DOI:
10.1039/D0RA03164J
(Paper)
RSC Adv., 2020,
10, 28059-28065
Interfacial engineering by creating Cu-based ternary heterostructures on C3N4 tubes towards enhanced photocatalytic oxidative coupling of benzylamines†
Received
8th April 2020
, Accepted 18th July 2020
First published on 28th July 2020
Abstract
Benzylamine coupling is a very important reaction for the synthesis of imine but still faces many challenges. Herein, we present a highly effective strategy towards the coupling reaction by using environmentally friendly catalysts. These catalysts are composed of Cu/Cu2O/Cu3N heterostructures supported by C3N4 tubes and the composites were synthesized by one-step hydrothermal treatment followed by calcination. Cu2O, Cu3N, and C3N4 all are responsive to visible light and the heterojunction formed can greatly enhance the charge separation. When used as photocatalysts for oxidative self-coupling of benzylamine at a low temperature of 323 K in air, Cu/Cu2O/Cu3N/C3N4 was able to give conversion and selectivity values of up to 99% and 98%, respectively. The high efficiency of the catalysts is attributable to their ability to generate large quantities of free radicals (such as ·OH and ·O2−) under visible-light irradiation.
1. Introduction
Imines are important intermediates in the synthesis of many organic compounds.1,2 The traditional synthetic method for imines is the condensation of amines with carbonyl compounds, which generally needs costly dehydrating agents, and Lewis acids as the catalysts.3,4 However, this method is not only cumbersome to operate, but also causes some side reactions, and even causes serious pollution to the environment. Alternatively, the direct synthesis of imines through an oxidative process such as self-coupling of benzylamine has attracted much attention, owing to reduced energy consumption, and simplified procedures.5,6 Furthermore, the utilization of solar light as the energy source for directly oxidizing benzylamine is a green and promising strategy.
For the oxidative coupling of benzylamine, CuCl was firstly used as the catalyst in the presence of air, and good activity was achieved.7 Subsequently, many other catalysts for this reaction have been explored. However, these catalytic reactions involve complex systems (oxidants and alkali auxiliaries), long reaction time, and are carried out at high temperatures and pressures.8 Juntrapirom et al.9 used O2 as an oxidant to convert benzylamine with a conversion rate and selectivity of up to 94% and 85%, respectively, at room temperature and under visible light irradiation for 4 hours. This may be due to the fact that benzylamine reacts with pure oxygen to form a series of by-products. At a low reaction temperature (35 °C), Chai et al.11 did not greatly improve the rate of benzylamine conversion carried out in air for 5 hours, although the selectivity was high (>99%). In the absence of O2 and at a low reaction temperature (35 °C), Zhang et al.10 achieved a conversion rate of 80% after conducting the reaction for a long time (200 hours). Therefore, it is still a challenge to increase the conversion of benzylamine under mild reaction conditions. It is well known that the pyrolysis of benzylamine releases toxic gases. Currently the most commonly used catalysts are expensive noble metals such as Ru,12 Pd,13 and Au.14 Thus, researchers have turned their attention to cheaper catalysts containing non-noble metals such as g-C3N4,15 Cu2O,16 Fe2O3,17 and TiO2,18 which also show excellent photocatalytic performance.
Among them, g-C3N4 is an attractive organic semiconductor photocatalyst owing to its outstanding visible light activity, thermal and chemical stability. Interestingly, it can also be applied for the oxidative coupling of benzylamine under visible light.19–21 Therefore, in this work, we present a Cu/Cu2O/Cu3N ternary composite formed on C3N4 tubes for catalyzing the oxidative coupling reaction of benzylamine under visible-light light using air as the oxidant. The conversion and selectivity of the reaction were as large as 99% and 98%, respectively. In particular, Cu3N, which has shown prominent activity in the electrocatalytic hydrogen evolution and oxygen evolution reactions,22,23 was explored for the first time as a co-photocatalyst for the coupling reaction.
2. Experimental
2.1 Materials
Copper acetate hydrate (Cu(Ac)2·H2O; analytically pure reagent, 99.98%), sodium hydroxide (NaOH; AR, 96%), melamine (AR, 99%) and dimethyl sulfoxide (DMSO; chromatographically pure, ≥99.8%) were purchased from Aladdin Reagent. Benzylamine (chromatographically pure, ≥99.0%) was bought from Sigma Aldrich. Deionized water was used during the experiments. The Cu3N and Cu2O used for testing and characterization in the experiment are purchased commercial products.
2.2 Fabrication of Cu2O on a supramolecular precursor of C3N4
First, 5 mL of 0.8 M NaOH solution was slowly added into 30 mL of 0.1 M Cu(Ac)2 solution under stirring for 20 min at 25 °C. Next, various amounts of melamine [nCu(Ac)2
:
nmelamine = 1
:
1, 1
:
2, 1
:
3 and 1
:
4 (molar ratio)] were each added into the above solution, and the resultant suspensions were stirred in a water bath at 60 °C for 30 min. Subsequently, the mixture solutions were transferred into 50 mL polytetrafluoroethylene autoclaves and then kept at 180 °C for 10 h. Finally, black-brown precipitates were obtained by centrifugation, washed three times with hot water, and then dried at 60 °C for 6 h.
2.3 Fabrication of Cu/Cu2O/Cu3N on hexagonal C3N4 tubes
The black-brown precipitates were each transferred to a porcelain boat and then were calcined at 400 °C for 2 h in an argon atmosphere that prevents the oxidation of Cu2O (the flow rate was set at 5 mL min−1). As a result, dark-green samples were obtained. These samples were named as Cu/Cu2O/Cu3N/C3N4-1, Cu/Cu2O/Cu3N/C3N4-2, Cu/Cu2O/Cu3N/C3N4-3, and Cu/Cu2O/Cu3N/C3N4-4, corresponding to the molar ratio of Cu(Ac)2 to melamine of 1
:
1, 1
:
2, 1
:
3 and 1
:
4, respectively.
2.4 Characterization
Morphologies of the samples were characterized through scanning electron microscopy (SEM) with Hitachi S-4800 operating at 15 KV and transmission electron microscopy (TEM) with JEMF200 an operating at an acceleration voltage of 200 kV. The X-ray diffraction (XRD) was conducted at room temperature on a Bruker D8 ADVANCE diffractometer with nickel-filtered Cu Ka radiation (λ = 1.5406 Å). X-ray photoelectron spectroscopy (XPS) was performed on VG ESCALAB MK II with an achromatic Mg Ka radiation (1253.6 eV) to analyze the electronic states of the surface. N2 adsorption–desorption isotherms were recorded on Tristar II 3020 and surface areas were determined by using the Brunauer–Emmett–Teller (BET) method. UV-visible diffuse reflectance spectra (UV-vis DRS) were obtained on a Perkin-Elmer-Lambda 950 spectrophotometer using BaSO4 as the referencing material. The photoluminescence (PL) spectra of the samples were measured with a spectrofluoro-photometer (Hitachi FLS1000) at an excitation wavelength of 375 nm. The Scanning Kelvin Probe (SKP) measurements (SKP5050 system, Scotland) were implemented under normal conditions (i.e., room temperature and ambient pressure). Electrochemical impedance spectroscopy (EIS) measurements were performed under simulated sunlight irradiation at open circuit voltage over a frequency range from 105 to 0.05 Hz with an AC voltage of 5 mV. Mott–Schottky analysis was performed by using an electrochemical Princeton instrument at different frequencies versus the saturated Ag/AgCl electrode (pH = 7). Benzylamine and imine products were detected using GC-MS (Thermo Trace1300).
2.5 Photocatalytic oxidative coupling of benzylamine
To begin with, 40 mg of a prepared product was placed in a Schlenk flask. Subsequently, 3 mL of chromatographically pure dimethyl sulfoxide (DMSO) was added as the solvent, followed by 3 mmol of chromatographically pure benzylamine. Next, the flask was placed in a sonicator for 5 min until a homogeneous suspension was obtained. The suspension was then heated to 323 K using a heating mantle and irradiated with a 300 W Xe lamp for 12 h. After the reaction, 1 mL of the suspension was taken and passed through a 0.22 μm organic filter to remove the catalyst. The resulting filtrate was extracted with ethyl acetate and then analyzed by GC-MS.
3. Results and discussion
3.1 Crystal structure and morphology
As is evident from the SEM image (Fig. 1a), the assembly of melamine in the presence of NaOH resulted in a supramolecular precursor of C3N4 with a hexagonal rod-like structure, and the rods are evenly decorated with a large number of CuO particles generated from the reaction between copper acetate and the base. Interestingly, after calcination these solid rods became smaller and hollow, producing a tubular structure (Fig. 1b and c). Moreover, the particles on the surface of each tube aggregated. In the high-resolution TEM (HRTEM) image of the calcined product, two lattice fringes can be clearly seen (Fig. 1d), which correspond to the (111) crystal plane of Cu2O (d111 = 0.24 nm) and the (111) crystal plane of Cu3N (d111 = 0.22 nm), respectively. In other words, copper(II) oxide was reduced to highly crystalline copper(I)-containing species during the thermal treatment. The presence of C, N, Cu, and O was verified by elemental mapping and these elements are uniformly distributed across the tubular structure (Fig. 1e–h).
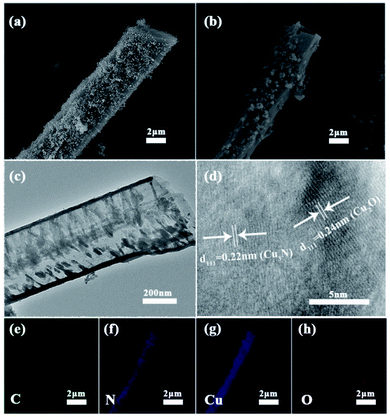 |
| Fig. 1 High-magnification SEM images of (a) the precursor and (b) Cu/Cu2O/Cu3N/C3N4-3; (c) TEM and (d) HRTEM images of Cu/Cu2O/Cu3N/C3N4-3; (e–h) elemental mappings of C, N, Cu, O for Cu/Cu2O/Cu3N/C3N4-3 in the region shown in (b). | |
Phases of the product were further investigated by XRD experiments. It turned out that samples synthesized from different Cu (Ac)2/melamine molar ratios have similar patterns (Fig. 2a). The peaks at 43.2°, 50.4° and 74.1° correspond to the (111), (200) and (220) crystal planes of Cu (PDF# 04-0836); those at 36.4°, 42.2° and 61.3° can be indexed to the (111), (200) and (220) crystal planes of Cu2O (PDF# 05-0667); lastly, the peaks at 23.2°, 40.8° and 47.5° correspond to the (100), (111) and (200) crystal planes of Cu3N (PDF# 47-1088).24–29 Further, a weak broad peak at about 27° is visible, which is attributable to the X-ray reflections from the (002) crystal planes of C3N4 (corresponding to interlayer stacking).30,31 Moreover, all these characteristic peaks are sharp and strong due to the good crystallinity of Cu, Cu2O, and Cu3N, which is in line with the TEM result. However, the crystallinity of carbon nitride is poor. Although the diffraction of X-rays by C3N4 is not well resolved due to the presence of Cu2O with higher crystallinity (Fig. 2a and S1†), in the XRD pattern of pure C3N4 synthesized without copper acetate pure, the characteristic peaks at 13° and 27° can be clearly seen (Fig. S2†), verifying that C3N4 indeed can be synthesized by the thermal polymerization of melamine. As the Cu(Ac)2/melamine molar ratio was increased, the content of C3N4 increases, the crystallinity of the composite will also decrease, as a result, Cu XRD peaks became weaker, indicating a decrease in the Cu content. This result was further verified by inductively coupled plasma (ICP) measurements, which show that the mass percentage was reduced from 66.46% (Cu/Cu2O/Cu3N/C3N4-1) to 33.47% (Cu/Cu2O/Cu3N/C3N4-4, Table S1†). In the FT-IR spectrum (Fig. S3†), the peak at 808 cm−1 is attributable to the vibration of the triazine ring, further supporting the presence of CN in Cu/Cu2O/Cu3N/C3N4. As the amount of melamine increases, the vibration intensity of C3N4 also strengthens. The peaks appearing in the range of 1200–1600 cm−1 are due to the tensile vibration of C–N and C
N. The broad peak in the range of 3100–3300 cm−1 is formed by the stretching vibration of N–H and O–H owing to adsorbed water molecules.
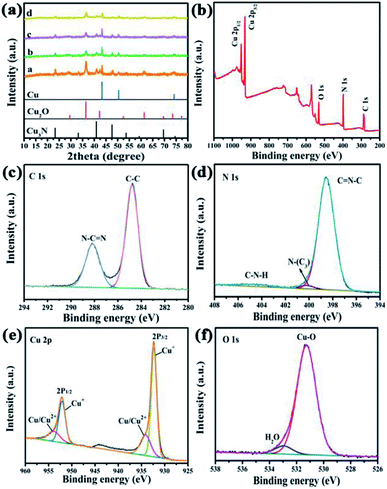 |
| Fig. 2 (a) XRD patterns. (a) Cu/Cu2O/Cu3N/C3N4-1; (b) Cu/Cu2O/Cu3N/C3N4-2; (c) Cu/Cu2O/Cu3N/C3N4-3; (d) Cu/Cu2O/Cu3N/C3N4-4. (b) XPS survey spectrum of Cu/Cu2O/Cu3N/C3N4-3; high-resolution XPS (c) C 1s, (d) N 1s, (e) Cu 2p, and (f) O 1s spectra of Cu/Cu2O/Cu3N/C3N4-3. | |
The chemical state of the synthesized materials and the elemental composition on the surfaces were studied by X-ray photoelectron spectroscopy (XPS). The characteristic peaks of C, N, Cu, and O are clearly visible in the survey spectrum (Fig. 2b), suggesting that the surface regions of the materials contain the same elements as the bulk parts. The high-resolution XPS C 1s spectrum exhibits two peaks at 284.6 eV and 288.1 eV (Fig. 2c), which can be assigned to carbon in C–C and N–C
N (namely sp2-hybridized C), respectively.32,33 The XPS N 1s spectrum can be deconvoluted into two core lines, featuring three peaks (Fig. 2d). The peak at 398.5 eV corresponds to N of the triazine ring (C
N–C) or Cu–N, and the peak at 400.2 eV is due to surface-adsorbed NH3 (which was produced during the thermal polymerization of melamine) and tertiary nitrogen in the N–(C)3 or HN(C)2 groups. The peak at 404.5 eV belongs to C–N–H.34,35 Therefore, the XPS results verify the successful preparation of C3N4.
In the XPS Cu 2p spectrum (Fig. 2e), a pair of peaks at 932.7 eV and 953.7 eV can be ascribed to 2p3/2 and 2p1/2, respectively, of Cu+.36 Another pair of peaks at 934.2 eV and 954.6 eV are attributed to metallic Cu0 or Cu2+.37 According to XRD characterization, there are characteristic peaks of Cu and no characteristic peaks of Cu2+ are found. Therefore, these two peaks are Cu, while the peak of Cu+ belongs to Cu2O in the catalyst. Hence, XPS further confirms the XRD result that during the thermal treatment of the supramolecular precursor CuO can be reduced to Cu and Cu2O, possibly due to the reducing gases such as NH3 released. The presence of two types of oxygen, namely lattice oxygen and oxygen in the water adsorbed on the surface, is evidenced by the peaks at 531.3 eV, and 532.9 eV, respectively, in the XPS O 1s spectrum (Fig. 2f).38 Quantitative analysis by using elemental analysis revealed that the C/N ratio for all the composites (Table S2†) is smaller than the theoretical value for C3N4 (i.e., 0.6428).39 Particularly, Cu/Cu2O/Cu3N/C3N4-3 has the smallest C/N ratio (0.5767). Carbon nitride with a larger percentage of N is believed to have more active sites and therefore give better photocatalytic performance. Hence, Cu/Cu2O/Cu3N/C3N4-3 was selected for the remaining experiments.
3.2 Optical properties and charge dynamics
Fig. 3a and S4a† show the diffuse reflectance spectra (DRS) of Cu2O, Cu3N, C3N4 and Cu/Cu2O/Cu3N/C3N4-3 in the 300–900 nm range. The absorption spectrum of Cu/Cu2O/Cu3N/C3N4-3 is similar to that of Cu3N but is slightly shifted toward larger absorbance. Cu3N, Cu2O and Cu/Cu2O/Cu3N/C3N4-3 show stronger optical absorption than C3N4 at wavelengths above the absorption edge of C3N4, namely 579.9 nm. The optical band gaps of Cu2O, Cu3N, C3N4 and Cu/Cu2O/Cu3N/C3N4-3 were determined to be 1.89 eV, 1.12 eV, 2.19 eV and 0.82 eV, respectively, from the Tauc plots displayed in Fig. 3b and S4b.† Photocatalysts with small band gaps are more favorable owing to their enhanced light absorption ability.
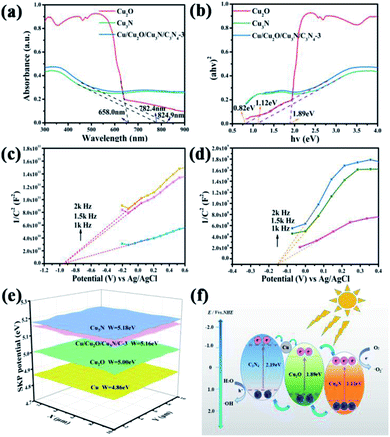 |
| Fig. 3 (a) Diffuse reflectance spectra (DRS) and (b) Tauc plots of Cu2O, Cu3N and Cu/Cu2O/Cu3N/C3N4-3. (c) Mott–Schottky plots of Cu2O and (d) Cu3N at various frequencies (pH = 7). (e) Scanning Kelvin probe mappings (SKP) of Cu2O, Cu3N and Cu/Cu2O/Cu3N/C3N4-3. (f) Energy band diagram. | |
Mott–Schottky plots of Cu3N and Cu2O at three frequencies (i.e., 1.0 kHz, 1.5 kHz, and 2.0 kHz) were recorded to determine the flat-band potentials. The flat-band potentials of Cu3N, Cu2O and C3N4 were assessed to be about 0.04, −0.76 and −1.12 (vs. NHE), respectively, by extrapolation. In turn, the valence band edges of Cu3N, Cu2O and C3N4 were calculated by using the band gaps. Based on the potential difference, electrons in Cu3N transfer to Cu2O. To further confirm this electron transfer direction, scanning Kelvin probe (SKP) was utilized to determine the work functions, which were calculated according to the following formula: W = 5.1 + Wave/1000, where W and Wave refer to the work function and work function average, respectively. It turned out that the work function decreases in the order of Cu3N > Cu/Cu2O/Cu3N/C3N4-3 > Cu2O > Cu (Fig. 3e). In other words, the Fermi level relative to the vacuum level increases in the order Cu3N < Cu/Cu2O/Cu3N/C3N4-3 < Cu2O < Cu; therefore, in the Cu/Cu2O/Cu3N/C3N4-3 heterojunction electrons transfer from Cu to Cu2O and finally to Cu3N. The band diagram of Cu/Cu2O/Cu3N/C3N4-3 is shown in Fig. 3f and S5.† Under the irradiation of visible light, Cu2O, Cu3N, and C3N4 are excited and electron–hole pairs are generated. According to the potential difference, the electrons on C3N4 are transferred to Cu, then to Cu2O and finally to Cu3N. In the meanwhile, holes are transferred from Cu3N to Cu2O and finally transported to C3N4. Subsequently, electrons react with O2 and holes react with water to produce highly reactive ·O2− and ·OH, respectively, which may induce the coupling reaction of benzylamine. The specific surface area (SSA) and pore size of Cu/Cu2O/Cu3N/C3N4-3 were determined to be 45.6 m2 g−1 and 24.0 nm, respectively, from nitrogen adsorption–desorption isotherms (Fig. S5†). SSA of Cu/Cu2O/Cu3N/C3N4-3 is larger than those of Cu, Cu2O, and Cu3N, indicating that the former has the most reaction sites on its surface, which is beneficial to the oxidation of benzylamine.
The performance of a photocatalyst greatly depends on its charge dynamics, which can be investigated by photoluminescence (PL) spectroscopy and electrochemical impedance spectroscopy (EIS).40 The measurement of PL intensity for different samples at an excitation wavelength of 375 nm is shown in Fig. 4a. As can be seen, the PL intensity of Cu/Cu2O/Cu3N/C3N4-3 is much lower than that of other samples, illustrating that the recombination of the electron–hole pairs in Cu/Cu2O/Cu3N/C3N4-3 is suppressed as compared with other samples. The conclusion is further confirmed by EIS (Fig. 4b). The Nyquist plot of Cu/Cu2O/Cu3N/C3N4-3 displays the smallest semicircle among all samples studied, which indicates that it has the smallest interfacial charge transfer resistance.
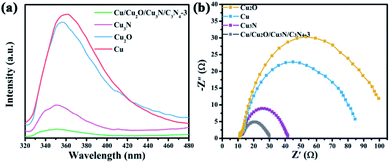 |
| Fig. 4 (a) Photoluminescence spectra of Cu, Cu2O, Cu3N and Cu/Cu2O/Cu3N/C3N4-3. (b) Nyquist plots of Cu, Cu2O, Cu3N and Cu/Cu2O/Cu3N/C3N4-3. | |
3.3 Photocatalytic tests
Photocatalysis is typically conducted at mild and environmentally friendly conditions, and it involves the generation of electrons and holes that trigger a series of redox reactions.41 The performance of the synthesized materials toward photocatalytic coupling reaction of benzylamine in the presence of air and at 323 K is shown in Table 1. It can be seen that both Cu2O and Cu3N are able to induce the oxidative coupling reaction of benzylamine and the conversion was 12% and 80%, respectively. However, Cu/Cu2O/Cu3N/C3N4 photocatalysts exhibited higher activities. Although Cu3N plays a major role in the reaction, Cu2O and Cu can catalyze the oxidation of benzylamine and thereby promote the reaction. Additionally, it was observed that for Cu/Cu2O/Cu3N/C3N4-3 the selectivity and conversion of benzylamine oxidation were 98% and 99%, respectively. In order to further study the effect of light on the catalytic reaction, a benzylamine oxidative coupling reaction was performed under a single reaction condition only, and the results are included in Table S3.† When Cu/Cu2O/Cu3N/C3N4-3 was heated to 50 °C (323 K) without light, the conversion and selectivity were very low, reaching only 33% and 94%, respectively. After light was introduced, the conversion and selectivity were increased to 74% and 98%, respectively. The results of oxidative coupling of benzylamine in different atmospheres are listed in Table S4.† It was found that under inert gas, the conversion rate of benzylamine is very low, only 44%, but the selectivity reaches 98%. In an atmosphere containing oxygen, the conversion of benzylamine can be close to 100%, but the selectivity of benzylamine under pure oxygen is lower than that under air. This may be due to the reaction of benzylamine with oxygen that produces some by-products such as benzylalcohol and benzaldehyde. All the above data are derived from Gas Chromatography-Mass Spectrometer (GC-MS). This paper uses external standard method to fit the line graph of benzylamine concentration and peak area as shown in the Fig. S6.† The benzylamine peak area acquired by the GC-MS test was calculated by a one-time function formula (Fig. S6†) to obtain the benzylamine concentration, and the benzylamine conversion rate was calculated. With reasonable test conditions, the retention time of chromatographic grade benzylamine is 1.69 min, as shown in the Fig. S7.†
Table 1 Coupling reaction of benzylamine with different catalystsa

|
Sample |
Conversion |
Selectivity |
Reaction condition: 3 mmol benzylamine, 12 h, 40 mg Cu/Cu2O/Cu3N/C3N4-3, cut 420 nm with 300 mW cm−2, Xe lamp, 3 mL dimethyl sulfoxide (DMSO), 323 K and air atmosphere. |
Cu2O |
12% |
99% |
Cu |
77% |
89% |
Cu3N |
80% |
85% |
Cu/Cu2O/Cu3N/C3N4-1 |
93% |
96% |
Cu/Cu2O/Cu3N/C3N4-2 |
97% |
92% |
Cu/Cu2O/Cu3N/C3N4-3 |
99% |
98% |
Cu/Cu2O/Cu3N/C3N4-4 |
60% |
98% |
— |
7% |
62% |
The photocatalytic activity of Cu/Cu2O/Cu3N/C3N4-3 was further studied by using many benzylamine derivatives with electron withdrawing or donating groups as the substrates. The electron-withdrawing groups include –F, –Cl, –Br, –OCH3 and –CF3, while the electron-donating groups include –CH3 and –(CH3)3C. The conversion rates involving the electron-donating groups are 98% for 4-methylbenzylamine and 78% for 4-tert-butylbenzylamine, while those involving the electron-withdrawing groups are 89% (–F), 85% (–Cl), 82% (–Br), 72% (–CF3) and 88% (–OCH3). Generally, electron-donating groups have stronger oxidizing ability than electron-withdrawing groups;42 however, it is not clearly reflected in the present work. Interestingly, the conversion rate of 4-tert-butylbenzylamine coupling was 20% lower than that of 4-methylbenzylamine coupling, indicating that the tert-butyl group is not conducive to the photocatalytic benzylamine coupling reaction, which may be ascribed to the steric effect.43 Furthermore, both the conversion and selectivity for the coupling reaction of any benzylamine with a methyl group were larger than 90% regardless of the position of the group. From the table, it finds that the conversion and selectivity of benzylamine with different groups are at the upper levels, indicating that the catalyst has a promoting effect on the organic reaction (Table 2).
Table 2 Selective oxidation of different aromatic amines with Cu/Cu2O/Cu3N/C3N4-3 as the catalysta
Substrate |
Produce |
Con. |
Sel. |
Reaction condition: 3 mmol different aromatic amines, 12 h, 40 mg Cu/Cu2O/Cu3N/C3N4-3, cut 420 nm with 300 mW cm−2, Xe lamp, 3 mL dimethyl sulfoxide (DMSO) and air atmosphere. |
 |
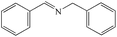 |
99% |
98% |
 |
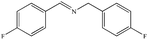 |
89% |
98% |
 |
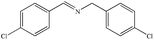 |
85% |
96% |
 |
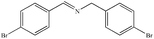 |
82% |
96% |
 |
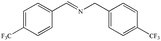 |
72% |
97% |
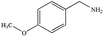 |
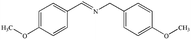 |
88% |
98% |
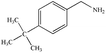 |
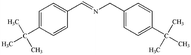 |
78% |
94% |
 |
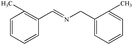 |
96% |
92% |
 |
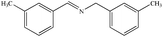 |
98% |
95% |
 |
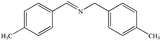 |
98% |
96% |
 |
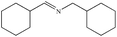 |
92% |
95% |
3.4 Mechanism of photocatalytic benzylamine coupling
The underlying mechanism of the coupling reaction of benzylamines was studied by electron spin resonance (ESR). To detect ·OH and ·O2−, 5, 5-dimethyl-1-pyrroline-N-oxide (DMPO) that captures these radicals was added. Based on the ESR spectra shown in Fig. 5a and b, the amount of these two radicals decreases in the sequence of Cu/Cu2O/Cu3N/C3N4-3 > Cu3N > Cu > Cu2O, which is consistent with their oxidation performance discussed above. ·O2− has a stronger oxidizing ability than ·OH, and therefore plays a major role in the photocatalytic coupling of benzylamine. Samples for GC-MS were taken at 3rd, 6th, 9th and 12th hour during the oxidative coupling reaction of benzylamine. The mass spectrum of the 3rd-hour sample shows peaks at m/z = 103, 105, 106.12, 107.15 and 108.13, corresponding to benzonitrile, intermediate I, benzaldehyde, benzylamine and benzylalcohol, respectively (Fig. S8a†). At the same time, a peak at m/z = 194.15, which belongs to imine, can be observed in the mass spectrum of the 3rd-hour sample (Fig. S8b†). In the chromatogram (Fig. S8c–f†), peaks with retention times of 1.69 and 1.71 are the characteristic peaks of benzylamine, while peaks with retention times of 5.41 and 5.42 belong to imines. As benzylamine was consumed while the imine was produced, the relative intensity of the imine peak to the benzylamine peak was increased. And it is also accompanied by the conversion of some by-products to imine.
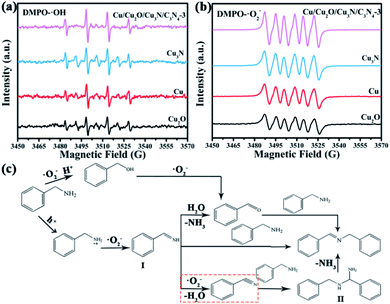 |
| Fig. 5 Electron spin resonance (ESR) spectra of radicals trapped by 5,5-dimethyl-1-pyrroline-N-oxide (DMPO) in (a) aqueous dispersion for DMPO-·OH and (b) methanol dispersion for DMPO-·O2−. (c) Proposed processes toward the formation of an imine from benzylamine over Cu/Cu2O/Cu3N/C3N4-3. | |
Therefore, two possible oxidation pathways are proposed in Fig. 5c. The first pathway is a traditional synthesis method, namely oxidative condensation of benzylalcohol and benzylamine to obtain imine. In the first pathway, benzylamine is oxidized by ·O2− to benzylalcohol with m/z = 108.13 and then to benzaldehyde with m/z = 106.12, which is subsequently dehydrated and condensed with a benzylamine molecule through nucleophilic substitution, producing an imine with m/z = 194.15. Specifically, the O atom bonded with α-C is replaced by the N atom of –NH2 in a benzylamine molecule.44
In the second pathway, a benzylamine molecule becomes a positively charged benzylamine radical with the help of a hole and then the benzylamine radical is oxidized by ·O2− to form an imine intermediate I with m/z = 105,45 which is converted to imine through three ways. The first way is the hydrolysis of intermediate I that releases NH3 molecules and creates benzaldehyde with m/z = 106.12.46 Same as the last step of the first pathway, the reaction between benzaldehyde and benzylamine results in the imine with the release of H2O.47 Also, a simpler and more dominant way is that intermediate I directly reacts with benzylamine and imine is produced as one of the two products (NH3 being the other product).48 The last way starts with the dehydration of intermediate I with the help of ·O2−, which yields benzonitrile (m/z = 103).49 Next, benzonitrile reacts with benzylamine to form a diamine (intermediate II), which then undergoes deamination to give an imine. This third path mainly occurs as a side reaction.49
4. Conclusions
In summary, ternary Cu/Cu2O/Cu3N heterostructures have been successfully prepared on C3N4 tubes by hydrothermal synthesis and heat treatment. Cu and Cu3N in the composites protect Cu2O from photo-corrosion and improve the photocatalytic activity. Particularly, Cu/Cu2O/Cu3N/C3N4-3 is responsive to a wide range of light from visible to near-infrared, and is able to produce highly reactive ·O2−. Therefore, under visible light irradiation, Cu/Cu2O/Cu3N/C3N4-3 can induce the synthesis of imine at 323 K by using benzylamine. The conversion and selectivity of benzylamine coupling reaction are up to 99% and 98%, respectively. This work not only demonstrates the potential of Cu3N and other copper-based semiconductors for organic synthesis, but also presents a promising strategy involving photocatalysis towards imine production through the coupling reaction of benzylamine.
Conflicts of interest
There are no conflicts to declare.
Acknowledgements
This work was supported by the National Natural Science Foundation of China (21771061).
Notes and references
- H. X. Wei, Z. F. Guo, X. Liang, P. Q. Chen, H. Liu and H. Z. Xing, ACS Appl. Mater. Interfaces, 2019, 11, 3016–3023 CrossRef CAS PubMed.
- J. I. Yang and C. Y. Mou, Appl. Catal., B, 2018, 231, 283–291 CrossRef CAS.
- H. Naeimi, F. Salimi and K. Rabiei, J. Mol. Catal. A: Chem., 2006, 260, 100–104 CrossRef CAS.
- J. N. Young, T. C. Chang, S. C. Tsai, L. Yang and S. C. Yu, J. Catal., 2010, 272, 253 CrossRef CAS.
- S. Furukawa, Y. Ohno, T. Shishido, K. Teramura and T. Tanaka, ACS Catal., 2011, 1, 1150 CrossRef CAS.
- M. H. So, Y. G. Liu, C. M. Ho and C. M. Che, Chem.–Asian J., 2009, 4, 1551 CrossRef CAS PubMed.
- R. D. Patil and S. Adimurthya, Adv. Synth. Catal., 2011, 353, 1695–1700 CrossRef CAS.
- J. M. Bermudez, J. A. Menendez, A. Arenillas, R. M. Palou, A. A. Romero and R. Luque, J. Mol. Catal. A: Chem., 2015, 406, 19–22 CrossRef CAS.
- S. Juntrapirom, S. Anuchai, O. Thongsook, S. Pornsuwan, P. Meepowpan, P. Thavornyutikarn, S. Phanichphant, D. Tantraviwat and B. Inceesungvorn, Chem. Eng. J., 2020, 394, 124934 CrossRef CAS.
- Y. W. Zhang, J. S. Xu, J. Mei, S. Sarina, Z. Y. Wu, T. Liao, C. Yan and Z. Q. Sun, J. Hazard. Mater., 2020, 394, 122529 CrossRef CAS PubMed.
- Y. Y. Chai, L. Zhang, Q. Q. Liu, F. l. Yang and W. L. Dai, ACS Sustainable Chem. Eng., 2018, 6, 10526–10535 CrossRef CAS.
- K. Yamaguchi and N. Mizuno, Angew. Chem., Int. Ed., 2003, 42, 1480–1483 CrossRef CAS PubMed.
- R. S. Wang, G. H. Qiu, Y. Xiao, X. Q. Tao, W. Peng and B. X. Li, J. Catal., 2019, 374, 378–390 CrossRef CAS.
- Z. X. Qin, D. Zhao, L. Zhao, Q. Xiao, T. T. Wu, J. W. Zhang, C. Q. Wan and G. Li, Nanoscale Adv., 2019, 1, 2529 RSC.
- K. X. Zhang, H. Su, H. H. Wang, J. J. Zhang, S. Y. Zhao, W. W. Lei, X. Wei, X. H. Li and J. S. Chen, Adv. Sci., 2018, 5, 1800062 CrossRef PubMed.
- M. Gopiraman and I. M. Chung, J. Taiwan Inst. Chem. Eng., 2017, 81, 455–464 CrossRef CAS.
- R. Mazzaro, S. B. Bibi, M. Natali, G. Bergamini, V. Morandi, P. Ceroni and A. Vomiero, Nano Energy, 2019, 61, 36–46 CrossRef CAS.
- T. Yang, Q. M. Yu and H. M. Wang, Catal. Lett., 2018, 148, 2382–2390 CrossRef CAS.
- X. C. Wang, K. Maeda, A. Thomas, K. Takanabe, G. Xin, J. M. Carlsson, K. Domen and M. Antonietti, Nat. Mater., 2009, 8, 76–80 CrossRef CAS PubMed.
- J. Liu, Y. Liu, N. Y. Liu, Y. Z. Han, X. Zhang, H. Huang, Y. Lifshitz, S. T. Lee, J. Zhong and Z. H. Kang, Science, 2015, 347, 970–974 CrossRef CAS PubMed.
- S. E. Guo, Z. P. Deng, M. X. Li, B. J. Jiang, C. G. Tian, Q. J. Pan and H. G. Fu, Energy Environ. Sci., 2011, 4, 2922–2929 RSC.
- C. Panda, P. W. Menezes, M. Zheng, S. Orthmann and M. Driess, ACS Energy Lett., 2019, 4, 747–754 CrossRef CAS.
- H. L. Liu, Q. Wu, L. M. Li and X. S. Tai, Dalton Trans., 2019, 16, 5131–5134 Search PubMed.
- Y. M. Juan, S. J. Chang, H. T. Hsueh, S. H. Wang, T. C. Cheng, S. W. Huang and C. L. Hsu, RSC Adv., 2015, 67, 54220–54224 RSC.
- F. L. Meng, H. X. Zhong, Q. Zhang, K. H. Liu, J. M. Yan and Q. Jiang, J. Mater. Chem. A, 2017, 5, 18972 RSC.
- Z. P. Zeng, Y. B. Yan, J. Chen, P. Zan, Q. H. Tian and P. Chen, Adv. Funct. Mater., 2018, 29, 1806500 CrossRef.
- S. R. Ye, A. R. Rathmell, A. R. Wilson and B. J. Wiley, Small, 2014, 10, 1771–1778 CrossRef CAS PubMed.
- C. Y. Su, B. H. Liu, T. J. Lin, Y. M. Chi, C. C. Kei, K. W. Wang and T. P. Perng, J. Mater. Chem. A, 2015, 3, 18983 RSC.
- F. L. Meng, H. X. Zhong, Q. Zhang, K. H. Liu, J. M. Yan and Q. Jiang, J. Mater. Chem. A, 2017, 5, 18972 RSC.
- J. S. Zhang, X. F. Chen, K. Takanabe, K. Maeda, K. Domen, J. D. Epping, X. Z. Fu, M. Antonietti and X. C. Wang, Angew. Chem., Int. Ed., 2010, 49, 441–444 CrossRef CAS PubMed.
- S. E. Guo, Y. Q. Tang, Y. Xie, C. G. Tian, Q. M. Feng, W. Zhou and B. J. Jiang, Appl. Catal., B, 2017, 218, 664–671 CrossRef CAS.
- J. Zhang, M. Zhang, G. Zhang and X. Wang, ACS Catal., 2012, 2, 940–944 CrossRef CAS.
- F. Dong, Z. W. Zhao, T. Xiong, Z. L. Ni, W. D. Zhang, Y. J. Sun and W. K. Ho, Appl. Mater. Interfaces, 2013, 5, 11392–11401 CrossRef CAS PubMed.
- R. Deshmukh, G. B. Zeng, E. Tervoort, M. Staniuk, D. Wood and M. Niederberger, Chem. Mater., 2015, 27, 8282–8288 CrossRef CAS.
- F. Hou, Y. Li, Y. T. Gao, S. Hu, B. G. Wu, H. L. Bao, H. Wang and J. B. Jiang, Mater. Res. Bull., 2019, 110, 18–23 CrossRef CAS.
- J. Zhang, J. G. Yu, Y. M. Zhang, Q. Li and J. R. Gong, Nano Lett., 2011, 11, 4774–4779 CrossRef CAS PubMed.
- S. Zhang, X. Wen, M. Long, J. Xi, J. Hu and A. Tang, J. Alloys Compd., 2020, 829, 154568 CrossRef CAS.
- A. Mitra, P. Howli, D. Sen, B. Das and K. K. Chattopadhyay, Nanoscale, 2016, 8, 19099–19109 RSC.
- Y. Q. Tang, M. Yuan, B. J. Jiang, Y. T. Xiao, Y. Fu, S. Chen, Z. P. Deng, Q. J. Pan, C. G. Tian and H. G. Fu, J. Mater. Chem. A, 2017, 5, 21979–21985 RSC.
- P. Z. Qiao, B. J. Sun, H. Z. Li, Y. C. Xu, L. P. Ren, K. Pan, L. Wang and W. Zhou, ACS Appl. Mater. Interfaces, 2019, 11, 7066–7073 CrossRef CAS PubMed.
- M. Mousavi, A. H. Yangjeh and S. R. Pouran, J. Mater. Sci.: Mater. Electron., 2018, 29, 1719–1747 CrossRef CAS.
- S. Furuawa, Y. Ohno, T. Shishido, K. Teramura and T. Tanaka, ACS Catal., 2011, 1, 1150–1153 CrossRef.
- A. L. Yuan, H. Lei, Z. S. Wang and X. P. Dong, J. Colloid Interface Sci., 2020, 560, 40–49 CrossRef CAS PubMed.
- P. Bai, X. l. Tong, J. Wan, Y. Q. Gao and S. Xue, J. Catal., 2019, 374, 257–265 CrossRef CAS.
- G. H. Qiu, R. S. Wang, F. Han, X. Q. Tao, Y. Xiao and B. X. Li, Ind. Eng. Chem. Res., 2019, 58, 17389–17398 CrossRef CAS.
- Y. Xiao, X. Q. Tao, G. H. Qiu, Z. F. Dai, P. Gao and B. X. Li, J. Colloid Interface Sci., 2019, 550, 99–109 CrossRef CAS PubMed.
- M. Largeron and M. B. Fleury, Chem.–Eur. J., 2015, 21, 1 CrossRef PubMed.
- T. Wang, X. Q. Tao, Y. Xiao, G. H. Qiu, Y. Yang and B. X. Li, Catal. Sci. Technol., 2020, 10, 138–146 RSC.
- M. M. Wang, H. H. Wu, C. R. Shen, S. P. Luo, D. Wang and L. He, ChemCatChem, 2019, 11, 1935–1942 CrossRef CAS.
Footnote |
† Electronic supplementary information (ESI) available. See DOI: 10.1039/d0ra03164j |
|
This journal is © The Royal Society of Chemistry 2020 |
Click here to see how this site uses Cookies. View our privacy policy here.