DOI:
10.1039/D0RA02428G
(Paper)
RSC Adv., 2020,
10, 20098-20109
Hypolipidemic effects of protein hydrolysates from Trachinotus ovatus and identification of peptides implied in bile acid-binding activity using LC-ESI-Q-TOF-MS/MS†
Received
16th March 2020
, Accepted 15th May 2020
First published on 27th May 2020
Abstract
In the present work, analysis of the hypolipidemic properties of Trachinotus ovatus protein hydrolysates (TOPHs) and identification of peptides with bile acid-binding activity were performed. Hydrolysates prepared by trypsin digestion exhibited the highest in vitro bile acid-binding capacities compared with hydrolysates prepared with the other four proteases and were mainly composed of small peptides and amino acids with molecular weights <3 kDa, accounting for 77.30%. Among the five ultra-filtration fractions of TOPHs, TOPHs-5 (<3 kDa) exhibited the highest in vitro bile acid-binding capacity, which was equivalent to 77.97% of cholestyramine at the same concentration. A total of 68 peptides were identified from TOPHs-5 by LC-ESI-Q-TOF-MS/MS and 9 of them had hydrophobicity of more than 60%. These highly hydrophobic peptides might be associated with the bile acid-binding activity of TOPHs-5. In vivo experiments indicated that the TOPHs could effectively reduce total cholesterol (TC), low-density lipoprotein cholesterol (LDL-C) and the atherogenic index (AI), while they could evidently increase the high-density lipoprotein cholesterol (HDL-C) content. Furthermore, TOPHs exerted a marked protective effect on hepatorenal function, as evidenced by decreased levels of aspartate aminotransferase (AST), alanine aminotransferase (ALT) and creatinine (CREA). Histopathological studies confirmed that TOPHs evidently protected the liver from histological alterations. In summary, for the first time, hypolipidemic effects and subsequential identification were obtained from TOPHs, which are promising natural ingredients that could potentially be employed in the management of hyperlipidemia.
1. Introduction
Hyperlipidemia is a common disorder in populations worldwide.1 It is defined as an imbalance in blood lipid homeostasis characterized by an elevation of lipids in the bloodstream, including fats, fatty acids, cholesterol, cholesterol esters, phospholipids, and triglycerides.2 Unhealthy living habits and dietary preferences in parallel with the rapid increase in the number of elderly people accentuate the onset of hyperlipidemia.3,4 Abnormalities in the plasma and serum lipid profiles play a significant role in the etiology of numerous chronic diseases, such as hepatic and renal diseases and metabolic disorders, such as obesity.5 In particular, hyperlipidemia is documented as the major cause of atherosclerosis and atherosclerosis-associated conditions, such as coronary heart disease, ischemic cerebrovascular disease, and peripheral vascular disease.6 In China, the morbidity of cerebrovascular and cardiovascular disease is up to 8%, and the mortality is almost 50%, which makes hyperlipidemia a leading cause of death.7
Currently, the hypolipidemic drugs that are most frequently used for managing hyperlipidemia are bile acid sequestrants, statins, fibrates and nicotinic acids, which exhibit rapid lipid-lowering effects and good efficacy, but the use of these drugs is restricted due to an array of potential side effects, such as liver injury and striated muscle dissolution.8,9 Therefore, the focus of recent research has been on identifying efficient and natural medicines with few side effects for the treatment of hyperlipidemia.10 Recent studies have corroborated that a wide variety of dietary proteins and protein hydrolysates produced by enzymatic hydrolysis from several origins exerted regulatory effects on lipid profiles in human subjects and animal models.11–13
Dietary fish is considered to be beneficial for human health as fish proteins are rich in active peptides.14 Fish protein hydrolysates are converted to smaller peptides containing 2 to 20 amino acids after enzymatic hydrolysis.15 Due to the balanced amino acid composition, the rich nutritional and medicinal value of the active peptides,16 fish protein hydrolysates shown a variety of activities, such as hypolipidemic,17 anti-obesity,18 antioxidative,15 antihypertensive19 and anti-diabetic20 activities, and have drawn considerable research attention.
Trachinotus ovatus, which belongs to Trachinotus sp., Trachinotinae, Carangidae, Perciformes, is a species of temperate water and carnivorous fish that mainly preys on some zooplankton and small crustacean, shellfish and fish.21,22 This fish is widely distributed in Southeast Asia, Australia, Japan, Eastern Africa and the Atlantic ocean.23 It has been considered as a good candidate species for aquaculture and an economically important marine fish due to its fast growth, palatability, the nutritional quality of its flesh, and its suitability for cage culture.24 Artificial breeding of this species has been successfully carried out on the southern coast of China, where it has been a great source of economic revenues in recent years.25 However, there is a knowledge gap about the bioactive substances of T. ovatus. Accordingly, the aim of the present study was to investigate the potential hypolipidemic effects of protein hydrolysates obtained by enzymatic hydrolysis from the muscles of T. ovatus on mice fed a high-fat diet. Moreover, the ultra-filtration fractions of the hydrolysates that possessed the highest in vitro bile acid-binding capacity were further fractionated, purified and subjected to amino acid sequence analysis.
2. Experimental
2.1 Materials
T. ovatus were purchased from Huangsha Aquatic Products Wholesale Market (Guangzhou, China) in the fresh state and authenticated by Prof. Huili Sun (South China Sea Institute of Oceanology, Chinese Academy of Sciences). The samples were packed in polyethylene bags filled with enough water and oxygen, and transported to the laboratory within 1 h. After cleaning the fish with tap water, the muscles were separated and rinsed with cold distilled water to remove the contaminants. The samples were used immediately for protein hydrolysate preparation or stored in sealed plastic bags at −20 °C. The positive control drug cholestyramine was purchased from Nanjing Lifecare Pharmaceutical Co., Ltd (Nanjing, China). Soybean protein peptides (SPPs) were purchased from Heze Zhongshitaihao Biology Produce Co. Ltd (Heze, China). Total cholesterol (TC), triglyceride (TG), low-density lipoprotein cholesterol (LDL-C), high-density lipoprotein cholesterol (HDL-C), aspartate aminotransferase (AST), alanine aminotransferase (ALT), blood urea nitrogen (UREA), and creatinine (CREA) assay kits were obtained from Biosino Bio-Technology and Science Incorporation (Hong Kong, China). The high-fat diet (HFD) consisted of 73.6% normal diet, 10% egg yolk powder, 15% lard, 1.2% cholesterol and 0.2% sodium cholate, and it was supplied by Guangdong Medicinal Laboratory Animal Center (Guangzhou, China). All other chemicals and reagents were of analytical grade.
2.2 Preparation of T. ovatus protein hydrolysates (TOPHs)
The amino acid composition of the fish muscle was determined according to the method described by Cai et al.26 and presented in Table 1. Raw muscles from T. ovatus were homogenized in a blender (Joyoung, JYL-Y910) for approximately 2 min. The homogenate was mixed with 4-fold (g mL−1) of distilled water, incubated at the working temperature for 2 h and then hydrolyzed with five proteases at 2000 U g−1 for 4 h. The pH and temperature of trypsin, papain, bromelain, Alcalase and Neutrase were 8.0, 37 °C; 6.0, 55 °C; 6.0, 55 °C; 8.0, 50 °C; and 7.0, 50 °C, respectively. The reaction was maintained at a constant pH by adding 1 M NaOH or HCl and stopped in a boiling water bath for 10 min to inactivate the enzyme. Then, the hydrolysates were centrifuged at 8000 rpm (500 mL centrifuge tube, Avanti J-26S XP Centrifuge, BECKMAN COULTER, Inc. 250 S. Kraemer Blvd. Brea, CA 92821, USA) for 20 min at 4 °C. One portion of supernatant was collected and concentrated in a vacuum and spray dried (at 105 °C inlet temperature and 85 °C outlet temperature) to obtain TOPHs. The other portion was fractionated by ultra-filtration cassettes (Vivaflow 200: 100k, 10k, 5k and 3k molecular weight cut-off (MWCO) PES, Sartorius Stedim Biotech GmbH Göttingen, Germany) in an ice-water bath. The nonhydrolyzed large molecular proteins and proteolytic enzymes were removed. Five ultra-filtration fractions were obtained and assigned as TOPHs-1 (>100 kDa), TOPHs-2 (>10–100 kDa), TOPHs-3 (5–10 kDa), TOPHs-4 (3–5 kDa) and TOPHs-5 (<3 kDa), respectively.
Table 1 Amino acid composition of T. ovatus proteins (%)a
Amino acid |
Content |
Amino acid |
Content |
*Essential amino acids. #Hydrophobic amino acids. TAA: total amino acids. EAA: essential amino acids. HAA: hydrophobic amino acids. |
Asp |
5.37 |
Tau |
0.56 |
Tyr |
1.89 |
Thr* |
2.47 |
Ser |
2.13 |
Ile*# |
2.50 |
Glu |
7.82 |
Leu*# |
4.26 |
Pro# |
1.92 |
Val*# |
2.74 |
Gly# |
3.46 |
Phe*# |
2.21 |
Ala# |
3.56 |
Lys* |
4.97 |
His |
1.44 |
Met*# |
1.65 |
Arg |
3.40 |
Trp*# |
0.51 |
TAA |
52.86 |
HAA/TAA |
43.15 |
EAA |
21.30 |
Met/Gly |
47.69 |
HAA |
22.81 |
Lys/Arg |
146.18 |
2.3 Determination of molecular weight distribution
Molecular weight (MW) distributions of TOPHs were determined by gel permeation chromatography (GPC) using an LC-20AT HPLC system (Shimadzu, Kyoto, Japan) equipped with a TSK-GEL G2000SWXL column (7.8 mm × 300 mm, Tosoh, Tokyo, Japan), and the mobile phase was composed of acetonitrile/water/trifluoroacetic acid (TFA) (20/80/0.1, v/v/v). The samples (10 μL) that had been filtered by a 0.22 μm microfiltration membrane were loaded. Samples were eluted at a flow rate of 0.5 mL min−1 and detected at 220 nm. Cytochrome C (12
400 Da), aprotinin (6511 Da), bacitracin (1450 Da), oxidized glutathione (307 Da), and phenylalanine (165 Da) were selected as standard samples to establish a calibration curve for the relation between retention time and log
Mw. All data were collected and analyzed by GPC software (Version 1.26 SP1, Shimadzu, Japan). The calibration curve obtained from duplicate runs was described as follows:
log MW = 0.002t3 − 0.14t2 + 2.8567t − 14.014 (R2 = 0.9963) |
where t was the elution time in min; MW was the molecular weight.
2.4 Bile acid-binding capacity assay
The in vitro bile-acid binding capacity was detected according to the method of T. S. Kahlon et al.27 with some modifications. The test samples (1 mL; 100 mg mL−1) and the positive control cholestyramine (1 mL; 20 mg mL−1) were added to the 25 mL stoppered test tubes, respectively, and 1 mL of HCl (0.01 mol L−1) was added to digest the samples for 1 h in a 37 °C shaker bath (simulating the stomach environment). After acid incubation to simulate gastric digestion, the sample pH was adjusted to 6.3 with 0.1 M NaOH. Each sample was added to 4 mL of choline salt solution (0.2 mmol L−1), including sodium cholate (SC), sodium glycocholate (SGC), and sodium taurocholate (STC), and 4 mL PBS was added to the blank. Then, 5 mL of porcine pancreatin (4 × USP, 10 mg mL−1 for simulating the intestinal environment) was added, and the reaction mixture was shaken at 37 °C for 1 h. In the bile salt blank, only 4 mL of the cholate standard solution was added, and the remaining steps were replaced with phosphate buffer. The thermostat-vibrated samples were transferred to a centrifuge tube and were centrifuged at 10
000 rpm for 15 min. Then, 2.5 mL of the supernatant was transferred into a 25 mL stoppered tube, and 7.5 mL of 60% sulfuric acid solution was added. After incubation at 70 °C for 50 min, the samples were frozen at −20 °C for 10 min. Triplicate analysis of each sample was performed, and values were determined from a cholate standard curve at a concentration of 0.02, 0.04, 0.06, 0.08, 1.0 mmol L−1. The bile acid-binding capacity was calculated according to the following equation:
where C0 was the concentration of bile salt blank and C1 was the concentration of cholate in the supernatant.
2.5 Purification of TOPHs-5
Based on the bile acid-binding capacity, TOPHs-5 with the highest activity was redissolved in water at a concentration of 100 mg mL−1 and loaded onto a Sephadex G-15 column (3.0 × 45 cm). The fraction was eluted with distilled water at a flow rate of 10 mL min−1 and monitored at 220 and 280 nm using automatic chromatography (EZ Purifier III, Shanghai Lishui Chemical Engineering Co., Ltd, Shanghai, China). Four fractions (Fr. 1–Fr. 4) were obtained, and two (Fr. 1 and Fr. 2) of which had a high yield. They were further purified through reversed phase high performance liquid chromatography (RP-HPLC) with Agilent 1260 HPLC (USA) on a YMC-Pack ODS-A column (250 × 4.6 mm I.D. S-5 μm, 12 nm) at 40 °C. The mobile phase was 0.1% TFA in water (solvent A) and 0.1% TFA in acetonitrile (solvent B). Gradient elution was performed according to the following process for each fraction: Fr. 1, 0–5 min, 6% B; 5–45 min, 6–25% B; 45–47 min, 25–35% B; 47–50 min, 35–75% B; 50–52 min, 75–90% B; 52–54 min, 90–6% B; Fr. 2, 0–5 min, 6% B; 5–45 min, 6–25% B; 45–50 min, 25–40% B; 50–52 min, 40–75% B; 52–54 min, 75–90% B; 54–55 min, 90–6% B, at a flow rate of 1.0 mL min−1. The eluted peptides were monitored by absorbance at 220 nm.
2.6 Identification of peptides by LC-ESI-Q-TOF-MS/MS
The fractions derived from RP-HPLC purification were subjected to LC-ESI-Q-TOF-MS/MS analysis in order to identify all the potential peptides. The analysis was conducted on a HPLC system (Agilent 1260, USA), connected to a Bruker Q-TOF Premier mass spectrometer (Bruker Da; TPMOCS Inc., Billerica, MA) equipped with an electrospray ion source. An aliquot of 5 μL of the fractions were separated on a YMC-Pack ODS-AQ column (250 × 4.6 mm, 5 μm) at 40 °C. The mobile phase used for elution consisted of solvent A (0.1% formic acid in water, v/v) and solvent B (0.1% formic acid in acetonitrile, v/v). The gradient elution procedure was the same as mentioned above. Detection by ESI-Q-TOF was carried out in the positive ion mode at a capillary voltage of 4500 V and a m/z range from 200 to 2000. Other MS conditions were applied as follows: nebulizer gas, nitrogen, 0.8 bar; dry gas, nitrogen, 5.0 L min−1, 180 °C; endplate offset, 500 V. The quadrupole ion energy and collision induced dissociation energy were set at 5.0 and 10.0 eV, respectively. The bioinformatics search engine Mascot Distiller v 2.6.0 software (Matrix Science, Boston, MA), UniProtKB/Swiss-Prot database and NCBInr protein database were used for analyzing the acquired MS/MS data, and peptides identified above an appropriate −10
log
P threshold were considered as a true positive.
2.7 In vivo experiment
2.7.1 Animals and treatment. Male Kunming (KM) mice (supplied by Guangdong Medical Laboratory Animal Center, Foshan, China, certification no. SCXK 2013-0002) weighing 18–22 g, were housed in a storage room. All the mice were kept in stainless steel cages with a constant temperature of 23 ± 1 °C, a relative humidity of 50 ± 5% and a 12 h light/dark cycle. The mice were given a standard diet and allowed free access to tap water. All animal procedures were performed in accordance with the U.S. National Institutes of Health Guide for the Care and Use of Laboratory Animals (publication no. 85-23, revised 1996) and experiments were approved by the Animal Ethics Committee of Sun Yat-sen University.After one week of accommodation, sixty KM mice were randomly divided into ten groups (six mice per group) and gavaged once a day for 8 weeks.
CD group: mice were fed daily with a standard diet and gavaged with deionized water at a dose of 1 mL daily for 8 weeks (control diet group).
HFD group: mice were fed daily with the high-fat diet (HFD) and gavaged daily with 1 mL of deionized water.
CD + SPPs group: mice were fed daily with a standard diet and treated with SPPs by gastric gavage daily at a dose of 2 g kg−1 body weight.
CD + TOPHs-H group: mice were fed daily with a standard diet and treated with TOPHs by gastric gavage daily at a dose of 5 g kg−1 body weight.
CD + CHO group: mice were fed daily with a standard diet and administered cholestyramine (CHO) at a daily dose of 0.2 g kg−1 body weight.
HFD + SPPs group: mice were fed daily with the high-fat diet and treated with SPPs at a daily dose of 2 g kg−1 body weight.
HFD + TOPHs-L, HFD + TOPHs-M and HFD + TOPHs-H groups: mice were fed daily with the high-fat diet and treated daily with TOPHs by gastric gavage at a dose of 0.5 g kg−1, 2.5 g kg−1 and 5.0 g kg−1 body weight, respectively.
HFD + CHO group: mice were fed daily with the high-fat diet and treated with cholestyramine at a daily dose of 0.2 g kg−1 body weight.
2.7.2 Blood and tissue sample collection. At the end of the feeding period, the mice were deeply anesthetized with ether and sacrificed by cervical decapitation to avoid stress after a 12 h overnight fast. A blood sample was collected, then centrifuged for 15 min at 4000 rpm for serum separation, and frozen in liquid nitrogen at −80 °C prior to the determination of the serum lipid profile. Livers, kidneys and spleens were excised from the experimental mice, rinsed with cold phosphate-buffered saline, and weighed for measuring and calculating the organ index, which was defined as organ weight/body weight.
2.7.3 Biochemical parameters assay. The blood urea nitrogen (BUN), creatinine (CREA), alanine aminotransferase (ALT), aspartate aminotransferase (AST), total cholesterol (TC), triglyceride (TG), low-density lipoprotein cholesterol (LDL-C), and high-density lipoprotein cholesterol (HDL-C) concentrations in serum were assayed enzymatically using commercial kits according to the manufacturer's instructions (Biosino Bio-Technology and Science, Inc.), and the atherogenic index (AI) was calculated as (TC-HDL-C)/HDL-C.28
2.7.4 Histopathological analysis. Once the livers and kidneys were obtained, they were immediately fixed in cold 4% paraformaldehyde in 0.01 M phosphate-buffered saline (PBS, pH 7.4) for 48 h. Then, they were subjected to a standard routine tissue processing technique and embedded in paraffin. The paraffin sections of the organs were cut to a thickness of 5 μm and adhered to coated glass. After the sections were dewaxed in xylene and rehydrated through a decreasing series of ethanol, they were stained with Harris's hematoxylin and eosin according to standard procedures and were examined under an Olympus light microscope (CX31; Olympus, Japan).
2.8 Statistical analysis
The data were analyzed using SPSS 24.0 software (BM INC., New York, USA). All values were expressed as the mean ± standard deviation. One-way ANOVA with Tukey's post hoc test was used to compare significant differences. Differences between the two groups were analyzed by the Student's t-test. The results were considered to be statistically significant if p < 0.05.
3. Results and discussion
3.1 Amino acid compositions
Several studies have indicated that the specific amino acid compositions of dietary proteins were most likely involved in the hypolipidemic effects of these proteins.29 The amino acid composition of the undigested T. ovatus protein is shown in Table 1. The fish protein was rich in amino acids and had a high percentage of essential amino acids (Val, Met, Lys, Ile, Leu, Phe, Trp and Thr), which was similar to the FAO/WHO reference protein. Studies have suggested that dietary proteins with low ratios of Met/Gly and Lys/Arg may contribute to the cholesterol-lowering effect of dietary protein.30,31 Many studies have also shown that fish protein hydrolysates that contain lower ratios of Met/Gly and Lys/Arg compared to casein have cholesterol-lowering effects.32,33 In addition, hydrophobic amino acids, which facilitate protein hydrolysates immersion in lipid micelles, play an important role in cholesterol-lowering activity, especially affecting bile acid-binding ability. This effect could be attributed to the interaction of the amino acid residues with the hydrophobic core and the anionic carboxylic acid group of the bile acids, which are amphipathic to bind other hydrophobic amino acids by weak hydrophobic forces, forming an insoluble complex for fecal removal.34,35 In this study, the ratio of Met/Gly, Lys/Arg and hydrophobic amino acids in fish protein was similar to that reported in many previous studies,36,37 suggesting that T. ovatus protein and its hydrolysates have potential lipid-lowering effects.
3.2 Preparation of T. ovatus protein hydrolysates (TOPHs)
Since the protein hydrolysates and peptides obtained by protease hydrolysis have better bioactivity compared to their parent proteins, the choice of enzymes, processing conditions, the specificity of the proteases, and the degree of hydrolysis often affect the bioactive properties of the protein hydrolysates.38 In the present study, trypsin, papain, bromelain, Alcalase and Neutrase were used for preparation of protein hydrolysates from T. ovatus muscle proteins. The bile acid-binding capacity was used as an assessment index to select the optimum enzyme and the hydrolysis conditions. The results comparing the bile acid-binding capacities of protein hydrolysates processed by five proteases are shown in Fig. 1A. The in vitro bile acid-binding capacity of the protein hydrolysates produced by trypsin was better than that of other proteases, and the binding rate of sodium cholate (SC), sodium glycocholate (SGC) and sodium taurocholate (STC) reached 42.07%, 33.55% and 30.07%, respectively. There are two kinds of bile in healthy humans, conjugated bile acid and free bile acid, and the former is dominant. Both SGC and STC belong to conjugated cholate. It has been reported that STC was a conjugated cholate that was difficult combine, and substances with strong STC binding capacity tended to have a stronger SGC binding capacity in the human body.39 Therefore, the trypsin hydrolysis approach was used to prepare protein hydrolysates, and the in vitro SGC binding rate was used as an activity indicator.
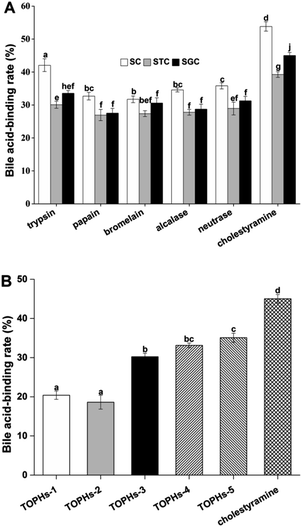 |
| Fig. 1 In vitro bile acid-binding capacity of different samples and cholestyramine was served as a positive control. (A) The in vitro binding capacities of bile acids of five hydrolysates. SC, SGC and STC represented sodium cholate, sodium glycocholate and sodium taurocholate, respectively. The concentration of the five hydrolysates was 100 mg mL−1, and the positive control group was 20 mg mL−1. (B) The in vitro SGC binding capacity of the ultra-filtration fractions and the positive control. The concentrations of the ultra-filtration fractions and the positive control group were 20 mg mL−1. The different letters on the histogram mean that there was a significant difference compared with the others, p < 0.05. | |
3.3 Molecular weight distribution of TOPHs and their ultra-filtrates
As presented in Table 2, the molecular weight of the TOPHs were mainly distributed in <1 kDa (50.68%, as illustrated in the Table 2 footnote), indicating a large proportion of low molecular weight peptides in TOPHs. The molecular weight of the hydrolysates was mostly attributable to small peptides with a molecular mass lower than 3 kDa, accounting for 77.30% of TOPHs. Meanwhile, the molecular weight distribution of the ultra-filtration fractions was consistent with that of the TOPHs, and the fraction of molecular weight <3 kDa in TOPHS-5 reached 98.07%. Related studies have pointed out that peptides with a molecular weight below 3 kDa contained a variety of biological activities, such as lowering cholesterol, antioxidation, lowering blood pressure, and immunoregulation,40 and they have broad application prospects in functional foods. This result was validated in the following bile acid binding experiments.
Table 2 Molecular weight distribution of TOPHs and their five ultra-filtratesa
|
Area percentages (%) |
>10 kDa |
5–10 kDa |
3–5 kDa |
<3 kDa |
TOPHs are the protein hydrolysates produced by trypsin; TOPHs-1, TOPHs-2, TOPHs-3, TOPHs-4 and TOPHs-5 are ultra-filtration fractions of TOPHs, that were produced sequentially by ultra-filtration with 100 kDa, 10 kDa, 5 kDa and 3 kDa MWCO membranes. The molecular weight distributions of 2–3 kDa, 1–2 kDa, and <1 kDa fractions of the TOPHs account for 8.92%, 17.70% and 50.68%, respectively. |
TOPHs |
4.11 |
8.77 |
9.82 |
77.30 |
TOPHs-1 |
5.85 |
9.78 |
10.26 |
74.11 |
TOPHs-2 |
5.61 |
13.29 |
12.04 |
69.06 |
TOPHs-3 |
0.41 |
6.60 |
10.76 |
82.23 |
TOPHs-4 |
0 |
0.41 |
2.72 |
96.87 |
TOPHs-5 |
0 |
0.25 |
1.68 |
98.07 |
3.4 Bile acid-binding capacity of the ultra-filtration fractions
The bile acid-binding capacities of the five ultra-filtration fractions are shown in Fig. 1B. Among the five fractions, TOPHs-3, TOPHs-4 and TOPHs-5 exhibited strong bile acid-binding capacity, up to 30.22%, 33.13%, and 35.09%, which were equivalent to 67.10%, 73.56% and 77.97% of cholestyramine at the same concentration. There was no significant difference between TOPHs-5 and TOPHs-4 (p > 0.05), but there were significant differences between TOPHs-5 and other fractions as well as the positive control cholestyramine (p < 0.05).
According to the aforementioned study, the remarkable bile acid-binding capacity of the ultra-filtration fractions may be attributed to the amino acid composition and the ratio of hydrophobic amino acids. In TOPHs-5, the components less than 3 kDa accounted for 98.07% (Table 2), suggesting that the main substance that bound to bile acid was peptides of molecular weight not more than 3 kDa. Some previous study had demonstrated that the smaller the molecular weight, the more significant binding capacity to bile acid.41 These were pivotal fractions with hypolipidemic potential and application prospects.
3.5 Purification and identification of peptides derived from TOPHs-5
Initially, TOPHs-5 was separated into four fractions (Fr. 1–Fr. 4) on a Sephadex G-15 chromatography column (Fig. 2A). Among the separated fractions, Fr. 3 and Fr. 4 had a low yield. Therefore, Fr. 1 and Fr. 2 were further purified with reverse phase HPLC on an analytical RP-column (ODS-AQ) and identified by LC-ESI-Q-TOF-MS/MS. As showed in Table S1 of ESI,† a total of 62 peptide sequences with relative molecular mass values between 527 and 2557 Da and between 7 and 24 amino acids in length were identified in Fr. 1, while a total of 7 peptide sequences with relative molecular mass values between 517 and 1762 Da and between 7 and 14 amino acids in length were identified in Fr. 2. The total ion current chromatograms of Fr. 1 and Fr. 2 were shown in Fig. 2B and C, in which the numbers on the chromatogram represented the identified peptides corresponding to the retention time. In fact, some studies have reported that high proportion of hydrophobic amino acid contributed significantly to the hypolipidemic effect of peptides. For instance, Lin et al. found that the hypocholesterolemic peptides comprised Val and Pro, and exerted a correlation between the hydrophobicity and the capacity to bind to bile acids.42 Zhong et al. found that the higher the content of hydrophobic amino acids in the fractions of soy protein hydrolysate, the stronger the inhibition of cholesterol micelle solubility in vitro, demonstrating a remarkable hypocholesterolemic effect.43 In the present study, there were eight peptides with hydrophobicity more than 60% in Fr. 1 and their sequences were identified as ANALAANLDK, ITALAPSTMK, EITALAPSTM, LNFDAFLPMLK, AAADGPMK, YVDIVVLK, LGVAAGA and APALEGA, respectively. There were two peptides with hydrophobicity more than 70% in Fr. 2 and their sequences were LNFDAFLPMLK and SFPLAEFL, respectively. Since LNFDAFLPMLK was the same peptide identified simultaneously in Fr. 1 and Fr. 2, a total of 9 peptides with hydrophobicity greater than 60% were identified from TOPHs-5 (Fig. 3). They were mainly composed of hydrophobic amino acid residues such as Pro, Ala and Leu, which could also be responsible for the bile acid-binding effect of TOPHs-5.
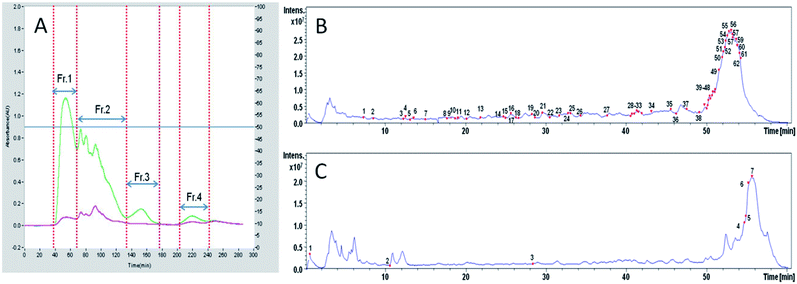 |
| Fig. 2 Purification of TOPHs-5 by gel filtration chromatography and RP-HPLC. (A) Sephadex G-15 gel filtration chromatography of TOPHs-5. Four fraction termed as Fr. 1, Fr. 2, Fr. 3 and Fr. 4 were obtained, and high yield fraction (Fr. 1 and Fr. 2) were further purified by RP-HPLC and identified using LC-ESI-Q-TOF-MS/MS system. (B) Total ion current (TIC) chromatogram of Fr. 1. (C) Total ion current (TIC) chromatogram of Fr. 2. The numbers on the chromatogram represented peptide sequences identified by LC-ESI-Q-TOF-MS/MS from Fr. 1 and Fr. 2, respectively (details are presented in Table S1 of the ESI†). RP-HPLC purification was performed with Agilent 1260 HPLC (USA) on a YMC-Pack ODS-A column (250 × 4.6 mm I.D. S-5 μm, 12 nm) at 40 °C. The mobile phase was 0.1% TFA in water (solvent A) and 0.1% TFA in acetonitrile (solvent B). The eluted peptides were monitored by absorbance at 220 nm. | |
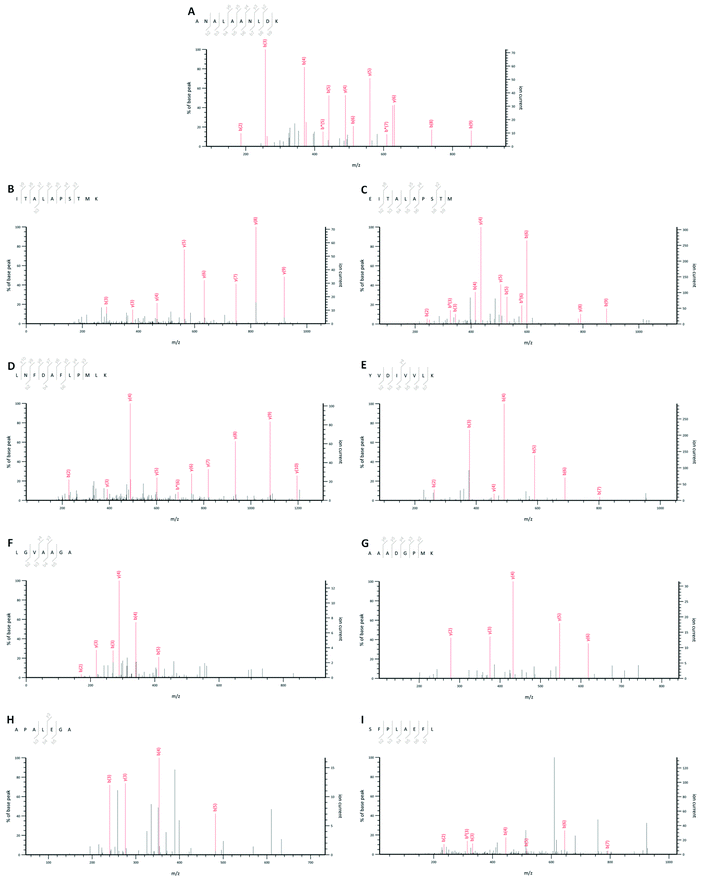 |
| Fig. 3 The peptides with hydrophobicity >60% identified in TOPHs-5. The identification was carried out using an Agilent 1260 HPLC system, connected to a Bruker Q-TOF Premier mass spectrometer equipped with an electrospray ion source. An aliquot of 5 μL of the fractions were separated on a YMC-Pack ODS-AQ column (250 × 4.6 mm, 5 μm), and then were subjected to mass spectrometry analysis. Mass spectrometry was performed in the positive ions electrospray scan mode (ESI+) at a capillary voltage of 4500 V and a m/z range from 200 to 2000. (A) LC-MS/MS spectrum of ANALAANLDK. (B) LC-MS/MS spectrum of ITALAPSTMK. (C) LC-MS/MS spectrum of EITALAPSTM. (D) LC-MS/MS spectrum of LNFDAFLPMLK. (E) LC-MS/MS spectrum of YVDIVVLK. (F) LC-MS/MS spectrum of LGVAAGA. (G) LC-MS/MS spectrum of AAADGPMK. (H) LC-MS/MS spectrum of APALEGA. (I) LC-MS/MS spectrum of SFPLAEFL. | |
3.6 Effects of TOPHs on body weight and organ indices in mice
Currently, dietary protein sources with the function of hypolipidemic effect mainly composed of soybean protein, milk protein, egg white protein, buckwheat protein and fish protein. However, most papers on hypolipidemic effect focused on soybean protein hydrolysates and peptides, and a series of studies covered various types of soybean protein, which made soybean protein and its peptides an ideal reference model for the hypolipidemic research.44 The growth parameters of the different groups of mice during the eight weeks are presented in Fig. S1 of ESI.† At the end of the experiment, body weight and organ indices of the mice in all the groups had an obvious increase. However, no significant differences were observed between the treatment groups, except for the decrease of liver index and the increase of spleen index in the SPPs combined with standard diet group caused by individual differences, indicating that the administration of TOPHs had no toxic effect at the experimental dose. The body weight change could be attributed to the high-fat diet formula, which contained a high proportion of cholesterol and cholate that affected the taste and showed an appetite-suppressing effect on mice. Therefore, the improvement of blood lipid profiles was more pronounced than the measurable difference in body weight. These results were consistent with those of Nasri et al.45
3.7 Effect of TOPHs on serum lipids in mice
The effects of TOPHs on the serum lipid profiles in experimental mice are shown in Table 3. A significant increase was observed in serum total cholesterol (TC), low-density lipoprotein cholesterol (LDL-C) levels and atherogenic index (AI) value by 112.20%, 77.92%, 621.05%, respectively, and there was a 33.53% decrease in the serum high-density lipoprotein cholesterol (HDL-C) levels in the HFD group compared to those of the CD group (p < 0.05). However, there were no significant differences in the serum TG content between the HFD group and the CD group (p > 0.05), which could be a typical hypercholesterolemia. In addition, mice fed with a standard diet and simultaneously treated with a 2 g kg−1 d−1 dose of SPPs, a 5 g kg−1 d−1 dose of TOPHs or a 0.2 g kg−1 d−1 dose of cholestyramine (CD + SPPs, CD + TOPHs-H and CD + CHO groups) did not have significant different serum lipid levels compared with those of the CD group, but there was a significant difference compared with those of the HFD group. The overall results indicated that the hyperlipidemic model was successfully established. Moreover, SPPs, TOPHs and cholestyramine have no obvious adverse effect on the serum lipids of normal mice.
Table 3 Serum TC, TG, HDL-C, LDL-C and AI in different KM mice groupsa
Groups |
TC (mmol L−1) |
TG (mmol L−1) |
HDL-C (mmol L−1) |
LDL-C (mmol L−1) |
AI |
Values are expressed as the mean ± SD of six mice in each group. aRepresents p < 0.05 compared to the CD groups. bRepresents p < 0.05 compared to the HFD group. cRepresents p < 0.05 compared to the HFD + SPPs group. dRepresents p < 0.05 compared to the HFD + TOPHs-L group. eRepresents p < 0.05 compared to the HFD + TOPHs-M group. No significant differences were observed between HFD + TOPHs-H and HFD + CHO groups (p > 0.05), for which no superscript letters are marked. |
CD |
2.54 ± 0.50 |
1.04 ± 0.11 |
1.67 ± 0.27 |
1.54 ± 0.19 |
0.57 ± 0.42 |
HFD |
5.39 ± 0.86a |
0.98 ± 0.08 |
1.11 ± 0.19a |
2.74 ± 0.56a |
4.11 ± 1.79a |
CD + SPPs |
2.68 ± 0.31b |
1.05 ± 0.10 |
1.16 ± 0.08b |
1.56 ± 0.22b |
0.84 ± 0.27b |
CD + TOPHs-H |
2.74 ± 0.23b |
1.45 ± 0.50ab |
1.30 ± 0.15a |
1.58± 0.12b |
1.11 ± 0.19b |
CD + CHO |
2.48 ± 0.27b |
1.36 ± 0.16ab |
1.95 ± 0.55b |
1.83 ± 0.28b |
0.36 ± 0.41b |
HFD + SPPs |
4.81 ± 0.30ab |
0.84 ± 0.08 |
1.55 ± 0.25b |
2.51 ± 0.06a |
2.18 ± 0.58ab |
HFD + TOPHs-L |
4.39 ± 0.39ab |
0.91± 0.21 |
1.46 ± 0.30b |
2.41 ± 0.26ab |
2.11 ± 68ab |
HFD + TOPHs-M |
4.28 ± 0.47ab |
0.86 ± 0.07 |
1.51 ± 0.04b |
2.11± 0.14abc |
1.83 ± 0.31ab |
HFD + TOPHs-H |
3.97 ± 0.19abc |
0.76 ± 0.11a |
1.67 ± 0.11b |
1.97 ± 0.17abcd |
1.38 ± 0.20ab |
HFD + CHO |
3.47 ± 0.51abcde |
0.70 ± 0.13ab |
1.56 ± 0.29b |
1.76 ± 0.25bcde |
1.28 ± 0.47bcd |
After 8 weeks gavage administration, the contol sample of SPPs, the different doses of TOPHs and the positive control drug of cholestyramine all significantly decrease the serum TC and LDL-C levels (excluding SPPs treatment group) and AI value of the hyperlipidemic mice (p < 0.05). Meanwhile, the treatments also led to a marked increase in serum HDL-C levels (p < 0.05). Changes in the TOPHs treatment groups revealed a dose-dependent manner. In comparison to HFD group, the TC, TG, LDL-C levels and AI value of the HFD + TOPHs-H group decreased by 26.28%, 22.53%, 28.20% and 66.37%, respectively, and the HDL-C increased by 51.43%, which was higher than that in the positive drug group (HFD + CHO group, 40.72%). However, there were no significant difference in serum lipid profiles and AI value between the HFD + TOPHs-M, HFD + TOPHs-H and the positive drug groups (p > 0.05). Compared with the HFD + SPPs group, the HFD + TOPHs-M and HFD + TOPHs-H groups had stronger effects on reducing TC and LDL-C levels. Although there was no statistical difference in reducing AI index, it was obvious that the TOPHs-H and TOPHs-M treated group had a better effect (66.37% and 55.52% compared with 48.72%). In general, the HFD + TOPHs-L group and the HFD + SPPs group had roughly similar effects in improving lipid profiles and AI index. The results showed that the improvement effect on the blood lipids and AI values in the middle-dose group and the high-dose group of TOPHs was similar to that of cholestyramine, but the effect was better than that of SPPs.
Previous studies have corroborated that high-fat feeding caused an elevation in serum TC, TG, LDL-C, and AI, and caused a reduction in HDL-C in laboratory animals. It led to abnormal lipid metabolism and consequent hyperlipidemia, which were simultaneously associated with increased risk of cardiovascular disease.46–49 Excessive cholesterol, particularly LDL-C, with elevated deposition in blood vessel walls leads to atherosclerosis. However, HDL-C facilitates cholesterol transport from peripheral tissues to the liver for catabolism. Decreasing the serum LDL-C and increasing HDL-C can lower the risk of developing cardiovascular disease.50,51 On the basis of the current literature, the hypolipidemic effects of dietary food proteins could be associated with the amino acid composition and sequence of the bioactive peptides, which are released by enzymatic hydrolysis.38
Numerous studies have suggested that the possible mechanisms of the hypolipidemic effects of dietary peptides and proteins include the following major aspects. Firstly, dietary peptides and proteins can disrupt the cholesterol micellar solubility. Secondly, they can bind bile acids by hydrophobic or ionic forces to form insoluble adducts. Thirdly, they can alter hepatic and adipocytic enzyme activities and gene expression associated with cholesterol metabolism.52,53 In the present study, administration of fish protein hydrolysates generated by trypsin obviously improved lipid metabolism and lowered the incidence of hyperlipoidemia in mice, but the precise hypolipidemic mechanisms of protein hydrolysates were not clear. However, several hypolipidemic mechanisms of fish protein hydrolysates have been reported in the literature, and several hypotheses may be proposed to explain the potential mechanisms of our study. One explanation could be that TOPHs inhibited cholesterol biosynthesis through a decrease in 3-hydroxyl-3-methyl-glutaryl-coenzyme A (HMG-CoA) reductase activity and an increase in the low density lipoprotein receptor (LDL-R).11,30 Another explanation could be that TOPHs enhanced the metabolism and decomposition of cholesterols and inhibited the enterohepatic cycle of bile acids. It mainly acted by upregulating the expression of liver X receptor (LXR) α and cholesterol 7α-hydroxylase (CYP7α-1) genes and by downregulating the expression of the hepatic farnesoid X receptor (FXR).54 Furthermore, TOPHs could effectively inhibit the absorption of cholesterol in the jejunum and bile acid in the ileum, which could attributed to their strong bile acid-binding capacity.55 These possible mechanisms are supported by several previous studies on hypolipidemic effects of fish protein hydrolysates.56–58 Further studies for the underlying mechanism of TOPHs on the hypolipidemic effect are warranted.
3.8 Effect of TOPHs on hepatorenal function of mice
ALT and AST are the main indicators of liver function, and BUN and CREA are the main indicators of kidney function. The increase of the four indicators mentioned above reflects the extent of liver damage and renal dysfunction, suggesting hepatocyte leakage and loss of functional integrity of the cell membrane and changes in glomerular structure and function.59 Changes in biochemical parameters in all the experimental mouse groups are shown in Table 4. Compared with the normal control group, the HFD group gave rise to a significant increase in ALT and AST (p < 0.05), demonstrating that the high-fat diet led to dysfunction of the liver. However, oral administration of TOPHs and cholestyramine decreased the levels of the two enzymes in the serum, and TOPHs acted in a dose-dependent manner. Moreover, the serum ALT and AST values of the HFD + TOPHs-H group declined by 49.35% and 32.28%, respectively, higher than those in the HFD + CHO group (36.02% and 24.45%, respectively). It was interesting to note that there were no significant changes in the BUN level of the treatment group (p > 0.05), although the CREA level in the TOPHs groups decreased in a dose-dependent manner compared with that in the HFD group (p < 0.05). The improvement effect of SPPs treatment group on liver and kidney function was similar to that of TOPHs-M treatment group. We speculated that the administration of TOPHs can significantly correct the disturbances of the hepatorenal function with no fat deposition in the liver, resulting in a protective effect on renal histological changes.60 This hypothesis would be validated in subsequent histopathological observations. Thus, the present study indicated that TOPHs treatment could effectively improve liver and kidney function and prevent the organ damages induced by high-fat diet.
Table 4 Serum ALT, AST, BUN and CREA in different KM mice groupsa
Groups |
ALT (U L−1) |
AST (U L−1) |
BUN (mmol L−1) |
CREA (μmol L−1) |
Values are expressed as the mean ± SD of six mice in each group. aRepresents p < 0.05 compared with the CD group. bRepresents p < 0.05 compared with the HFD group. cRepresents p < 0.05 compared with the HFD + SPPs group. dRepresents p < 0.05 compared with the HFD + TOPHs-L group. eRepresents p < 0.05 compared with the HFD + TOPHs-M group. Except for the difference in ALT between the HFD + TOPHs-M and the HFD + TOPHs-H groups, there was no significant difference between the HFD + TOPHs-M, HFD + TOPHs-H and HFD + CHO groups (p > 0.05). |
CD |
19.78 ± 1.05 |
91.81 ± 3.46 |
5.21 ± 0.30 |
163.07 ± 8.39 |
HFD |
30.88 ± 9.38a |
110.93 ± 22.22a |
5.63 ± 0.21 |
160.46 ± 10.02 |
CD + SPPs |
20.62 ± 3.17b |
94.13 ± 6.71b |
5.53 ± 0.36 |
160.87 ± 14.44 |
CD + TOPHs-H |
18.02 ± 1.81b |
87.82 ± 6.08b |
5.77 ± 0.55a |
166.65 ± 13.48 |
CD + CHO |
12.47 ± 2.66b |
77.88 ±4.82ab |
5.14± 0.24 |
161.19 ± 7.69 |
HFD + SPPs |
26.85 ± 6.94 |
90.72 ± 6.61b |
5.83 ± 0.51a |
186.09 ± 8.54ab |
HFD + TOPHs-L |
29.75± 10.81a |
98.91 ±11.47 |
6.18 ±0.69ab |
150.18 ± 2.31ac |
HFD + TOPHs-M |
25.01 ± 8.30 |
88.71 ± 19.04b |
5.92 ± 0.67a |
148.88 ± 5.37abc |
HFD + TOPHs-H |
15.64 ± 6.01bcde |
75.12 ± 11.17abcd |
5.83 ± 0.09a |
146.55 ± 5.56abc |
HFD + CHO |
19.76 ± 4.55bd |
83.80 ± 10.21bd |
5.41 ± 0.47d |
148.99 ± 8.29abc |
3.9 Effect of TOPHs on hepatic and renal histopathological changes
The results of histological examination of mouse liver and kidney are shown in Fig. 4(a) and (b), respectively. As shown in Fig. 4(a-A), the liver of the CD group showed a normal hepatic lobule architecture and normal hepatocytes structure. In contrast, the arrangement of the hepatic cords of mice in the HFD group was disordered or disappeared in the light microscope, and a large number of hepatocytes were swollen and had undergone hydropic degeneration. Moreover, hepatocytes showed typical steatosis and cytoplasmic vacuoles, and diffuse steatosis was observed in the liver cells of some samples. A small number of lymphocytes and macrophages were observed in the portal areas, where pseudo lobules along with spotty and focal necrosis of hepatocytes were also observed (Fig. 4(a-B)). As shown in Fig. 4(a-F), the hepatocytes of HFD mice treated with SPPs showed focal mild to moderate steatosis, which was concentrated around the portal area, and a few hepatocytes were edematous. The effects of TOPHs on hepatocyte cells of HFD mice are presented in Fig. 4(a-(G–I)). With increasing intervention doses, the fat droplets and fat vacuoles decreased and became smaller until they gradually disappeared, the hepatocyte edema and degeneration was gradually ameliorated, and the structure of hepatocytes tended to become normal. Healthy mice, which fed SPPS, TOPHs-H and cholestyramine, exhibited a normal liver histological appearance (Fig. 4(a-(C–E))). Interestingly, hepatocytes from HFD mice treated with cholestyramine revealed improvement with mild steatosis, edema, and fewer fat vacuoles, and an essentially normal liver cell structure (Fig. 4(a-J)). The kidneys of healthy control mice showed normal histologies with clear renal cortex and medulla structures (Fig. 4(b-A)). No significant histological changes were observed in the kidneys of the HFD, SPPs, TOPHs, or cholestyramine treated groups (Fig. 4(b-(B–J))). This result was consistent with the biochemical parameters determination of the aforementioned serum BUN and CREA, which proved our previous hypothesis.
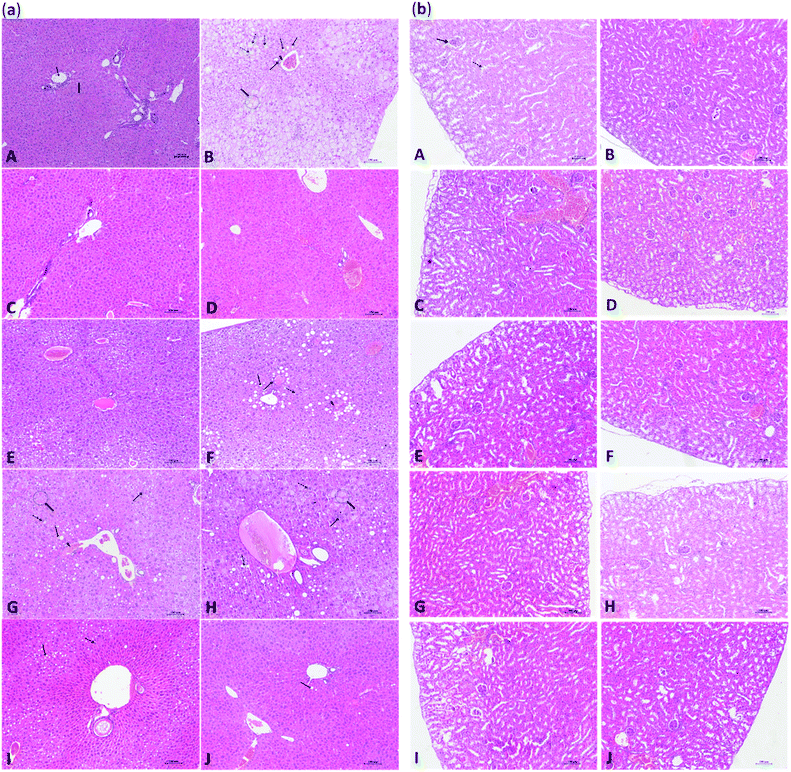 |
| Fig. 4 Effect of TOPHs on the histopathological changes in mouse liver and kidney tissue. (a) Liver and (b) kidney sections were stained with hematoxylin and eosin. (A) CD group. (B) HFD group. (C) CD + SPPs group. (D) CD + TOPHs-H group. (E) CD + CHO group. (F) HFD + SPPs group. (G) HFD + TOPHs-L group. (H) HFD + TOPHs-M group. (I) HFD + TOPHs-H group. (J) HFD + CHO group. Arrows indicate in (a): ( ) hepatocyte; ( ) central vein; ( ) steatosis; ( ) hydropic degeneration; ( ) vacuolization; ( ) leucocyte infiltration; ( ) vascular congestion. Arrows indicate in (b): ( ) Bowman's space; ( ) tubular lumen. | |
4. Conclusions
In this study, hydrolysates obtained from T. ovatus muscle proteins by treatment with trypsin hydrolysis were analyzed for their hypolipidemic effect on hyperlipidemic mice. The hydrolysates were mainly composed of small molecules below 3 kDa, up to 77.30%, revealed strong bile acid binding capability. Fractions of the ultra-filtration, which showed the highest bile acid binding capability, were purified by gel filtration chromatography and RP-HPLC and identified using LC-ESI-Q-TOF-MS/MS. A total of 68 peptides were identified from TOHPs-5, of which 9 peptides were hydrophobic more than 60%, which could be a significant contributor to the hypolipidemic effect of the protein hydrolysates and peptides. The fish protein hydrolysates had no toxic effect at the experimental dose, significantly improved the lipid profile and hepatorenal function, and reduced the risk of development of hepatocellular injury and atherosclerosis-associated conditions. The overall results suggest that T. ovatus muscle is an excellent dietary protein resource for the production of beneficial proteins and peptides, and they could be used to develop functional foods or therapeutic agents aimed at preventing or treatment of hyperlipidemia and atherosclerosis-related disease.
Conflicts of interest
The authors declare no conflict of interest.
Acknowledgements
The authors are indebted to Dr Yongli Gao, Shikun Dai, and engineer Yun Zhang (the Equipment Public Service Center, SCSIO, CAS) for their assistance with the high-speed refrigerated centrifuge and mass spectrometric analysis. This research was funded by the National Key R&D Program of China (No. 2018YFC0311202), the Science and Technology Program of Guangzhou, China (No. 201804010321, 201804010364 and 201707010164), the Natural Science Foundation of Guangdong Province (No. 2018A0303130144, 2018A030313903, 2018A030313088 and 2018A030313626), the Special Project for Marine Economic Development of Guangdong Province (No. [2020]036 and [2020]038), the Open Projects of Guangdong Key Laboratory of Marine Materia Medica (LMM2016-4) and the Pearl River S&T Nova Program of Guangzhou (201710010095).
References
- S. Kianbakht, B. Abasi, M. Perham and F. Hashem Dabaghian, Phytother. Res., 2011, 25, 1849–1853 CrossRef CAS PubMed.
- H. B. Khaled, Z. Ghlissi, Y. Chtourou, A. Hakim, N. Ktari, M. A. Fatma, A. Barkia, Z. Sahnoun and M. Nasri, Food Res. Int., 2012, 45, 60–68 CrossRef.
- N. Inoue, K. Nagao, K. Sakata, N. Yamano, P. E. R. Gunawardena, S.-Y. Han, T. Matsui, T. Nakamori, H. Furuta and K. Takamatsu, Lipids Health Dis., 2011, 10, 85 CrossRef CAS PubMed.
- B. Deng, T. Luo, Y. Huang, T. Shen and J. Ma, High Alt. Med. Biol., 2012, 13, 13–21 CrossRef CAS PubMed.
- A. K. Ramírez-Jiménez, R. Reynoso-Camacho, M. E. Tejero, F. León-Galván and G. Loarca-Piña, Food Res. Int., 2015, 76, 92–104 CrossRef.
- K. S. Jain, M. Kathiravan, R. S. Somani and C. J. Shishoo, Bioorg. Med. Chem., 2007, 15, 4674–4699 CrossRef CAS PubMed.
- Y. Ye, H. Xing and Y. Guo, Indian J. Exp. Biol., 2013, 51, 458–463 CAS.
- G. Kumar, A. Srivastava, S. K. Sharma and Y. K. Gupta, AYU J., 2012, 33, 197–201 CrossRef PubMed.
- B. Santharam, P. Ganesh, R. Soranam and P. L. NCJ, World J. Pharm. Pharm. Sci., 2015, 4, 1593–1607 CAS.
- S. Surya, R. Arun Kumar, B. Carla and C. Sunil, Bull. Fac. Pharm. Cairo Univ., 2017, 55, 73–77 Search PubMed.
- R. Hosomi, M. Fukao and K. Fukunaga, Trace Nutrients Res., 2010, 27, 21–27 Search PubMed.
- I. Lassoued, M. Trigui, Z. Ghlissi, R. Nasri, K. Jamoussi, M. Kessis, Z. Sahnoun, T. Rebai, A. Boualga and M. Lamrisenhadji, Food Funct., 2014, 5, 1224–1231 RSC.
- C. C. Udenigwe and K. Rouvinen-Watt, Int. J. Mol. Sci., 2015, 16, 9303–9313 CrossRef CAS PubMed.
- G. Chiesa, M. Busnelli, S. Manzini and C. Parolini, Mar. Drugs, 2016, 14, 113 CrossRef PubMed.
- M. Chalamaiah, k. B. Dinesh, R. Hemalatha and T. Jyothirmayi, Food Chem., 2012, 135, 3020–3038 CrossRef CAS PubMed.
- P. Laurent, R. Rozenn, F. Martine, V. Laurent, J. Pascal, C. D. Maryse, G. Fabienne, C. Aurélie, L. G. Yves and A. Oscarmartinez, J. Sci. Food Agric., 2010, 90, 1819–1826 Search PubMed.
- R. Hosomi, K. Fukunaga, H. Arai, S. Kanda, T. Nishiyama and M. Yoshida, J. Food Sci., 2011, 76, 116–121 CrossRef PubMed.
- L. Liu, Y. Wang, C. Peng and J. Wang, Int. J. Mol. Sci., 2013, 14, 3124–3139 CrossRef CAS PubMed.
- N. Ktari, R. Nasri, K. Mnafgui, K. Hamden, O. Belguith, T. Boudaouara, A. El Feki and M. Nasri, Process Biochem., 2014, 49, 890–897 CrossRef CAS.
- T.-Y. Wang, C.-H. Hsieh, C.-C. Hung, C.-L. Jao, M.-C. Chen and K.-C. Hsu, J. Funct. Foods, 2015, 19, 330–340 CrossRef CAS.
- R. Wang, J. Feng, Y. Su, L. Ye and J. Wang, Vet. Microbiol., 2013, 162, 957–963 CrossRef CAS PubMed.
- H. Lin, X. Chen, S. Chen, Z. Li, Z. Huang, J. Niu, K. Wu and X. Lu, Aquacult. Res., 2012, 44, 151–156 CrossRef CAS.
- M. Gao, L. Feng, T. Jiang, J. Zhu, L. Fu, D. Yuan and J. Li, Food Control, 2014, 37, 1–8 CrossRef CAS.
- Z. Ma, H. Guo, P. Zheng, L. Wang, S. Jiang, J. G. Qin and D. Zhang, Fish Physiol. Biochem., 2014, 40, 1157–1167 CAS.
- H. Guo, Z. Ma, S. Jiang, D. Zhang, N. Zhang and Y. Li, Indian J. Fish., 2014, 61, 93–95 Search PubMed.
- B. Cai, H. Chen, H. Sun, H. Sun, P. Wan, D. Chen and J. Pan, J. Med. Food, 2015, 18, 1262–1269 CrossRef CAS PubMed.
- T. S. Kahlon, M. H. Chapman and G. E. Smith, Food Chem., 2007, 103, 676–680 CrossRef CAS.
- Z. Zhu, Z. Lin, H. Jiang, Y. Jiang, M. Zhao and X. Liu, Food Funct., 2017, 8, 1680–1687 RSC.
- F. Blachier, A. H. Lancha Júnior, C. Boutry and D. Tomé, Amino Acids, 2010, 38, 15–22 CrossRef CAS PubMed.
- D. Kritchevsky, S. A. Tepper, S. K. Czarnecki and D. M. Klurfeld, Atherosclerosis, 1982, 41, 429–431 CrossRef CAS.
- A. Biswas, P. Dhar and S. Ghosh, J. Food Sci., 2010, 75, H274–H279 CrossRef CAS PubMed.
- H. Wergedahl, B. Liaset, O. A. Gudbrandsen, E. Lied, M. Espe, Z. Muna, S. Mørk and R. K. Berge, J. Nutr., 2004, 134, 1320–1327 CrossRef CAS PubMed.
- S. Louala, S. Hamza-Reguig, A. Benyahia-Mostefaoui, A. Boualga and M. Y. Lamri-Senhadji, J. Funct. Foods, 2011, 3, 321–328 CrossRef CAS.
- J. U. Kongodiamoukala, J. Nsoratindana and H. Zhang, Asian J. Biochem., 2011, 6, 439–449 CrossRef CAS.
- G. Porez, J. Prawitt, B. Gross and B. Staels, J. Lipid Res., 2012, 53, 1723–1737 CrossRef CAS.
- M. S. Ramsvik, B. Bjørndal, R. Vik, I. Bruheim, J. Skorve and R. K. Berge, Funct. Foods Health Dis., 2013, 3, 428–440 CrossRef CAS.
- C. C. Udenigwe, A. Mohan and S. Wu, J. Food Biochem., 2015, 39, 344–348 CrossRef CAS.
- C. C. Udenigwe and R. E. Aluko, J. Food Sci., 2012, 77, R11–R24 CrossRef CAS PubMed.
- T. Saeki, T. Kuroda, M. Matsumoto, R. Kanamoto and K. Iwami, J. Agric. Chem. Soc. Jpn., 2002, 66, 467–470 CAS.
- R. J. S. de Castro and H. H. Sato, Food Res. Int., 2015, 74, 185–198 CrossRef PubMed.
- G. Chen, Y. Chen, Y. Hou, Y. Huo, A. Gao, S. Li and Y. Chen, Lebensm.-Wiss. Technol., 2020, 117, 108571 CrossRef CAS.
- Y.-H. Lin, J.-S. Tsai and G.-W. Chen, J. Food Biochem., 2017, 41, e12385 CrossRef.
- F. Zhong, X. Zhang, J. Ma and C. F. Shoemaker, Food Res. Int., 2007, 40, 756–762 CrossRef CAS.
- J. C. R. Ruiz, D. A. B. Ancona and M. R. S. Campos, Nutr. Hosp., 2014, 29, 776–784 CAS.
- R. Nasri, O. Abdelhedi, I. Jemil, I. Ben Amor, A. Elfeki, J. Gargouri, A. Boualga, M. Karra-Châabouni and M. Nasri, RSC Adv., 2018, 8, 9383–9393 RSC.
- Y. Ding, L. Pu and J. Kan, J. Funct. Foods, 2017, 32, 80–89 CrossRef CAS.
- S. J. Ban, C. W. Rico, I. C. Um and M. Y. Kang, Food Chem. Toxicol., 2012, 50, 130–134 CrossRef CAS PubMed.
- D. He, P. Zhang, S. Xuan, X. Li, L. Wang and Y. Xu, Plant Foods Hum. Nutr., 2017, 72, 1–8 CrossRef PubMed.
- D. Su, R. Zhang, F. Hou, J. Chi, F. Huang, S. Yan, L. Liu, Y. Deng, Z. Wei and M. Zhang, Food Funct., 2017, 8, 808–815 RSC.
- J. E. Feig, B. Hewing, J. D. Smith, S. L. Hazen and E. A. Fisher, Circ. Res., 2014, 114, 205–213 CrossRef CAS PubMed.
- A. V. Khera, M. Cuchel, M. de la Llera-Moya, A. Rodrigues, M. F. Burke, K. Jafri, B. C. French, J. A. Wilensky, E. R. Mohler, G. H. Rothblat and D. J. Rader, N. Engl. J. Med., 2011, 364, 127–135 CrossRef CAS PubMed.
- H. Zhang, J. Wang, Y. Liu, L. Gong and B. Sun, Food Funct., 2016, 7, 2747–2753 RSC.
- A. Howard and C. C. Udenigwe, Food Funct., 2013, 4, 40–51 RSC.
- R. Hosomi, K. Fukunaga, H. Arai, T. Nishiyama and M. Yoshida, J. Agric. Food Chem., 2009, 57, 9256–9262 CrossRef CAS PubMed.
- R. Hosomi, K. Fukunaga, H. Arai, S. Kanda, T. Nishiyama and M. Yoshida, J. Food Sci., 2011, 76, H116–H121 CrossRef CAS PubMed.
- M. Nasri, Adv. Food Nutr. Res., 2016, 81, 109–159 Search PubMed.
- B. Liaset, L. Madsen, Q. Hao, G. Criales, G. Mellgren, H.-U. Marschall, P. Hallenborg, M. Espe, L. Frøyland and K. Kristiansen, Biochim. Biophys. Acta Mol. Cell Biol. Lipids, 2009, 1791, 254–262 CrossRef CAS PubMed.
- H. Wergedahl, O. A. Gudbrandsen, T. H. Røst and R. K. Berge, Nutrition, 2009, 25, 98–104 CrossRef CAS PubMed.
- M. Rizk, M. El-Sherbiny, I. H. Borai, M. K. Ezz, H. Aly, A. Matloub, A. R. Farrag and G. I. Fouad, Int. J. Pharm. Pharmaceut. Sci., 2016, 8, 43–55 CAS.
- F. Shi, J. Li, L. Yang, G. Hou and M. Ye, Food Funct., 2018, 9, 880–889 RSC.
Footnote |
† Electronic supplementary information (ESI) available: The peptide sequences identified in the TOPHs-5 fraction using LC-ESI-Q-TOF-MS/MS (Table S1). Effects of TOPHs on body weight and organ indices in mice (Fig. S1). See DOI: 10.1039/d0ra02428g |
|
This journal is © The Royal Society of Chemistry 2020 |