DOI:
10.1039/D0RA01926G
(Paper)
RSC Adv., 2020,
10, 17180-17184
La2O2CO3:Tb3+ one-dimensional nanorod with green persistent luminescence
Received
29th February 2020
, Accepted 14th April 2020
First published on 1st May 2020
Abstract
Trivalent terbium-doped oxycarbonate (La2O2CO3:1%Tb3+) one-dimensional nanorods are synthesized via a facile precipitation method. The average length of La2O2CO3:1%Tb3+ nanorods is 184.5 nm. Doping Tb3+ ions led to several visible emission peaks at 486 nm, 542 nm, and 587 nm under excitation of 258 nm wavelength light. The green afterglow at 542 nm can be detected almost 600 s after ceasing the UV-light irradiation. It can be calculated that the La2O2CO3:1%Tb3+ sample has one shallow trap depth (E = 0.848 eV) by measuring the thermoluminescence. All the results indicate that a simple precipitation method can synthesize a one-dimensional nanorod with green persistent luminescence.
Introduction
Persistent luminescent nanoparticles (PLNPs) are types of optical materials that have plenty of crystal defects as the charge-trappers. PLNPs can store charge carriers (electrons and holes) under excitation with ultraviolet (UV) radiation and then release subsequently for several seconds, minutes and even hours.1–4 PLNPs are playing an important role in multi-disciplinary fields because of their broad applications, such as in safety signage, night-vision surveillance, latent fingerprint images, decoration and biomedicine.5–9
Nowadays, only a few terbium doped bulk-phosphors such as Lu2O3:Tb3+,10 SrAl2O4:Tb3+ etc,11 have demonstrated their green afterglow. The synthesis method of these materials is a solid-state method, yet almost no successful chemical route can be traced to perfect the size, morphology and dispersion for PLNPs.10–14 However, the effective combination of nanostructure and rare earth doped persistent luminescent materials are the valid ways to expand the development of PLNPs in the multi-disciplinary fields.15–17 For example, it had been reported many PLNPs with excellent morphology and size properties synthesized via effectively chemical methods, which have shown great application potential in the field of biology.2,18–22 Therefore, it is necessary and meaningful to find a method of synthesizing a green PLNPs with controllability morphology and uniform dispersity. Furthermore, the inorganic compounds: La2O2CO3 are used to be host materials. Because rare-earth doping La2O2CO3 can be substituted for La3+ ions homogeneously and cannot change host crystal structures, as well as bringing good luminescent properties and high chemical stability.12,23
In this research, the green La2O2CO3:1%Tb3+ PLNPs are prepared via a facile precipitation method which appear to be uniformly rod-shaped (length: 50–200 nm, uniform and regular). The La2O2CO3:1%Tb3+ nanorods exhibit a visible emission at 486 nm, 542 nm, and 587 nm under 258 nm excitation. The green afterglow at 542 nm can be detected almost 600 s after ceasing the UV-light irradiation. The thermoluminescence (ThL) spectrum reveals that La2O2CO3:1%Tb3+ nanorods have a shallow trap depth at 0.848 eV.
Experiment methods
Sample preparation
Chemicals and materials. The initial rare-earth nitrates, including La(NO)3·6H2O (99.99%) and Tb(NO3)3·6H2O (99.99%) were purchased from Shanghai Aladdin Biochemical Technology Company, and NH4OH (25 wt%) was purchased from Tianjin Zhiyuan Chemical Reagent Company, and anhydrous ethanol was purchased from Sinopharm Chemical Reagent Company. All chemicals were of analytical-grade reagents and were used directly without further purification.
Preparation of La2O2CO3:1%Tb3+. The La2O2CO3:1%Tb3+ nanorods were compounded by a precipitation method.23,24 Firstly, 0.01 mol of La(NO3)3·6H2O and 0.0001 mol of Tb(NO3)3·6H2O were added to 100 mL of deionized water followed through vigorous stirring for 10 min. Secondly, NH4OH (25 wt%) was added to the mixture to reach the pH = 10. Finally, the mixture in a beaker, wrapped with a polyethylene film, was heated in a water bath at 90 °C for 2 h and heavily stirred and then cooled to room temperature. The precursor products La(OH)3:1%Tb3+ nanorods were obtained by washing the resulting precipitates with deionized water and anhydrous ethanol respectively for three times, and then dried at 80 °C for 12 h in the oven. The final La2O2CO3:1%Tb3+ nanorods were collected after annealing the precursor products at 600 °C for 3 h in the muffle furnace. The carbon atom maybe come from carbon dioxide in air atmosphere.
Characterization
The X-ray diffraction (XRD) (Cu/Kα) patterns of samples were recorded on a PANAlytical X'Pert PRO X-ray polycrystal diffractometer. Transmission electron microscopy (TEM) images and scanning electron microscopy (SEM) images were obtained on a Thermo-Talos F200S and Hitach-SU8220, respectively. Dynamic light scattering spectrum of samples was performed on a Malvern Instruments-Zetasizer Nano ZS at 25 °C. The dispersant is ethanol. Room temperature photoluminescence (PL), PL excitation (PLE) spectra, afterglow spectrum and decay curves of samples were monitored on a high-resolution spectrofluorometer (UK, Edinburgh Instruments, FLS 980) equipped with a 500 W Xenon lamp as an excitation source, with a Hamamatsu R928P visible photomultiplier (250–850 nm). Thermoluminescence (ThL) spectrum of samples was measured on a FJ-427A TL meter (Beijing, China).
Results and discussions
Phase and crystal structure
The X-ray diffraction (XRD) patterns of samples are described in Fig. 1a. The XRD patterns of the host La2O2CO3 are in good agreement with the hexagonal La2O2CO3 phase (JCPDS No. 37-0804) and no impurity peak was detected. When small amount of Tb3+ doped in the host, the peak position remains unchanged but the main peak shape of the XRD patterns becomes wider. In La2O2CO3, the La site (ionic radius: 0.127 nm, CN = 10) bonds to three category O atoms (three O sites: O1, O2, O3), thus offering the applicable decahedron coordination LaO10 to attach the rare-earth emitter Tb3+ (ionic radius: 0.110 nm, CN = 9).25 Tb3+ may replace La3+ lattice site in this architecture based on the ionic radius and LaO10 coordination configuration (Fig. 1b). It is possible that the peak of the doped sample is broadening owing to the substitution of the La3+ by the relatively size Tb3+.
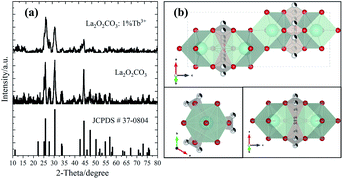 |
| Fig. 1 XRD patterns (a) and crystal structure (b) of La2O2CO3:1%Tb3+ sample. | |
Morphology and texture
The size and morphology of La2O2CO3:1%Tb3+ nanorods are investigated via scanning electron microscopy (SEM), transmission electron microscopy (TEM) and high-resolution TEM (HRTEM). As revealed in Fig. 2a, La2O2CO3:1%Tb3+ sample is a kind of one-dimension rod-like nanomaterial with uniform dispersion. Additionally, the hydrodynamic size of La2O2CO3:1%Tb3+ nanorods is measured via dynamic light scattering (DLS). It confirms that size distribution follows a normal distribution with average length 184.5 nm. The polydispersity index (PdI) value (0.248) shows that the sample is moderately polydisperse (Fig. 2b).26 These results indicate that the precipitation method can successful compound well-dispersed PLNPs.
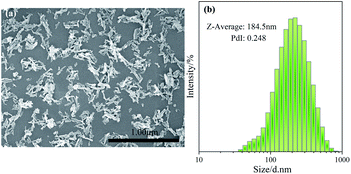 |
| Fig. 2 SEM image (a) and dynamic light scattering (DLS) spectrum (b) of La2O2CO3:1%Tb3+ sample. | |
For further revealing the information on morphology and structural features of La2O2CO3:1%Tb3+ nanorods, the TEM and HRTEM also be measured. Fig. 3a and b display the typical TEM images of La2O2CO3:1%Tb3+ nanorods. It demonstrates that the diameter of nanorods is 15–20 nm and the length are 50–200 nm, which is in keeping with DLS result. Fig. 3c displays the HRTEM image of La2O2CO3:1%Tb3+ nanorods. The lattice planes of nanorod are counted to be 2.0388 nm and 1.3299 nm, which belong to (110) and (211) crystallographic planes of La2O2CO3, respectively. It indicates that La2O2CO3:1%Tb3+ nanorod is polycrystalline while the different crystal orientations can be found distinctly. The presence of indiscernible lattice fringes in La2O2CO3 nanorod due to the formation of defects.27 The crystal diffraction spot image of a single nanorod (Fig. 3d) insinuates that nanorod is polycrystalline, which matches greatly with the HRTEM results.
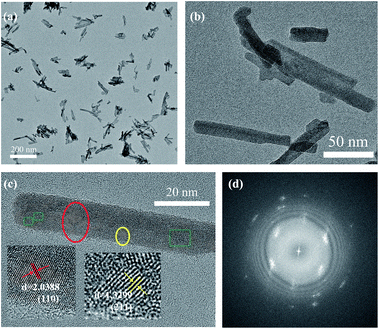 |
| Fig. 3 TEM images (a and b), HRTEM image (c) and crystal diffraction spot image (d) of La2O2CO3:1%Tb3+ sample. | |
Photoluminescent properties
The excitation and emission spectra are used to illustrate the photoluminescent property of La2O2CO3:1%Tb3+ nanorods (the doping content of 1% has been reported to be a suitable value for preparing Tb3+-doped luminescent phosphors),5,23,28,29 as shown in Fig. 4a. An evident excitation peak at 258 nm can be measured when monitoring at 542 nm emission at room temperature. It can be assigned to the spin allowed inter-configurational 4f8–4f75d1 transition of Tb3+.30–33 The emission spectrum of La2O2CO3:1%Tb3+ covers a wide wavelength range between 400 nm and 600 nm. Peaks at 486 nm, 542 nm and 587 nm are observed, which can be ascribed by the 4f–4f transitions from 5D4 to 7FJ (J = 6, 5, 4). The blue emission peaks in 400–470 nm are attributed to the 5D3 to 7FJ (J = 5, 4, 3) transitions, respectively (Fig. 4b).9,32,34–37 The bright green-light emitting belongs to the strongest transition 5D4 to 7F5 of largest probability for both forced electric dipole and magnetic dipole induced transitions.13,36
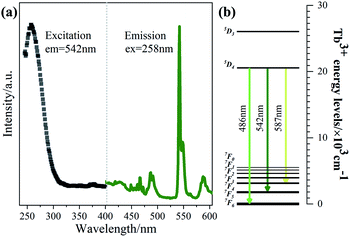 |
| Fig. 4 The excitation and emission spectrum (a) and the energy levels image (b) of the La2O2CO3:1%Tb3+ sample. | |
Persistent luminescence property
The afterglow decay curve of La2O2CO3:1%Tb3+ sample is presented in Fig. 5a. It clearly reveals that the decay process includes a fast decay part and a slow decay process. A strong afterglow at 542 nm appear at the initial stage of fast decay while a long afterglow duration almost 600 s after ceasing the UV-light irradiation support the existing slow decay. The color of the sample is yellow-color possibly due to the existence of extra tetravalent terbium ions (Tb4+).38 Particularly, no feature fluorescence of Tb4+ are found in Fig. 4a, because it may play the role of ionic impurities. It is well-known that the generation of long persistent luminescence is primarily attributed to the capture of excited electrons via creating traps in the host lattice.1 The ThL curve can provide vital information about the number, depth and density of traps,39 which are advantageous to reveal the possible afterglow mechanism. Therefore, ThL curve of La2O2CO3:1%Tb3+ sample is measured (Fig. 5b). Samples were excited at Texc by 254 nm light for 20 min, the heating rate of 2 K s−1. ThL spectrum consists a broad band locating at 424 K. The depths and densities of traps can be estimated by the following equations:40 E = Tm/500 (where E is the thermo-active energy of trap depths (in eV) denoting the energy gap between the electron trap and the conduction band, and Tm is the ThL peak temperature in kelvin). The trap depth of La2O2CO3:1%Tb3+ is 0.848 eV.
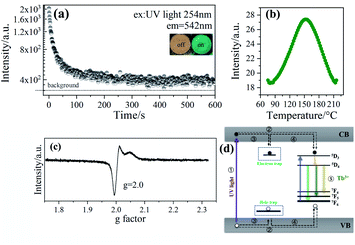 |
| Fig. 5 The persistent luminescence spectrum (the duration time is 5 s between the turn-off of the excitation light and the beginning of the measurements, and measurement apparatus) (a), thermoluminescence spectrum (the scan rate is 2 K s−1, and the duration time is 10 s between the turn-off of the excitation light and the beginning of the measurements for the TL measurements) (b), EPR spectrum (c) and the afterglow mechanism (d) of the La2O2CO3:1%Tb3+ sample. | |
Electron spin resonance (ESR) is an effective and direct characterization to analysis varied behaviours of defects.13,26 Fig. 5c exhibits the ESR test result of La2O2CO3:1%Tb3+ sample. It can be seen an ESR signal at g = 2.0, which is close to free electrons generating from oxygen vacancies
26,41–43
is a common electron-trap in oxide long afterglow phosphors.44
The terbium ions in the La2O2CO3 host lattice would substitute the La3+. Hence, when most of Tb3+ ions entered La3+ site, the neutral defect sites
will be given as eqn (1):36,44
|
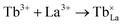 | (1) |
On the other hand, the Tb4+ impurities also have chance to substitute the La3+, the unbalanced substitution occurred as eqn (2):36,45
|
 | (2) |
The Tb ions with a positive net charge
acted as electron-traps. Meanwhile, a charge compensation is coming out owing the unequally charges possibly.46 It means a simultaneous creation of a La3+ vacancy
which acted as hole-traps.
Based on experimental results of afterglow properties, doping Tb3+ ions not only acted as an activator but also formed point defects
The Tb4+ impurities not only changed the sample's color, but also created electron-traps
and hole-traps
A possible diagrammatic drawing of La2O2CO3:1%Tb3+ afterglow mechanism is shown in Fig. 5d. To explain, the Tb3+ energy levels are putted between the conduction band (CB) and valence band (VB). The band gap of La2O2CO3 is 5.83 ev.24 During ultraviolet (UV) light irradiation (①), most of the excited electrons and holes are transferred through the CB and VB to the luminescence centers of Tb3+ respectively (②), and then causing the characteristic emissions of Tb3+ (⑤). However, some of the excited electrons and holes will be captured by electron-traps
from CB or hole-traps
from VB through non-radiative transition respectively (③). Then, the stored electrons and holes can escape from the traps by the proper thermal activation, and the released energy be transferred to Tb3+ ions via the VB and CB (④). Then the afterglow of Tb3+ (⑤) is appearing.
Conclusions
In this paper, the La2O2CO3:1%Tb3+ PLNPs have been synthesized via a facile precipitation method. The morphology and texture of La2O2CO3:1%Tb3+ sample display uniform dispersion and rod-like shape. The strongest emission peak locates at 542 nm which attributes to 5D4 to 7F5 transition. The green afterglow at 542 nm can be detected almost 600 s after ceasing the UV-light irradiation. ThL measurement reveals that La2O2CO3:1%Tb3+ sample possesses one shallow trap depth calculated to be 0.848 eV. The results show that the one-dimensional nanorods with green long afterglow can be successfully synthesized via a simple precipitation method.
Conflicts of interest
There are no conflicts to declare.
Acknowledgements
This work was financially supported by the National Natural Science Foundation of China (Grant No. 51602063), Guangdong Natural Science Foundation (Grant No. 2019A030310444), and Science and Technology Program of Guangzhou (201804010257). This work was also supported by Guangdong High-level personnel of special support program and Open Fund of the State Key Laboratory of Luminescent Materials and Devices (South China University of Technology).
References
- Y. Li, M. Gecevicius and J. Qiu, Chem. Soc. Rev., 2016, 45, 2090–2136 RSC.
- J. Wang, Q. Ma, X.-X. Hu, H. Liu, W. Zheng, X. Chen, Q. Yuan and W. Tan, ACS Nano, 2017, 11, 8010–8017 CrossRef CAS PubMed.
- Z. Pan, Y.-Y. Lu and F. Liu, Nat. Mater., 2012, 11, 58–63 CrossRef CAS PubMed.
- Z. Yan, Z. R. xia, Z. Xia, R. X. guang, H. Z. dong and Z. J. hua, Chin. J. Lumin., 2010, 31, 489–492 Search PubMed.
- M. Li, X. Yu, T. Wang, J. Qiu and X. Xu, Ceram. Int., 2015, 41, 11523–11527 CrossRef CAS.
- Y. Teng, J. Zhou, S. N. Khisro, S. Zhou and J. Qiu, Mater. Chem. Phys., 2014, 147, 772–776 CrossRef CAS.
- H. Li, R. Pang, S. Zhang, L. Lv, J. Feng, L. Jiang, D. Li, C. Li and H. Zhang, Dalton Trans., 2018, 47, 9814–9823 RSC.
- Y. Liang, F. Liu, Y. Chen, K. Sun and Z. Pan, Dalton Trans., 2016, 45, 1322–1326 RSC.
- G. Ju, Y. Hu, L. Chen, Z. Yang, T. Wang, Y. Jin and S. Zhang, Ceram. Int., 2015, 41, 14998–15004 CrossRef CAS.
- C. C. Pedroso, J. M. Carvalho, L. C. Rodrigues, J. Holsa and H. F. Brito, ACS Appl. Mater. Interfaces, 2016, 8, 19593–19604 CrossRef CAS PubMed.
- B.-g. Zhai and Y. M. Huang, J. Mater. Sci.: Mater. Electron., 2017, 52, 1813–1822 CrossRef CAS.
- M.-H. Lee and W.-S. Jung, Bull. Korean Chem. Soc., 2013, 34, 3609–3614 CrossRef CAS.
- B. Lei, B. Li, H. Zhang, L. Zhang, Y. Cong and W. Li, J. Electrochem. Soc., 2007, 154, H623–H630 CrossRef CAS.
- J. Trojan-Piegza, J. Niittykoski, J. Hölsä and E. Zych, Chem. Mater., 2008, 20, 2252–2261 CrossRef CAS.
- A. Escudero, A. I. Becerro, C. Carrillocarrion, N. O. Nunez, M. V. Zyuzin, M. Laguna, D. Gonzalezmancebo, M. Ocana and W. Parak, Nanophotonics, 2017, 6, 881–921 CAS.
- H.-X. Mai, Y.-W. Zhang, R. Si, Z.-G. Yan, L.-d. Sun, L.-P. You and C.-H. Yan, J. Am. Chem. Soc., 2006, 128, 6426–6436 CrossRef CAS PubMed.
- Y. Xia, Nat. Mater., 2008, 7, 758–760 CrossRef CAS PubMed.
- B. B. Srivastava, A. Kuang and Y. Mao, Chem. Commun., 2015, 51, 7372–7375 RSC.
- E. Teston, S. Richard, T. Maldiney, N. Lievre, G. Y. Wang, L. Motte, C. Richard and Y. Lalatonne, Chem.–Eur. J., 2015, 21, 7350–7354 CrossRef CAS PubMed.
- Z. Li, Y. Zhang, X. Wu, L. Huang, D. Li, W. Fan and G. Han, J. Am. Chem. Soc., 2015, 137, 5304–5307 CrossRef CAS PubMed.
- Z. Zhou, W. Zheng, J. Kong, Y. Liu, P. Huang, S. Zhou, Z. Chen, J. Shi and X. Chen, Nanoscale, 2017, 9, 6846–6853 RSC.
- H. Liu, X. Hu, J. Wang, M. Liu, W. Wei and Q. Yuan, Chin. Chem. Lett., 2018, 29, 1641–1644 CrossRef CAS.
- G. Li, C. Peng, C. Zhang, Z. Xu, M. Shang, D. Yang, X. Kang, W. Wang, C. Li and Z. Cheng, Inorg. Chem., 2010, 49, 10522–10535 CrossRef CAS PubMed.
- X. Dou, Y. Li, T. Vaneckova, R. Kang, Y. Hu, H. Wen, X. Gao, S. Zhang, M. Vaculovicova and G. Han, J. Lumin., 2019, 215, 116635 CrossRef CAS.
- R. D. Shannon, Acta Crystallogr., Sect. A: Cryst. Phys., Diffr., Theor. Gen. Crystallogr., 1976, 32, 751–767 CrossRef.
- S. Bhattacharjee, J. Controlled Release, 2016, 235, 337–351 CrossRef CAS PubMed.
- F. Dong, X. Xiao, G. Jiang, Y. Zhang, W. Cui and J. Ma, Phys. Chem. Chem. Phys., 2015, 17, 16058–16066 RSC.
- H. Luo, A. J. Bos and P. Dorenbos, J. Phys. Chem. C, 2017, 121, 8760–8769 CrossRef CAS PubMed.
- L. C. Rodrigues, H. F. Brito, J. Hölsä, R. Stefani, M. C. Felinto, M. Lastusaari, T. Laamanen and L. A. Nunes, J. Phys. Chem. C, 2012, 116, 11232–11240 CrossRef CAS.
- Y. Mayama, T. Masui, K. Koyabu and N. Imanaka, J. Alloys Compd., 2008, 451, 132–135 CrossRef CAS.
- T. Masui, K. Koyabu, S. Tamura and N. Imanaka, J. Mater. Sci.: Mater. Electron., 2005, 40, 4121–4123 CrossRef CAS.
- C. Liu, G. Che, Z. Xu and Q. Wang, J. Alloys Compd., 2009, 474, 250–253 CrossRef CAS.
- S. X. yuan, F. X. xuan, H. J. jie, L. Q. song, J. G. yuan, D. Yun, L. Y. shi and W. C. lei, Chin. J. Lumin., 2020, 41, 265–270 CrossRef.
- J. Cao, X. Wang, X. Li, Y. Wei, L. Chen and H. Guo, J. Lumin., 2016, 170, 207–211 CrossRef CAS.
- J. Santos, E. Silva, N. Souza, Y. Alves, D. Sampaio, C. Kucera, L. Jacobsohn, J. Ballato and R. Silva, Ceram. Int., 2019, 45, 3797–3802 CrossRef CAS.
- X. Fu, S. Zheng, J. Shi, Y. Li and H. Zhang, J. Lumin., 2017, 184, 199–204 CrossRef CAS.
- L. Z. qiu, Z. J. su, L. X. ping, S. J. shi, C. L. hong, Z. H. yang and C. B. jiu, Chin. J. Lumin., 2013, 34, 30–34 CrossRef.
- J. Kaszewski, B. S. Witkowski, Ł. Wachnicki, H. Przybylińska, B. Kozankiewicz, E. Mijowska and M. Godlewski, J. Rare Earths, 2016, 34, 774–781 CrossRef CAS.
- X. Lin, R. Zhang, X. Tian, Y. Li, B. Du, J. Nie, Z. Li, L. Chen, J. Ren, J. Qiu and Y. Hu, Adv. Opt. Mater., 2018, 6, 1701161 CrossRef.
- Y. Li, S. Zhou, Y. Li, K. Sharafudeen, Z. Ma, G. Dong, M. Peng and J. Qiu, J. Mater. Chem. C, 2014, 2, 2657–2663 RSC.
- K. A. Alim, V. A. Fonoberov, M. Shamsa and A. A. Balandin, J. Appl. Phys., 2005, 97, 124313 CrossRef.
- A. B. Djurišić, W. C. Choy, V. A. L. Roy, Y. H. Leung, C. Y. Kwong, K. W. Cheah, T. Gundu Rao, W. K. Chan, H. Fei Lui and C. Surya, Adv. Funct. Mater., 2004, 14, 856–864 CrossRef.
- S. Zhang, S.-H. Wei and A. Zunger, Phys. Rev. B: Condens. Matter Mater. Phys., 2001, 63, 075205 CrossRef.
- Z. Long, J. Zhou, J. Qiu, Q. Wang, D. Zhou, X. Xu, X. Yu, H. Wu and Z. Li, J. Rare Earths, 2018, 36, 675–679 CrossRef CAS.
- Y. Jin, Y. Hu, C. Li, X. Wang, G. Ju and Z. Mu, J. Lumin., 2013, 138, 83–88 CrossRef CAS.
- X. Zhou, G. Ju, T. Dai and Y. Hu, Opt. Mater. Express, 2019, 96, 109322 CrossRef CAS.
|
This journal is © The Royal Society of Chemistry 2020 |