DOI:
10.1039/D0RA02766A
(Paper)
RSC Adv., 2020,
10, 19371-19381
MoS42− intercalated NiFeTi LDH as an efficient and selective adsorbent for elimination of heavy metals
Received
26th March 2020
, Accepted 10th May 2020
First published on 20th May 2020
Abstract
The enormous increase of heavy metal pollution has led to a rise in demand for synthesizing efficient and stable adsorbents for its treatment. Therefore, we have designed a novel adsorbent by introducing (MoS4)2− moieties within the layers of NiFeTi LDH-NO3, via an ion exchange mechanism, as a stable and efficient adsorbent to deal with the increasing water pollution due to heavy metals. Characterization techniques such as XRD, FTIR, TGA, SEM, TEM, and Raman spectroscopy were used to confirm the formation of (MoS4)2− intercalated NiFeTi LDH and structural changes after the adsorption process. The efficiency of the material was tested with six heavy metal ions, among which it was found to be effective for toxic Pb2+ and Ag+ ions. When selectivity was studied with all six of the metal ions copresent in one solution, the material showed greater selectivity for Pb2+ and Ag+ ions with the selectivity order of Ni2+ < Cu2+ < Zn2+ < Fe3+ < Pb2+ < Ag+, with great adsorption capacities of 653 mg g−1 for Pb2+ and 856 mg g−1 for Ag+ metal ions. Further, the kinetics adsorption study for both the metal ions had a great correlation with the pseudo-second-order model and supported the chemisorption process via the formation of M–S bonding. The adsorption process obeyed the Langmuir model. Therefore, the MoS4-LDH material could be a promising adsorbent for the removal of heavy metals.
1. Introduction
According to a recent survey by the GOES organization, water pollution is considered to be one of the leading environmental problems and could be a threat to existence of life on the planet in the upcoming decades.1 Therefore, it has become a significant concern for various societies.2 Direct emission of an enormous number of pollutants (such as dyes, pharmaceutical compounds, organic compounds, heavy metals, etc.) into water resources is the main root of water contamination.3,4 Among the listed pollutants, pollution caused by heavy metals has become a fundamental environmental issue in environmental remediation and separation science due to its harmful effects on human health and the ecological environment.5
Heavy metals are considered to be those metals in chemistry that have higher atomic weight, atomic number, and a density greater than 5 g cm−3.6 They are further classified as toxic, precious, and radioactive metals.7 Heavy metals with characteristics of non-biodegradability, carcinogenicity and mutagenesis, accumulate in the food chain and subsequently harm the human beings and other living bodies.8 They are basically discarded by various industrial activities, for instance, electronics plating, metal finishing, textiles, metallurgy, mining, battery manufacturing, tanning, and chemical manufacturing.9 Among all heavy metals, lead (Pb), mercury (Hg), chromium (Cr), cadmium (Cd), arsenic (As), zinc (Zn), copper (Cu), tin (Sn), nickel (Ni), silver (Ag) and cobalt (Co) are the most toxic ones.10 However, heavy metals such as zinc and copper are also essential elements when present at lower concentrations but harmful when present at higher levels. Among the listed toxic metals, lead (Pb) is ranked as the second most hazardous element, and lead poisoning directly affects the liver, kidney, gastrointestinal system, and central nervous system.11 Moreover, direct or indirect long-term lead exposure may cause hepatitis, anemia, nephritic syndrome, encephalopathy, brain damage, brain swelling, and also death.12 Lead poisoning also causes mental disabilities and behavioral fluctuations, mainly in children and nearly 143
000 deaths annually in developing countries.13 As a result, it has become imperative to eliminate such hazardous metal ions from wastewater before its disposal to water bodies.
Numerous methods such as ion exchange, electrical coagulation, flotation, bio-sorption, flocculation treatment, chemical precipitation, filtration membrane, and adsorption have been used worldwide for heavy metals removal and recovery from wastewater.14–21 Among these treatment methods, adsorption is considered to be more promising because of its strong operability, low cost, and simple design.22 Several synthetic and natural adsorbents such as activated carbon, zeolites, biomaterials, sorption resins, and polymers have been brought in the application for efficient removal of heavy metals.19–30 Clays with characteristics of high surface area, low cost, and hydrophilicity have gained attraction as natural adsorbents for heavy metal ions treatment.
Layered double hydroxides (LDHs), a type of anionic clays, comprises of positively charged layers intercalated with counter anions. The outstanding intercalated anion exchange properties of LDHs allow it to show multipurpose applications in the field of 2-D nanoreactors, catalysts, scavengers, and adsorbents.31–36 Also, sulfides are known to form strong heavy metals-sulfides covalent bonds, which can be very beneficial in designing new efficient adsorbent materials for heavy metal capture from heavy metals contaminated wastewater.37,38 With the attractive applications of LDHs and sulfide containing groups, we can assume that after functionalizing LDHs with the sulfide containing groups, a single-phase having the advantages of both the materials (oxides and sulfides) would be acquired with enriched properties of heavy metals contaminated wastewater remediation. Previously, Ma and coworkers42 have fabricated a novel adsorbent for the removal of heavy metals by intercalating MoS42− ions in binary MgAl LDH, and the same was characterized by using various techniques confirming the intercalation of MoS42− ions in the layers of binary MgAl LDH. They employed the same for the uptake of heavy metals for wastewater.42
Our research group has previously fabricated a ternary NiFeTi LDH and applied it for the elimination of various anionic dyes from wastewater.39 Herein, we have designed a novel modified LDH form by functionalizing ternary NiFeTi LDH with (MoS4)2− ions in the interlayers and investigated its ability of heavy metal removal from the wastewater system. The ascribed MoS4-intercalated LDH material exhibits excellent adsorption ability for Pb2+ and Ag+ at a fast rate as compared to previously used binary LDH moieties for such uptakes. Therefore, the ability to reduce heavy metal concentrations below <5 ppb levels makes MoS4-LDH a good alternative for wastewater remediation in the future.
2. Experimental section
2.1 Materials
NiFeTi–CO3 LDH was reproduced using a previously reported hydrothermal method.39 NiFeTi–CO3 LDH was further converted into NiFeTi–NO3 LDH by using the ion-exchange method.40 Ammonium tetrathiomolybdate ((NH4)2MoS4) was obtained from SigmaAldrich. Also, NO3− anion from NiFeTi–NO3 LDH was replaced by (MoS4)2− anion to acquire brown colored MoS4-LDH. For (MoS4)2− anion exchange, 0.3 g NiFeTi–NO3 LDH and 0.3 g (NH4)2MoS4 were dispersed and further stirred for 36 hours in 20 mL of deionized water at ambient temperature. The obtained solid was filtered, also washed with degassed water, and then with acetone. The resulting material was air-dried to get brown colored MoS4-LDH.
2.2 Uptake of heavy metals
The uptake studies of heavy metals from aqueous solutions at various concentrations were carried out using the batch method. At the initial stage, heavy metal uptake experiments were carried for six metal ions (Ag+, Pb2+, Zn2+, Fe3+, Cu2+, and Ni2+) coming from their corresponding nitrate salts. The solid adsorbent was dispersed in metal ion solutions for a fixed duration of time, followed by centrifugation to separate the solid sorbent, and finally, the metal ion concentrations were determined from their respective supernatant solutions by using atomic absorption spectroscopy (AAS). The difference in the metal concentrations of mother solutions and supernatant solutions was used to evaluate the adsorption capacity. The distribution coefficient (Kd) is estimated by using the given equation: |
Kd = (V[(C0 − Cf)/Cf])/m
| (1) |
where C0 represents initial concentrations of respective Mn+ ion (ppm), Cf denotes the final concentrations of respective Mn+ ion (ppm) after contact, m represents the amount of solid adsorbent in g and V stands for volume of the solution used for adsorption process.41 The heavy metal removal% is determined by |
% Removal = 100 × (C0 − Cf)/C0
| (2) |
The removal capacity of adsorbent (qm) is obtained by
|
qm = ((C0 − Cf)V/m) × 10−3
| (3) |
The adsorption studies for the heavy metals (except for Ag) were carried out at v/m ratio = 3846 mL g−1 and for Ag at 8333 mL g−1 because when batch experiments were employed for Ag at v/m ratio of 3846 mL g−1, the efficiency of synthesized material for the uptake of Ag ions was very much fast and showed 100% uptake efficiency within 5 min of experiment and became difficult to evaluate the kinetic study. Further, the high selectivity for Ag+ and Pb2+ was determined by using a mixture of all the metal ions together with ∼100 ppm initial concentration for each ion. Experiments were also carried out to examine the removal capacity (qm) for Ag+ and Pb2+ by varying the initial metal ion concentrations at ambient temperature for a contact time of 2 hours. The acquired data was used for determining the adsorption isotherms.
2.3 Kinetics study
Adsorption kinetic study was performed for Ag+ and Pb2+ ions at different adsorption times (5–120 min). For the kinetic study of Ag+ ion, 0.006 g of sorbent was dispersed in 50 mL of 100 ppm Ag solution with a v/m ratio of 8333 mL g−1. After fixed time intervals, suspensions were drawn out, centrifuged, and ion content was analyzed using AAS. Similar kinetic experiment was performed for Pb2+ with 0.013 g of sorbent for 50 mL of 100 ppm Pb2+ solution (v/m = 3846 mL g−1).
2.4 Characterization techniques
The XRD patterns of synthesized materials were collected using an X-ray diffractometer (Model no. D8 DISCOVER). Fourier transformed infrared (FT-IR) spectra were recorded on IRAffinity-1S FTIR Shimadzu. Morphology was determined by JEOL JSM 6610 SEM with an accelerating voltage of 30 kV and by TECNAI 200 kV HRTEM (Fei, Electron Optics). BET specific surface area of LDH was determined using Autosorb iQ Station 1, Quantachrome Instruments by Brunauer–Emmett–Teller (BET) method. The metal ion concentrations were determined using ZEEnit 700 Atom Absorption Spectrometer.
3. Results and discussion
3.1 Characterization of synthesized MoS4-LDH
The obtained brown colored MoS4 LDH was characterized thoroughly using various characterization techniques such as XRD, FTIR, SEM, TEM, BET, Raman, and TGA.
The XRD patterns of NO3 LDH and the ion-exchanged product MoS4 LDH is depicted in Fig. 1. The comparison of basal spacing of NO3 LDH (dbasal = 0.80 nm) (Fig. 1a) with the enlarged MoS4 LDH spacing (dbasal = 1.10 nm) (Fig. 1b), confirms the exchange of NO3-ions with MoS4-ions in the interlayer spacing of LDH. The layered phase is approved by the existence of (00l) reflections at 1.10, 0.61 and 0.35 nm. The lower intensity of (003) reflection at 1.10 nm as compared to that of (006) reflection at 0.61 nm could be attributed to the heavy nature and substantial scattering property of MoS4-ions intercalated in the layers of LDH.42,51
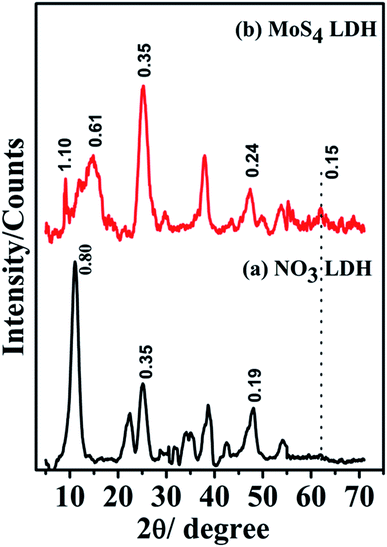 |
| Fig. 1 XRD patterns of (a) NO3 LDH and (b) MoS4 LDH. | |
SEM and HRTEM images are depicted in Fig. 2. SEM images illustrate the layered structures of MoS42− intercalated NiFeTi LDH. SEM images clearly show that the layered structure of MoS4 LDH resembles the CO3-LDH, as reported in our previous work.39
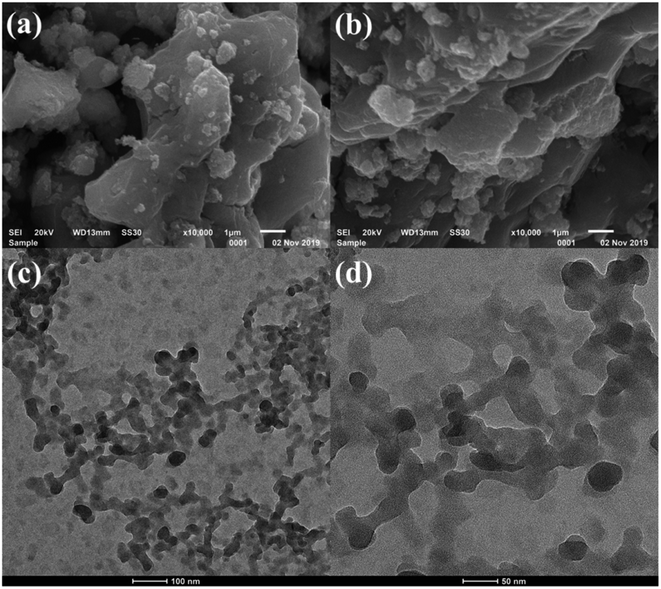 |
| Fig. 2 SEM (a and b) and TEM (c and d) images of MoS4 LDH. | |
The intercalation of MoS4-ions via the exchange of NO3− ions from interlayers is confirmed by FTIR analysis. Fig. 3a illustrates the spectrum of NO3-LDH, which confirms the formation of NO3− intercalated LDH. Strong band appearing at 1381 cm−1 could be attributed to the stretching vibration of nitrate ion in the layers of LDH. A broad band at 3393 cm−1 is assigned to the stretching vibration occurring because of the O–H group of interlayered water molecules and metal bonded hydroxyl groups. The existence of a weaker band at 1638 cm−1 could be assigned to the O–H bending vibration of interlayered hydroxyl and water molecules. Fig. 3b illustrates the FTIR spectrum of MoS4 LDH. The complete disappearance of NO3− band at 1381 cm−1 confirms the entire exchange of NO3− ions by MoS42− ions. In the case of free (NH4)2MoS4 (Fig. 3c), the appearance of the band at 476 cm−1 is allotted to the Mo–S vibration. The band at 3018 cm−1 could be assigned to the N–H mode of ammonium group (NH4+). The absorption band at 3484 cm−1 could have corresponded to the hydroxyl stretching vibration (O–H) of water molecules. The bands existing at 1392 and 1633 cm−1 might be attributed to the in-plane N–H bending mode.39,42,43
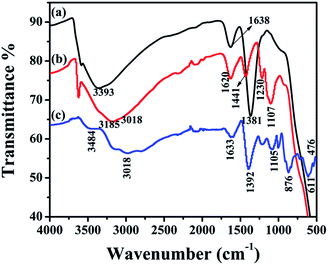 |
| Fig. 3 FTIR spectra of (a) NO3-LDH and (b) MoS4-LDH and (c) (NH4)2MoS4. | |
The thermal gravimetric analysis (TGA) is depicted in Fig. 4a. The initial weight loss at temperature range 100–150 °C is attributed to the elimination of interlayered water molecules (approximately 10% weight loss). The subsequent degradation till 330 °C is assigned to the partial loss of MoS42− from MoS4 LDH (31.57% weight loss). The complete elimination of MoS42− from MoS4 LDH occur up to 600 °C (weight loss (water molecules + MoS4 ions) = 42.31%).44
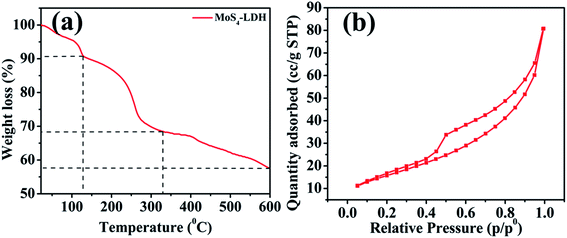 |
| Fig. 4 TGA (a) and BET (b) spectra of MoS4-LDH. | |
Fig. 4b illustrates the N2 adsorption–desorption isotherm carried out to study the porosity of MoS4 LDH. From the BJH data, the surface area was found to be 64.66 m2 g−1. The pore volume and pore diameter were found to be 0.122 cm3 g−1 and 3.832 nm, respectively. The isotherm type confirms the formation of mesopores.
Raman spectra of designed material and pure NH4MoS4 are depicted in Fig. 5. The Raman spectra were found to be very much helpful in studying the details of stretching bands due to Mo–S bonds. The pure form of NH4MoS4 clearly showed two peaks at 455 and 476 cm−1, due to the Mo–S bond stretching. Whereas, the interaction of the intercalated MoS42− with the hydroxides of LDH via hydrogen bonding results into a redshift due to which the bands occurs at 361 and 387 cm−1.42
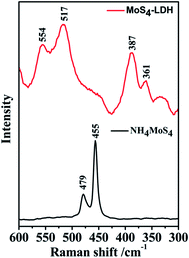 |
| Fig. 5 Raman spectra of MoS4-LDH. | |
From all the shreds of evidence obtained from various characterizations, it could be stated that the NO3-LDH was successfully converted into more negatively charged MoS4-LDH.
3.2 Heavy metal uptake using MoS4 LDH
The removal of heavy metals from aqueous solution by MoS4-LDH adsorbent was examined using batch method experiments. The MoS4-LDH affinity towards employed heavy metals was evaluated in the form of a distribution coefficient (Kd). The adsorption study was carried out using individual heavy metal solutions such as Ag+, Pb2+, Zn2+, Fe3+, Cu2+, and Ni2+ and mixture solution containing all the six ions together. The obtained adsorption results for individual ions studies are illustrated in Table 1. From the results, it could be stated that MoS4-LDH showed a greater ability for Ag+ and Pb2+ as compared to other ions. Both the metal ions were removed entirely from 100 ppm metal ion concentrated solution with Kd values higher than 105. In contrast, MoS4-LDH showed low adsorption capacity for other heavy metals, which makes it one of a suitable method for the elimination of such ions.
Table 1 Adsorption results toward individual ionsa
Entry |
Single ions |
Initial conc. (ppm) |
Final conc. (ppm) |
Mn+ removal (%) |
Kd (mL g−1) |
Ion concentration 100 ppm per ion (approx.), contact time = 90 min, V = 10 mL, m (mass of solid sample) = 0.0026 g, v/m ratio = 3846 mL g−1. m (mass of solid sample) = 0.0012 g, v/m ratio = 8333 mL g−1. |
1 |
Pb2+ |
100 |
0.4392 |
99.56 |
8.7 × 105 |
2 |
Zn2+ |
100 |
17.25 |
82.75 |
1.8 × 104 |
3 |
Ni2+ |
100 |
42.74 |
57.26 |
5.2 × 103 |
4 |
Fe3+ |
100 |
29.49 |
70.51 |
9.2 × 103 |
5b |
Ag+ |
100 |
0.001 |
100 |
8.3 × 109 |
6 |
Cu2+ |
100 |
74.08 |
25 |
1.3 × 103 |
Table 2 illustrates the competitive adsorption results with all six ions in one solution. From the results, the selectivity order was obtained to be Ni2+ < Cu2+ < Zn2+ < Fe3+ < Pb2+ < Ag+, and it can be stated that MoS4-LDH showed high selectivity for soft Lewis acid metal ions. As compared to the results obtained from individual ions removal tests, Pb2+ and Ag+ were found to be in high correlation, except for other ions. Hence, it can be said that ascribed material can rapidly eliminate heavy metals by reducing the concentration of soft Lewis heavy metals from aqueous solution by trapping the heavy metal ion via M–S coordination bond formation. All the results point out towards great potential of designed MoS4-LDH as an efficient adsorbent for purifying heavy metals polluted water.
Table 2 Adsorption results toward mixed ionsa
Entry |
Mixed ions |
Initial conc. (ppm) |
Final conc. (ppm) |
Mn+ removal (%) |
Kd (mL g−1) |
Ion concentration ∼ 100 ppm per ion (approx.), contact time = 90 min, V = 50 mL, m (mass of solid sample) = 0.013 g, v/m ratio = 3846 mL g−1. |
1 |
Pb2+ |
128.9 |
4.7 |
96.35 |
1.01 × 105 |
2 |
Zn2+ |
108.36 |
87.18 |
19.54 |
934.40 |
3 |
Ni2+ |
83.66 |
76.5 |
8.55 |
359.97 |
4 |
Fe3+ |
85.97 |
55.76 |
35.14 |
2.08 × 103 |
5 |
Ag+ |
128.1 |
3.358 |
97.37 |
1.40 × 105 |
6 |
Cu2+ |
98.5 |
82.725 |
16.015 |
733.43 |
Adsorbent amount variation study was also evaluated for both metal ions (Ag+ and Pb2+), and results are depicted in Fig. 6a. From the study, it could be clearly stated that for the elimination of Pb2+ pollutants from wastewater, 0.0026 g of adsorbent was sufficient for complete removal of Pb2+ from 100 ppm sample and for Ag+ removal 0.0012 g of MoS4-LDH was appropriate for complete elimination from 100 ppm sample.
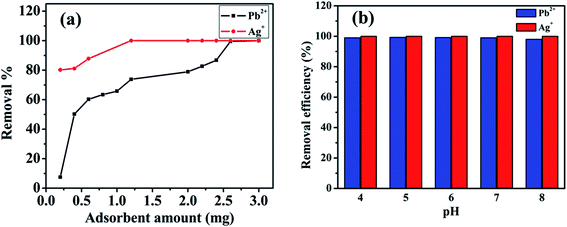 |
| Fig. 6 (a) Adsorbent amount variation study for Pb2+ and Ag+ removal, (b) effect of pH on removal efficiency. | |
Further, the pH variation was also evaluated for Pb2+ and Ag+ (varying from 4 to 8) by using 0.1 N HCl and 0.1 N NaOH solutions. Interestingly, it was found that no significant change in the removal efficiency was observed for both the cases (Pb2+ and Ag+) when pH of the solution was varied from 4 to 8 (Fig. 6b).
3.3 Kinetic study
The adsorption kinetic study was examined for heavy metal ions, which are depicted in Fig. 7 (for Pb2+ and Ag+) to determine the adsorption rate and its pathway towards equilibrium. The sorption rates for the elimination of Pb2+ and Ag+ from respective individual solutions were found to be very fast, as depicted in Fig. 7a. MoS4-LDH efficiently removed >90% of Pb2+ within 5 min and 98% of Ag+ within 40 min. The adsorption equilibrium was attained within 80 min for all the employed heavy metals pollutants. The adsorption rate was estimated with the help of two different kinetic rate equations: pseudo-first-order and pseudo-second-order kinetics rate equations which can be defined as: |
 | (4) |
|
 | (5) |
where qe (mg g−1) represents the amount of heavy metal adsorbed at equilibrium stage, qt (mg g−1) is the amount of heavy metal adsorbed at time t, k1 (min−1) is the rate constant for pseudo-first-order kinetic rate equation, k2 (g mg−1 min−1) denotes the rate constant for the pseudo-second-order kinetic equation. The values of k1 and k2 were obtained by linear plots: ln(qe − qt) vs. t and t/qt against t, respectively. The kinetic parameters obtained from the linear kinetic plots for Pb2+ and Ag+ are summarized in Table 3. The pseudo-first-order linear plots are depicted in Fig. 7b and c, and the pseudo-second-order linear kinetic plots for adsorption of Pb2+ and Ag+ are depicted in Fig. 7e and f. From the kinetic plots of the metal ions, it could be easily stated that the adsorption process follows the pseudo-second-order rate equation and the correlation coefficient (R2) is close to one, which suggests that the process involved is chemisorption.42,50 The kinetic data displays that the adsorption of Ag+ ions attained the equilibrium faster than Pb2+ ions.
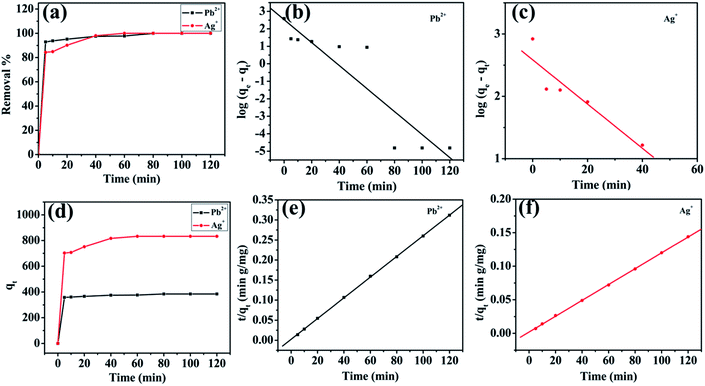 |
| Fig. 7 Kinetics plots for Pb2+ and Ag+ removal: (a) contact time effect on removal%, (b) pseudo-first order plot for Pb2+ removal, (c) pseudo-first order plot for Ag+ removal, (d) adsorption capacity (qt) as a function of time, (e) pseudo-second order plot for Pb2+ removal, (f) pseudo-second order plot for Ag+ removal. | |
Table 3 Kinetic parameters toward individual ions adsorption (Pb2+ and Ag+) over MoS4-LDH
Adsorbate |
Pseudo-first order |
Pseudo-second order |
Calculated qe (mg g−1) |
qe (mg g−1) |
k1 (min −1) |
R2 |
qe (mg g−1) |
k2 (g mg−1 min−1) |
R2 |
Pb2+ |
337.427 |
0.151 |
0.91 |
387.59 |
2.7 × 10−3 |
0.999 |
385.23 |
Ag+ |
385.363 |
0.0817 |
0.92 |
847.48 |
7.2 × 10−4 |
0.999 |
839.16 |
3.4 Adsorption isotherm study
Selective studies clearly illustrated that the MoS4-LDH was highly efficient for the removal of Ag+ and Pb2+. Further, the adsorption isotherm study was used to estimate the maximum sorption capacity of MoS4 LDH for Ag+ and Pb2+. From the studies, it was witnessed that Ag+ and Pb2+ capture by MoS4 LDH increased successively when the concentration was increased (50–500 ppm). The percentage of heavy metals removed as a function of the concentration of Ag+ and Pb2+ is depicted in Fig. 8a and b. From the obtained results, the ascribed material effectively captured Ag+ and Pb2+ over a broad range of concentrations. The maximum removal capacity (qm) reached 856 and 653 mg g−1 for Ag+ and Pb2+, respectively which is extraordinarily higher than the finest adsorbents for Ag+ removal such as magnetic cellulose xanthate (166 mg g−1),42 S4-LDH (383 mg g−1)46 and Mg/Al–MoS4-LDH (450 mg g−1).42 Similarly, the obtained qm value for Pb2+ is exceptionally higher than the previously reported adsorbents employed for the uptake of lead ion from contaminated water such as Mg/Al–MoS4-LDH (290 mg g−1),42 DTPA-LDH (170 mg g−1)47 and MNP–CTS (140 mg g−1).48 The comparison of the obtained qm values of the ascribed material is depicted in Table 4.42,45–49 From the comparison table, it can be stated that the synthesized MoS4-LDH illustrates excellent elimination capacities for heavy metal ions, which could be attributed to the intercalation of MoS42− in the gallery of NiFeTi-LDH.
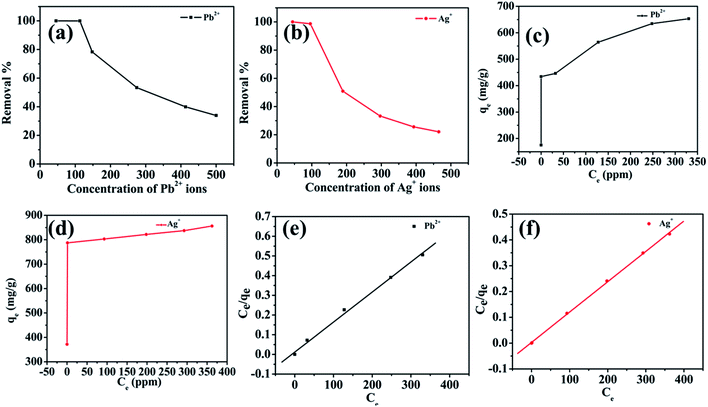 |
| Fig. 8 Isotherm plots for Pb2+ and Ag+ removal: (a) removal% with respect to concentration variation of Pb2+ ions, (b) removal% with respect to concentration variation of Ag+ ions, (c) sorption isotherm for Pb2+ ions, (d) sorption isotherm for Ag+ ions, (e) Langmuir isotherm model for Pb2+ removal, (f) Langmuir isotherm model for Ag+ removal. | |
Table 4 Comparison of maximum adsorption capacities of MoS4 LDH with different adsorbents
Adsorbents |
Adsorbates |
qmax (mg g−1) |
References |
Magnetic cellulose xanthate |
Ag+ |
166 |
45 |
S4-LDH |
Ag+ |
383 |
46 |
Mg/Al–MoS4-LDH |
Ag+ |
450 |
42 |
KMS-2 |
Ag+ |
408 |
49 |
Ni/Fe/Ti–MoS4-LDH |
Ag+ |
856 |
Present study |
Mg/Al–MoS4-LDH |
Pb2+ |
290 |
42 |
DTPA-LDH |
Pb2+ |
170 |
47 |
MNP–CTS |
Pb2+ |
140 |
48 |
Ni/Fe/Ti–MoS4-LDH |
Pb2+ |
653 |
Present study |
Langmuir isotherm model was also used to evaluate the data in which adsorbate is assumed to form monolayer coverage on the surface of the adsorbent, and once such coverage is formed, no further sorption could occur at that active site. The Langmuir isotherm is defined by the following equation:
|
 | (6) |
where
qe (mg g
−1) denotes adsorption capacity after achieving equilibrium,
qm (mg g
−1) represents theoretical maximum adsorption capacity,
Ce (mg L
−1) denotes heavy metal ion equilibrium concentration, and
b (L mg
−1) is the Langmuir constant. The equilibrium adsorption capacity as a function of equilibrium concentrations for both the ions are depicted in
Fig. 8c and d. Also, the Langmuir isotherms are depicted in
Fig. 8e and f. The data for both the heavy metal ions were found to be in great correlation with the Langmuir model and theoretically obtained
qm values (848 (mg g
−1) for Ag
+ and 653 (mg g
−1) for Pb
2+) are approximately equal to the obtained experimental values. The Langmuir parameters are summarized in
Table 5. Correlation coefficient approaching unity (
R2 > 0.99) shows a linearly well-fitted curve with the Langmuir model, which confirms the monolayer adsorption and no occurrence of transmigration in the adsorbates.
44
Table 5 Langmuir and Freudlich parameters
Adsorbates |
Langmuir model |
Freundlich model |
qm (mg g−1) |
b (L mg−1) |
R2 |
Kf (mg g−1) (L mg−1)1/n |
1/n |
R2 |
Pb2+ |
654 |
0.134 |
0.998 |
490.90 |
0.032 |
0.76 |
Ag+ |
848 |
0.011 |
0.999 |
616.59 |
0.06 |
0.93 |
Further, the Freundlich isotherm model is also evaluated. In general, this model is based on the assumption that the non-uniform distribution of heat occurred over a heterogeneous surface and is represented as:
|
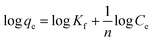 | (7) |
where
qe and
Ce have their usual meanings, and
Kf and
n denote Freundlich constants. These constants are obtained from the intercept and slope of a linear plot between log
qe and log
Ce. The linear Freundlich fitting is illustrated in
Fig. 9, and the parameters are clubbed in
Table 5. From the summarized data in
Table 5, it can be claimed that the Langmuir model was in high correlation for both the metal ions. Hence, it could be stated that the metal adsorption is a monolayer.
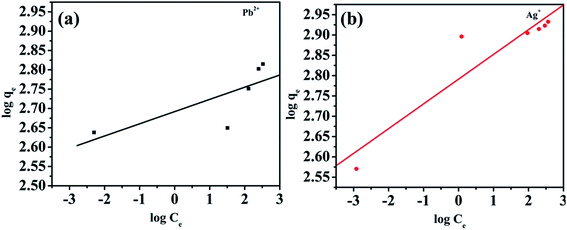 |
| Fig. 9 (a) Freundlich isotherm model for Pb2+ removal, (b) Freundlich isotherm model for Ag+ removal. | |
3.5 Adsorbent stability after heavy metal ion adsorption
After the sorption study, the adsorbent was recollected using the centrifugation technique, and the stability of MoS4-LDH and adsorption were analyzed by FTIR spectroscopy (depicted in Fig. 10). The band which occurred at 1360 cm−1 in the spectrum of NO3-LDH, which is the characteristic band of nitrate ion was absent in all the six samples after metal ion adsorption, which indicates that no NO3− ions accompanied the heavy metal ions during the adsorption process. The constant vibrations at 1628, 1422, 1233, and 1105 cm−1 imply the LDH stability during the sorption process.42 Based on previously reported studies and the complexation chemistry of (MoS4)2− ions, three mechanisms could be held responsible for such metal ion capture. The first mechanism is based on the condition when lower content of metal ions is present, and adsorbent is present in large concentration. Under such circumstance, the amount of active intercalated (MoS4)2− sites are much higher than the metal ion (Mn+), and the excess (MoS4)2− ions combine with Mn+ resulting into the formation of complex moiety such as [M(MoS4)2]2− (when the charge on the metal ion is 2), which retains within the LDH layers. The second mechanism occurs when both the ions are present in almost equal amounts. In such a case, (MoS4)2− ions are still present in excess quantity compared to the M2+ ions, and [M(MoS4)2]2− complex is dominant with an almost equal amount of LDH-NO3. The third mechanism occurs when the metal cations are present in excess amount and the LDH-(MoS4)2− gets saturated with the coordinated metal ions. Hence, the formation of amorphous neutral salt occurs along with the intercalated nitrate phase.42
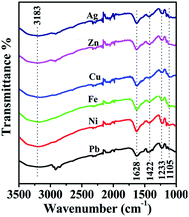 |
| Fig. 10 FTIR spectra of MoS4-LDH after metal ions adsorption. | |
4. Conclusion
MoS4-LDH follows ion-exchange chemistry, during the formation process by replacing the nitrate ions with (MoS4)2− ions in the layer spacing of hydrothermally synthesized NiFeTi LDH. Due to the tremendous increase in pollution caused by heavy metals and the scarcity of available techniques, it has become essential to design an efficient adsorbent for heavy metals uptake. Sulfide groups present in the (MoS4)2− ions show excellent uptake towards soft Lewis metal ions due to their soft Lewis basic properties. When all the heavy metal ions are present in aqueous solution, the material showed greater selectivity for Pb2+ and Ag+ ions (Ni2+ < Cu2+ < Zn2+ < Fe3+ < Pb2+ < Ag+) and high adsorption capacities, 653 and 856 mg g−1, for Pb2+ and Ag+ ions, respectively. The adsorption was found to obey the pseudo-second-order model towards Pb2+ and Ag+ ions supporting the chemisorption process via the formation of M–S bonding. All the above-mentioned features suggest ternary MoS4-LDH material be very efficient for the removal of heavy metals from polluted water.
Conflicts of interest
The authors have no conflict regarding the publication of the paper.
Acknowledgements
GR and RC are highly thankful to the University of Delhi for providing financial support.
References
- D. Howard, We have 10 years to save the seas, Global Oceanic Environmental Survey, September 1, 2019 Search PubMed.
- G. Salehi, R. Abazari and A. R. Mahjoub, Visible-Light-Induced Graphitic-C3N4@Nickel-Aluminum Layered Double Hydroxide Nanocomposites with Enhanced Photocatalytic Activity for Removal of Dyes in Water, Inorg. Chem., 2018, 57, 8681–8691 CrossRef CAS PubMed.
- D. Li, S. Zeng, M. He and A. Z. Gu, Water Disinfection Byproducts Induce Antibiotic Resistance-Role of Environmental Pollutants in Resistance Phenomena, Environ. Sci. Technol., 2016, 50, 3193–3201 CrossRef CAS PubMed.
- X. Gong, D. Huang, Y. Liu, G. Zeng, R. Wang, J. Wei, C. Huang, P. Xu, J. Wan and C. Zhang, Pyrolysis and reutilization of plant residues after phytoremediation of heavy metals contaminated sediments: for heavy metals stabilization and dye adsorption, Bioresour. Technol., 2018, 253, 64–71 CrossRef CAS PubMed.
- H. He, Z. Xiang, H. Chen, X. Chen, H. Huang, M. Wen and C. Yang, Biosorption of Cd(II) from synthetic wastewater using dry biofilms from biotrickling filters, Int. J. Environ. Sci. Technol., 2018, 15, 1491–1500 CrossRef CAS.
- O. Pourret and J. C. Bollinger, What to do now: to use or not to use?, Sci. Total Environ., 2018, 610, 419–420 CrossRef PubMed.
- B. K. Bansod, T. Kumar, R. Thakur and S. Rana, A review on various electrochemical techniques for heavy metal ions detection with different sensing platforms, Biosens. Bioelectron., 2017, 94, 443–455 CrossRef CAS PubMed.
- X. Gong, D. Huang, Y. Liu, G. Zeng, R. Wang, J. Wan, C. Zhang, M. Cheng, X. Qin and W. Xue, Stabilized nanoscale zerovalent iron mediated cadmium accumulation and oxidative damage of Boehmeria nivea (L.) gaudich cultivated in cadmium contaminated sediments, Environ. Sci. Technol., 2017, 51, 11308–11316 CrossRef CAS PubMed.
- F. Fu and W. Qi, Removal of heavy metal ions from wastewaters: a review, J. Environ. Manag., 2011, 92, 407–418 CrossRef CAS PubMed.
- G. Liu, X. Chai, Y. Shao, L. Hu, Q. Xie and H. Wu, Toxicity of copper, lead, and cadmium on the motility of two marine microalgae Isochrysis galbana and Tetraselmis chui, J. Environ. Sci., 2011, 23, 330–335 CrossRef CAS.
- Ö. Gerçel and H. F. Gerçel, Adsorption of lead(II) ions from aqueous solutions by activated carbon prepared from biomass plant material of Euphorbia rigida, Chem. Eng. J., 2007, 132, 289–297 CrossRef.
- M. Momčilović, M. Purenović, A. Bojić, A. Zarubica and M. Ranđelović, Removal of lead(II) ions from aqueous solutions by adsorption onto pine cone activated carbon, Desalination, 2011, 276, 53–59 CrossRef.
- N. Muhammad, N. Banooria, A. Akbarb, A. Azizullah, M. Khan, M. Qasim and H. Rahman, Microbial and toxic metal contamination in well drinking water: potential health risk in selected areas of Kohat, Pakistan, Urban Water J., 2017, 14, 394–400 CrossRef CAS.
- Z. Xia, L. Baird, N. Zimmerman and M. Yeager, Heavy metal ion removal by thiol functionalized aluminum oxide hydroxide nanowhiskers, Appl. Surf. Sci., 2017, 416, 565–573 CrossRef CAS.
- M. Nemati, S. M. Hosseini and M. Shabanian, Novel electrodialysis cation exchange membrane prepared by 2-acrylamido-2-methylpropane sulfonic acid; heavy metal ions, J. Hazard. Mater., 2017, 337, 90–104 CrossRef CAS PubMed.
- G. G. Aregay, A. Jawad, Y. Du, A. Shahzad and Z. Chen, Efficient and selective removal of chromium(VI) by sulfide assembled hydrotalcite compounds through concurrent reduction and adsorption processes, J. Mol. Liq., 2019, 294, 111532 CrossRef CAS.
- T. K. Tran, K. F. Chiu, C. Y. Lin and H. J. Leu, Electrochemical treatment of wastewater: selectivity of the heavy metals removal process, Int. J. Hydrogen Energy, 2017, 10, 27741–27748 CrossRef.
- J. Guo, Y. Kang and Y. Feng, Bioassessment of heavy metal toxicity and enhancement of heavy metal removal by sulfate-reducing bacteria in the presence of zero valent iron, J. Environ. Manage., 2017, 203, 278–285 CrossRef CAS PubMed.
- R. Kumar, G. B. V. S. Lakshmi, K. Singh and P. R. Solanki, A novel approach towards optical detection and detoxification of Cr(VI) to Cr(III) using L-Cys-VS2QDs, J. Environ. Chem. Eng., 2019, 7, 103202 CrossRef CAS.
- N. Chaudhary, P. K. Gupta, S. Eremin and P. R. Solanki, One-step green approach to synthesize highly fluorescent carbon quantum dots from banana juice for selective detection of copper ions, J. Environ. Chem. Eng., 2020, 8, 103720 CrossRef CAS.
- S. Verma and R. K. Dutta, Development of cysteine amide reduced graphene oxide (CARGO) nano-adsorbents for enhanced uranyl ions removal from aqueous medium, J. Environ. Chem. Eng., 2017, 5, 4547–4558 CrossRef CAS.
- X. H. Wang, W. Y. Deng, Y. Y. Xie and C. Y. Wang, Selective removal of mercury ions using a chitosan–poly(vinyl alcohol) hydrogel adsorbent with three-dimensional network structure, Chem. Eng. J., 2013, 228, 232–242 CrossRef CAS.
- G. Blanchard, M. Maunaye and G. Martin, Removal of heavy metals from waters by means of natural zeolites, Water Res., 1984, 18(12), 1501–1507 CrossRef CAS.
- D. Mohan and P. S. Kunwar, Single-and multi-component adsorption of cadmium and zinc using activated carbon derived from bagasse—an agricultural waste, Water Res., 2002, 36, 2304–2318 CrossRef CAS PubMed.
- X. Zhao, Q. Jia, N. Song, W. Zhou and Y. Li, Adsorption of Pb(II) from an aqueous solution by titanium dioxide/carbon nanotube nanocomposites: kinetics, thermodynamics, and isotherms, J. Chem. Eng. Data, 2010, 55, 4428–4433 CrossRef CAS.
- W. Du, L. Yin, Y. Zhuo, Q. Xu, L. Zhang and C. Chen, Catalytic oxidation and adsorption of elemental mercury over CuCl2-impregnated sorbents, Ind. Eng. Chem. Res., 2014, 53, 582–591 CrossRef CAS.
- J. Liu, Y. Ma, T. Xu and G. Shao, Preparation of zwitterionic hybrid polymer and its application for the removal of heavy metal ions from water, J. Hazard. Mater., 2010, 178, 1021–1029 CrossRef CAS PubMed.
- A. B. Albadarin, H. Ala'a, N. A. Al-Laqtah, G. M. Walker, S. J. Allen and M. N. M. Ahmad, Biosorption of toxic chromium from aqueous phase by lignin: mechanism, effect of other metal ions and salts, Chem. Eng. J., 2011, 169, 20–30 CrossRef CAS.
- V. Tharanitharan and K. Srinivasan, Kinetic and equilibrium studies of removal of pb(ii) and cd(ii) ions from aqueous solution by modified duolite XAD-761 resins, Asian J. Chem., 2010, 22, 3036 CAS.
- A. Benhammou, A. Yaacoubi, L. Nibou and B. Tanouti, Adsorption of metal ions onto Moroccan stevensite: kinetic and isotherm studies, J. Colloid Interface Sci., 2005, 282, 320–326 CrossRef CAS PubMed.
- A. I. Khan and D. O'Hare, Intercalation chemistry of layered double hydroxides: recent developments and applications, J. Mater. Chem., 2002, 12, 3191–3198 RSC.
- V. R. L. Constantino and T. J. Pinnavaia, Structure-reactivity relationships for basic catalysts derived from a Mg2+/A13+/CO3− layered double hydroxide, Catal. Lett., 1994, 23(3–4), 361–367 CrossRef CAS.
- A. Corma, V. Fornes, F. Rey, A. Cervilla, E. Llopis and A. Ribera, Catalytic air oxidation of thiols mediated at a Mo(VI) O2 complex center intercalated in a Zn(II)-Al(III) layered double hydroxide host, J. Catal., 1995, 152, 237–242 CrossRef CAS.
- G. Rathee, N. Singh and R. Chandra, Simultaneous Elimination of Dyes and Antibiotic with a Hydrothermally Generated NiAlTi Layered Double Hydroxide Adsorbent, ACS Omega, 2020, 5(5), 2368–2377 CrossRef CAS PubMed.
- V. Rives and M. A. Ulibarri, Layered double hydroxides (LDH) intercalated with metal coordination compounds and oxometalates, Coord. Chem. Rev., 1999, 181, 61–120 CrossRef CAS.
- X. Xue, Q. Gu, G. Pan, J. Liang, G. Huang, G. Sun, S. Ma and X. Yang, Nanocage structure derived from sulfonated β-cyclodextrin intercalated layered double hydroxides and selective adsorption for phenol compounds, Inorg. Chem., 2014, 53, 1521–1529 CrossRef CAS PubMed.
- A. C. Sutorik and M. G. Kanatzidis, Stabilization of uranyl cations in molten sodium polysulfide and formation of the novel solid oxysulfide Na4(UO2)Cu2S4, J. Am. Chem. Soc., 1997, 119(33), 7901–7902 CrossRef CAS.
- A. Müller, E. Krickemeyer, V. Wittneben, H. Bögge and M. Lemke, (NH4)2[Re2S16], a soluble metal sulfide with interesting electronic properties and unusual reactivity, Angew. Chem., Int. Ed. Engl., 1991, 30, 1512–1514 CrossRef.
- G. Rathee, A. Awasthi, D. Sood, R. Tomar, V. Tomar and R. Chandra, A new biocompatible ternary layered double hydroxide adsorbent for ultrafast removal of anionic organic dyes, Sci. Rep., 2019, 9, 1–14 CrossRef CAS PubMed.
- S. Ma, C. Fan, L. Du, G. Huang, X. Yang, W. Tang, Y. Makita and K. Ooi, Intercalation of macrocyclic crown ether into well-crystallized LDH: formation of staging structure and secondary host–guest reaction, Chem. Mater., 2009, 21, 3602–3610 CrossRef CAS.
- M. J. Manos, N. Ding and M. G. Kanatzidis, Layered metal sulfides: exceptionally selective agents for radioactive strontium removal, Proc. Natl. Acad. Sci. U. S. A., 2008, 105(10), 3696–3699 CrossRef CAS PubMed.
- L. Ma, Q. Wang, S. M. Islam, Y. Liu, S. Ma and M. G. Kanatzidis, Highly selective and efficient removal of heavy metals by layered double hydroxide intercalated with the MoS42− ion, J. Am. Chem. Soc., 2016, 138, 2858–2866 CrossRef CAS PubMed.
- K. Gupta, J. B. Huo, J. C. E. Yang, M. L. Fu, B. Yuan and Z. Chen, (MoS4)2− intercalated CAMoS4·LDH material for the efficient and facile sequestration of antibiotics from aqueous solution, Chem. Eng. J., 2019, 355, 637–649 CrossRef CAS.
- J. Ali, Z. Liao, Z. Zhou, A. Khan, T. Wang, J. Ifthikar, A. Shahzad, Z. Chen and Z. Chen, Fe-MoS4: an effective and stable LDH-based adsorbent for selective removal of heavy metals, ACS Appl. Mater. Interfaces, 2017, 9, 28451–28463 CrossRef PubMed.
- M. H. Beyki, M. Bayat, S. Miri, F. Shemirani and H. Alijani, Synthesis, characterization, and silver adsorption property of magnetic cellulose xanthate from acidic solution: prepared by one step and biogenic approach, Ind. Eng. Chem. Res., 2014, 53, 14904–14912 CrossRef CAS.
- S. Ma, Q. Chen, H. Li, P. Wang, S. M. Islam, Q. Gu, X. Yang and M. G. Kanatzidis, Highly selective and efficient heavy metal capture with polysulfide intercalated layered double hydroxides, J. Mater. Chem., 2014, 2, 10280–10289 RSC.
- X. Liang, W. Hou, Y. Xu, G. Sun, L. Wang, Y. Sun and X. Qin, Sorption of lead ion by layered double hydroxide intercalated with diethylenetriaminepentaacetic acid, Colloids Surf., A, 2010, 366, 50–57 CrossRef CAS.
- L. Zhou, L. Ji, P. C. Ma, Y. Shao, H. Zhang, W. Gao and Y. Li, Development of carbon nanotubes/CoFe2O4 magnetic hybrid material for removal of tetrabromobisphenol A and Pb(II), J. Hazard. Mater., 2014, 265, 104–114 CrossRef CAS PubMed.
- Z. H. Fard, C. D. Malliakas, J. L. Mertz and M. G. Kanatzidis, Direct extraction of Ag+ and Hg2+ from cyanide complexes and mode of binding by the layered K2MgSn2S6 (KMS-2), Chem. Mater., 2015, 27, 1925–1928 CrossRef.
- J. P. Simonin, On the comparison of pseudo-first order and pseudo-second order rate laws in the modeling of adsorption kinetics, Chem. Eng. J., 2016, 300, 254–263 CrossRef CAS.
- A. Jawad, L. Peng, Z. Liao, Z. Zhou, A. Shahzad, J. Ifthikar, M. Zhao, Z. Chen and Z. Chen, Selective removal of heavy metals by hydrotalcites as adsorbents in diverse wastewater: different intercalated anions with different mechanisms, J. Cleaner Prod., 2019, 211, 1112–1126 CrossRef CAS.
|
This journal is © The Royal Society of Chemistry 2020 |
Click here to see how this site uses Cookies. View our privacy policy here.