCs, showing there being a relatively large reactivity of the anion exchange compared to the cation exchange. The similar result was reported for Ni doping in CsPbBr3 NCs,31 which may be due to the halide and counteraction rich surfaces.32 The chemical formulas for those NCs are shown in Table 2. For example, when the PbBr2/FeCl2 is 1/0.5, the actual values of Fe/Pb and Cl/Br are 1.5% and 0.44, respectively, and the chemical formula is CsPb0.99Fe0.01(Br0.69Cl0.31)3.
Table 2 The values of Cl/Br and y from the EDAX spectrum, and the chemical formulas for CsPb1−xFex(Br1−yCly)3 NCs
PbBr2/FeCl2 |
Cl/Br |
y |
CsPb1−xFex(Br1−yCly)3 |
1/0 |
0 |
0 |
CsPbBr3 |
1/0.5 |
0.44 |
0.31 |
CsPb0.99Fe0.01(Br0.69Cl0.31)3 |
1/1 |
0.88 |
0.47 |
CsPb0.97Fe0.03(Br0.53Cl0.47)3 |
1/2 |
2.05 |
0.67 |
CsPb0.93Fe0.07(Br0.33Cl0.67)3 |
1/3 |
3.8 |
0.79 |
CsPb0.90Fe0.10(Br0.21Cl0.79)3 |
Typical TEM images of CsPb1−xFex(Br1−yCly)3 NCs with PbBr2/FeCl2 being 1/0, 1/1, and 1/3 are shown in Fig. 3(a–c). In Fig. 3(a), the undoped CsPbBr3 NCs have a cubic morphology with an average cube length of 8.3 nm showing homogeneous distribution. When PbBr2/FeCl2 is 1/1, the size (8.5 nm) and the dispersity these NCs remain identical, as displayed in Fig. 3(b). However, in Fig. 3(c), for the NCs with PbBr2/FeCl2 being 1/3, the size decreases to 7.4 nm, and the homogeneous distribution is destroyed obviously. Thus, a larger amount of Fe2+ doped would damage the dispersity for CsPb1−xFex(Br1−yCly)3 NCs. The similar results have been obtained by Pradhan et al.27 and Wang et al.29 Fig. 3(d) is the HRTEM image of CsPb1−xFex(Br1−yCly)3 NCs with PbBr2/FeCl2 being 1/1. It can be seen that the lattice spacing observed articulately demonstrates the good crystallinity of the synthesized NCs.
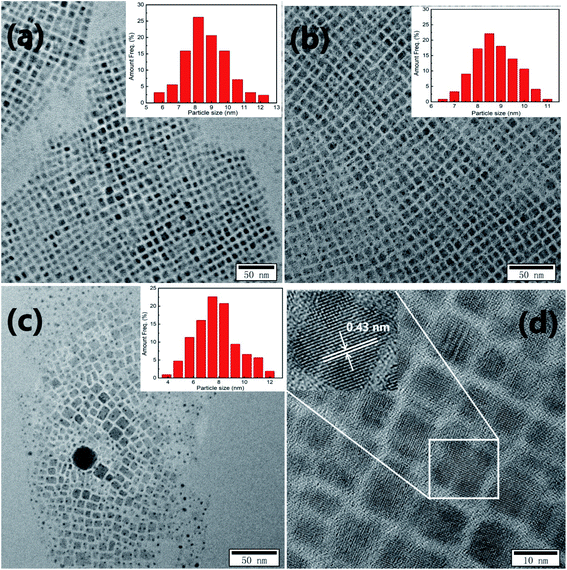 |
| Fig. 3 TEM images of CsPb1−xFex(Br1−yCly)3 NCs with PbBr2/FeCl2 being (a) 1/0, (b)1/1, and (c) 1/3 (inset: size distribution), and HRTEM image (d) of NCs with PbBr2/FeCl2 being 1/1 (inset: the high magnification image). | |
Fig. 4(a and b) shows the UV-Visible absorption and steady-state PL spectra of CsPb1−xFex(Br1−yCly)3 NCs. In Fig. 4(a), the exciton absorption band of undoped CsPbBr3 NCs is about 507 nm, and there is a blue shift with increasing FeCl2 concentration. On the other hand, in Fig. 4(b), the exciton PL peak is at 511 with full width at half maximum (FWHM) of 16.7 nm (79.9 meV) for undoped CsPbBr3 NCs. With the cation–anion co-exchange, the peak is located at 477, 454, 431 and 415 nm, with the FWHM of 15.2 nm (82.8 meV), 14.1 nm (85.0 meV), 12.4 nm (82.6 meV) and 11.6 nm (83.6 meV), respectively. The obvious blue shift is caused by the doping of Cl− ions. For the NCs with low Fe doping concentration, the decrease in FWHM may be caused by the reduction of defects, while, for the NCs with high Fe doping concentration, it should be caused by the decrease in size of the NCs. Based on the previous reports for mixed chloride-bromide perovskites,33 those observations in Fig. 4 (a and b) imply there are the cation–anion co-exchange in CsPb1−xFex(Br1−yCly)3 NCs, while the band-edge energies are mainly affected by the anion exchange.
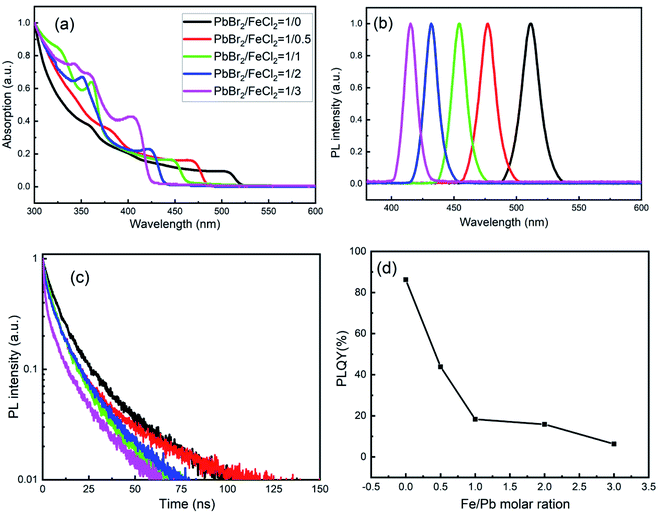 |
| Fig. 4 Absorption (a), PL spectra (b), decay (c) and PLQYs (d) of CsPb1−xFex(Br1−yCly)3 NCs. The solid lines of black, red, green and magenta are corresponding to the NCs with the mole ratios of PbBr2/FeCl2 being 1/0, 1/0.5, 1/1, 1/2, and 1/3, respectively. | |
Fig. 4(c) shows the PL decay curves of CsPb1−xFex(Br1−yCly)3 NCs. All decay curves are fitted by a biexponential function: A(t) = A1
exp(−t/τ1) + A2
exp(−t/τ2). In the equation, τ1 and τ2 are the lifetime constants, while A1 and A2 are the PL amplitudes. The average PL lifetime τav is calculated by formula:34
|
τav = (A1 × τ12) + (A2 × τ22)/(A1 × τ1) + (A2 × τ2)
| (1) |
The values of τ1, τ2, A1, A2 and τav are listed in Table 3. The average PL lifetimes of undoped CsPbBr3 NCs was calculated to be about 16.1 ns. The values are lower than that of CsPbBr3 bulk films (about 100 ns), confirming the excitation recombination nature of CsPbX3 NCs. On codoping of Fe2+ and Cl−, τav decreases compared to the undoped CsPbBr3 NCs, which indicates there are some defects existing in the NCs. And those defects will decrease the PLQY (seeing Fig. 4(d)). In Fig. 4(d), the value of PLQY for the undoped CsPbBr3 NCs is about 86.2%, and then decrease sharply after Fe2+ and Cl− doping. It is known that halide vacancies form shallow trap levels in CsPbBr3 as well as deep trap states in CsPbCl3, which promotes nonradiative relaxation of the carries and lower the PLQY. Thus the decrease in PLQY is mainly affected by the anion exchange.
Table 3 PL lifetimes of CsPb1−xFex(Br1−yCly)3 NCs
PbBr2/FeCl2 |
A1 |
τ1 (ns) |
A2 |
τ2 (ns) |
τav (ns) |
1/0 |
0.652 |
5.4 |
0.312 |
21.7 |
16.1 |
1/0.5 |
0.566 |
3.4 |
0.404 |
13.6 |
11.0 |
1/1 |
0.528 |
2.1 |
0.460 |
13.6 |
11.8 |
1/2 |
0.742 |
1.1 |
0.315 |
12.4 |
10.4 |
1/3 |
0.637 |
2.5 |
0.372 |
9.3 |
7.2 |
Fig. 5 gives the magnetic hysteresis loops measured at room temperature. There is an obvious hysteresis behavior for CsPb1−xFexCl3 NCs. For undoped CsPbBr3 NCs, the saturation magnetization Ms is about 0.14 meum g−1. The small ferromagnetism may be caused by the vacancies and the defects in the NCs.35 With increasing the concentration of Fe, Ms increases monotonically. The ferromagnetism of CsPb1−xFex(Br1−yCly)3 NCs should be attributed to the doping of Fe2+ in the lattice as a substituent for carrier-induced ferromagnetism.36
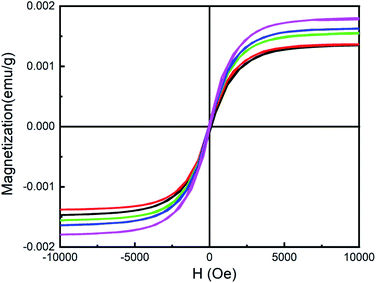 |
| Fig. 5 Hysteresis loops of CsPb1−xFexCl3 NCs at room temperature. | |
4. Conclusions
In summary, we have studied the luminescent and magnetic properties of CsPb1−xFex(Br1−yCly)3 NCs. The diffraction peak in XRD patterns shift toward a higher 2θ position indicating the successful doping of Fe2+ and Cl− into CsPbBr3 NCs. Although there is cation–anion co-exchange, PL spectra imply the band-edge energies are mainly affected by the anion exchange. The values of τav and PLQY are smaller than that of undoped CsPbBr3 NCs. In addition, with increasing the concentration of FeCl2, Ms increases monotonically, which should be attributed to the doping of Fe2+ in the lattice as a substituent for carrier-induced ferromagnetism. It is fortunate that the CsPb0.99Fe0.01(Br0.69Cl0.31)3 NCs obtained have a PLQY of 43.9% at 477 nm and show an obvious room-temperature ferromagnetism, which is of great significance to accelerate the practical application of perovskites in the magneto-optical field.
Conflicts of interest
There are no conflicts to declare.
Acknowledgements
This work was supported by the National Natural Science Foundation of China (11504132) and the Thirteenth Five-Year Program for Science and Technology of Education Department of Jilin Province (JJKH20180768KJ).
References
- J. Hieulle, S. Luo, D. Y. Son, A. Jamshaid, C. Stecker, Z. Liu, G. Na, D. Yang, R. Ohmann, L. K. One, L. Zhang and Y. Qi, J. Phys. Chem. Lett., 2020, 11, 818–823 CrossRef CAS.
- T. Q. Ma, S. W. Wang, Y. W. Zhang, K. X. Zhang and L. X. Yi, J. Mater. Sci., 2020, 55, 464–479 CrossRef CAS.
- Q. S. Chen, J. Wu, X. Y. Ou, B. L. Huang, J. Almutlaq, A. A. Zhumekenov, X. W. Guan, S. Y. Han, L. L. Liang, Z. G. Yi, J. Li, X. J. Xie, Y. Wang, Y. Li, D. Y. Fan, D. B. L. Teh, A. H. All, O. F. Mohammed, O. M. Bakr, T. Wu, M. Bettinelli, H. H. Yang, W. Huang and X. G. Liu, Nature, 2018, 561, 88–93 CrossRef CAS.
- L. Protesescu, S. Yakunin, M. I. Bodnarchuk, F. Krieg, R. Caputo, C. H. Hendon, R. X. Yang, A. Walsh and M. V. Kovalenko, Nano Lett., 2015, 15, 3692–3696 CrossRef CAS.
- A. Pramanik, K. Gates, Y. Gao, S. Begum and R. P. Chandra, J. Phys. Chem. C, 2019, 123, 5150–5156 CrossRef CAS.
- X. Yuan, X. M. Hou, J. Li, C. Q. Qu, W. J. Zhang, J. L. Zhao and H. B. Li, Phys Chem Phys, 2017, 19, 8934–8940 RSC.
- J. Li, Y. Hu, X. M. Hou, X. Yuan and L. Wang, Nanotechnology, 2020, 31, 085701 CrossRef.
- X. Li, S. Q. Chen, P. F. Liu, Y. L. Zhang, Y. Chen, H. L. Wang, H. M. Yuan and S. H. Feng, J. Am. Chem. Soc., 2020, 143, 3316–3320 CrossRef.
- J. Szeremeta, M. A. Antoniak, D. Wawrzynczyk, M. Nyk and M. Samoc, Nanomaterials, 2020, 10, 1054 CrossRef CAS.
- F. Liu, Y. H. Zhang, C. Ding, S. Kobayashi, T. Izuishi, N. Nakazawa, T. Toyoda, T. Ohta, S. Hayase, T. Minemoto, K. Yoshino, S. Y. Dai and Q. Shen, ACS Nano, 2017, 11, 10373–10383 CrossRef CAS.
- J. Pan, Y. Q. Shang, J. Yin, M. D. Bastiani, W. Peng, I. Dursun, L. Sinatra, A. M. El-Zohry, M. N. Hedhili, A. H. Emwas, O. F. Mohammed, Z. J. Ning and O. M. Bakr, J. Am. Chem. Soc., 2018, 140, 562–565 CrossRef CAS.
- C. Lou, W. Li, D. Xiong, J. Fu and W. Q. Yang, Nanoscale, 2019, 11, 15206–15215 RSC.
- Y. Zhang, H. O. Zhu, T. W. Huang, Z. P. Song and S. C. Ruan, Photonics Res, 2019, 7, 837–846 CrossRef CAS.
- C. Chen, Y. J. Wu, L. Liu, Y. B. Gao, X. F. Chen, W. B. Bi, X. Chen, D. L. Liu, Q. L. Dai and H. W. Song, Adv. Sci., 2019, 6, 1802046 CrossRef.
- J. Li, L. Gan, Z. S. Fang, H. P. He and Z. Z. Ye, J. Phys. Chem. Lett., 2017, 8, 6002–6008 CrossRef CAS.
- Y. Wu, C. Wei, X. Li, Y. Li, S. Qiu, W. Shen, B. Cai, Z. Sun, D. Yang, Z. Deng and H. Zeng, ACS. Energy. Lett., 2018, 3, 2030–2037 CrossRef CAS.
- W. Z. Lv, X. X. Tang, L. Li, L. G. Xu, M. G. Li, R. F. Chen and W. Huang, Phys. Chem. C., 2019, 123, 24313–24320 CrossRef CAS.
- H. W. Liu, Z. N. Wu, H. Gao, J. R. Shao, H. Y. Zou, D. Yao, Y. Liu, H. Zhang and B. Yang, ACS. Appl. Mater. Inter., 2017, 9, 42919–42927 CrossRef CAS.
- J. Li, Y. Hu, J. L. Zhao, X. Gao and L. Wang, J. Phys. D: Appl. Phys., 2019, 52, 505113 CrossRef CAS.
- C. Luo, W. Li, D. Xiong, J. Fu and W. Q. Yang, Nanoscale, 2019, 11, 15206–15215 RSC.
- M. A. Uddin, J. D. Glover, S. M. Park, J. T. Pham and K. R. Graham, Chem. Mater., 2020, 32, 5217–5225 CrossRef CAS.
- Z. T. Li, C. J. Song, L. S. Rao, H. G. Lu, C. M. Yan, K. Cao, X. R. Ding, B. H. Yu and Y. Tang, Nanomaterials, 2019, 9, 1296 CrossRef CAS.
- Y. A. Liu, G. C. Pan, R. Wang, H. Shao, H. Wang, W. Xu, H. N. Cui and H. W. Song, Nanoscale, 2018, 10, 14067–14072 RSC.
- K. Xing, X. Yuan, Y. Wang, J. Li, Y. J. Wang, Y. Fan, L. Yuan, K. Li, Z. J. Wu, H. B. Li and J. L. Zhao, J. Phys. Chem. Lett., 2019, 10, 4177–4184 CrossRef CAS.
- Y. D. Wang, J. L. Duan, X. Y. Yang, L. Q. Liu, L. L. Zhao and Q. W. Tang, Nano Energy, 2020, 69, 104418 CrossRef CAS.
- Z. Cao, J. Li, L. Wang, K. Xing, X. Yuan, J. L. Zhao, X. Gao and H. B. Li, Mater. Res. Bull., 2020, 121, 110608 CrossRef CAS.
- S. Shyamal, S. K. Dutta and N. Pradhan, Phys. Chem. Lett., 2019, 10, 7965–7969 CrossRef CAS.
- P. J. S. Rana, T. Swetha, H. Mandal, A. Saeki, P. R. Bangal and S. P. Singh, J. Phys. Chem. C, 2019, 123, 17026–17034 CrossRef CAS.
- Y. Hu, X. Y. Zhang, C. Q. Yang, J. Li and L. Wang, RSC Adv., 2019, 9, 33017–33022 RSC.
- A. N. Yadav, J. K. Bindra, N. Jakhar and K. Singh, CrystEngComm, 2020, 22, 1738–1745 RSC.
- A. Shapiro, M. W. Heindl, F. Horani, M. H. Dahan, J. Tang, Y. Amouyal and E. Lifshitz, J. Phys. Chem. C, 2019, 123, 24979–24987 CrossRef CAS.
- V. K. Ravi, P. K. Santra, N. Joshi, J. Chugh, S. K. Singh, H. Rensmo, P. Ghosh and A. Nag, J. Phys. Chem. Lett., 2017, 8, 4988–4994 CrossRef CAS.
- G. Pan, X. Bai, D. W. Yang, X. Chen, P. T. Jing, S. N. Qu, L. J. Zhang, D. L. Zhou, J. Y. Zhu, W. Xu, B. Dong and H. W. Song, Nano Lett., 2017, 17, 8005–8011 CrossRef CAS.
- F. Zhang, H. ZHong, C. Chen, X. G. Wu, X. Hu, H. Huang, J. Han, B. Zou and Y. Dong, ACS Nano, 2015, 9, 4533–4542 CrossRef CAS.
- A. N. Yadav, J. K. Bindra, N. Jakhar and K. Singh, CrystEngComm, 2020, 22, 1738–1745 RSC.
- J. K. Bindra, G. Kurian, J. H. Christian, J. V. Tol, K. Singh, N. S. Dalal, M. D. Mochena, S. A. Stoian and G. F. Strouse, Chem. Mater., 2018, 30, 8446–8456 CrossRef CAS.
|
This journal is © The Royal Society of Chemistry 2020 |
Click here to see how this site uses Cookies. View our privacy policy here.